DOI:
10.1039/C9RA06581D
(Paper)
RSC Adv., 2019,
9, 34854-34861
A nanoscopic icosahedral {Mo72Fe30} cluster catalyzes the aerobic synthesis of benzimidazoles†
Received
21st August 2019
, Accepted 19th October 2019
First published on 29th October 2019
Abstract
In this study, the catalytic efficiency of amorphous {Mo72Fe30} nanocapsules as a safe Keplerate polyoxometalate in organic synthesis was exploited. The easy-made solid catalyst exhibited high efficiency using a very low dosage (0.02–0.05 mol%) in the catalyzed condensation of various aromatic 1,2-diamines and aldehydes for the aerobic synthesis of benzimidazoles with very small E-factor values (0.11–0.33). The superior catalytic activity of amorphous nanoclusters compared to that of its crystalline counterpart was demonstrated. The high activity and recyclability of heterogeneous catalysts in a green reaction media under oxygen atmosphere, make this environmentally benign organic process appropriate for our applied goals.
Introduction
In recent decades, carbon–heteroatom bond formations have been the central subject of modern organic synthesis. Among them, N-containing heteroaromatic compounds are useful and important structures found in natural and synthetic compounds.1,2 Benzimidazole and its derivatives are an important and promising class of N-heterocycles due to their diverse biological activities and pharmacological properties.3 Extensive interest in benzimidazole-containing structures has led to wide studies for their synthesis. Three methods have been reported for the synthesis of these compounds including (i) condensation of o-phenylenediamines with carboxylic acids and their derivatives (imidates, nitriles, or orthoesters) which often requires high reaction temperatures and strong acidic conditions,4 (ii) transition-metal catalyzed coupling reactions to create the benzimidazole nucleus,5 and (iii) the condensation of o-phenylenediamine with aldehydes,6 which has been extensively used due to the accessibility of a large number of aldehydes although it requires a stoichiometric oxidant for dehydration.7 In addition to some significant limitations such as the use of expensive and noxious reagents, harsh conditions, low isolation yields and toxic metal oxidants, the use of homogeneous catalysts that cannot be recovered from the reaction medium is a serious drawback. Accordingly, the design of heterogeneous catalysts for the straight and easy synthesis of benzimidazoles is of extreme demand.8 In recent years, various catalysts including metal oxides and supported heteropolyacid catalysts have been developed as heterogeneous catalysts for benzimidazole derivatives synthesis.9,10
Polyoxometalates (POMs) as a subset of metal oxides, have an unmatched range of physical and chemical properties and the ability to form dynamic structures. They have been well considered as alternative oxidation catalysts in the past few decades.11 POMs with a structure of Keplerate, are giant metal-oxide-based nanocapsules in which 12 pentagonal [(Mo)–Mo5O21(H2O)6]6− units are linked together by 30 linkers such as {MoV2O4} in {Mo132}, or FeIIIaq, CrIIIaq and VOaq2+ in mixed metal derivatives. Keplerate-type polyoxometalates have attracted more and more attention of scientists in the areas of chemistry, physics, biology, and materials.12 Recently, it was established that, iron containing Keplerate, {Mo72Fe30} nanocluster, has potential for using in targeted delivery of drugs because of lack of toxicity.13 It can be isolated in two forms, (i) crystalline form of cluster resulting from replacement of the {MoV2O4} linkers in {Mo132} by aqua-FeIII polyhedra [FeIIIO5(H2O)]3+ in a long time,14 and (ii) amorphous form of cluster,15 which is prepared easily and immediately in high yield by addition of an iron salt to an acidified aqueous solution of sodium molybdate. The amorphous cluster is insoluble in water and ethanol making a golden opportunity for heterogeneous catalysis in green media. Just recently, we discovered catalase-like activity of {Mo72Fe30} nanoclusters in aqueous solution under visible-light irradiation.16 Our finding revealed the superior catalytic activity of amorphous {Mo72Fe30} than that of crystalline counterpart.16 The catalytic utilization of Keplerates in organic reaction, unlike the Keggin and Dawson POMs, has not been considered extensively.11 The oxidation of sulfides catalyzed by crystalline form of {Mo72Fe30} reported in 2009 is the first study on catalytic activity of Keplerate.17 After that, other research groups including us, tried to use different Keplerates in a variety of organic transformations.18–25 As {Mo72Fe30} as concerned, sulfides oxidation,17,23 and epoxidation of olefins,22 are few works performed in the present of crystalline form of cluster, and to the best of our knowledge, there is no report on catalytic utilization of amorphous {Mo72Fe30} as a high yield and easy-made heterogeneous catalyst in organic transformation yet. Following our ongoing research on catalytic activity of Keplerates,21–25 and in the line of our interest on development of new green methods for the synthesis of benzimidazoles,24,26,27 herein, for the first time the catalytic efficiency of amorphous {Mo72Fe30} in organic synthesis is exploited. A simple, mild, and efficient procedure for the synthesis of benzimidazole derivatives that employs only oxygen as the oxidant in the presence of amorphous {Mo72Fe30} as a safe heterogeneous catalyst in ethanol is described (Scheme 1). The nanoscopic polyoxometalate catalyst proved to be reusable while maintaining icosahedral integrity.
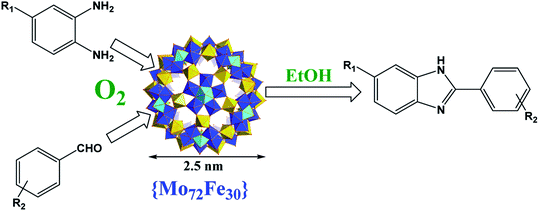 |
| Scheme 1 Synthesis of benzimidazole derivatives by {Mo72Fe30} nanoclusters and O2 as oxidant. | |
Experimental
The synthesis procedures for amorphous and crystalline {Mo72Fe30} with full characterization are given in ESI.†
General procedure for the synthesis of benzimidazoles
To a mixture of benzaldehyde (0.2 mmol) and o-phenylendiamine (0.21 mmol) in 1 mL EtOH, 2 mg {Mo72Fe30} nanocluster (0.1 μmol, 0.05 mol%) was added and the reaction mixture was stirred under 1 atm O2 (7–10 mL min−1) at 40–60 °C for the required time. Progress of the reaction was monitored by TLC. After the completion of the reaction, the mixture was cooled to room temperature and the catalyst was separated by filtration and washed with EtOH. The desired product (liquid phase) was extracted by plate chromatography, and eluted with n-hexane/ethyl acetate (10/3). Assignments of the products were made by comparison with authentic samples.24
Results and discussion
The catalytic activity of {Mo72Fe30} was tested in the synthesis of benzimidazoles (Scheme 1) by choosing a model reaction between o-phenylenediamine and 4-chlorobenzaldehyde. Trace amount of benzimidazole product was detected in a blank experiment where the model reaction was performed in the absence of {Mo72Fe30} at different conditions, suggesting the need of catalyst for successful conversion of reactants to products. To obtain the optimum reaction conditions in the presence of amorphous {Mo72Fe30}, the effect of solvent, temperature, catalyst dose and oxidant was screened (Fig. 1). As shown in Fig. 1(i and ii) ethanol is the best solvent for the reaction to proceed faster. In addition to solubility of materials, hydrogen bonding between alcohol molecules and hydroxyl/water sites on POM surface is reasonable for such an exhibition.28 The results presented in Fig. 1(iii) showed that the model reaction reached to highest performance using only 1 mg (0.05 μmol, 0.025 mol%) of the catalyst featuring a turnover number of 4000 within 25 min. Also, a minor increasing in the temperature to 35 and 40 °C reduces significantly the reaction time from 300 to 150 and 35 min respectively (iv) for condensation of the above mentioned substrates. However, less reactive substituted aldehydes and diamines required higher catalyst dose of 0.05 mol% (2 mg) and temperature of 60 °C to react efficiently at appropriate times (Table 1).
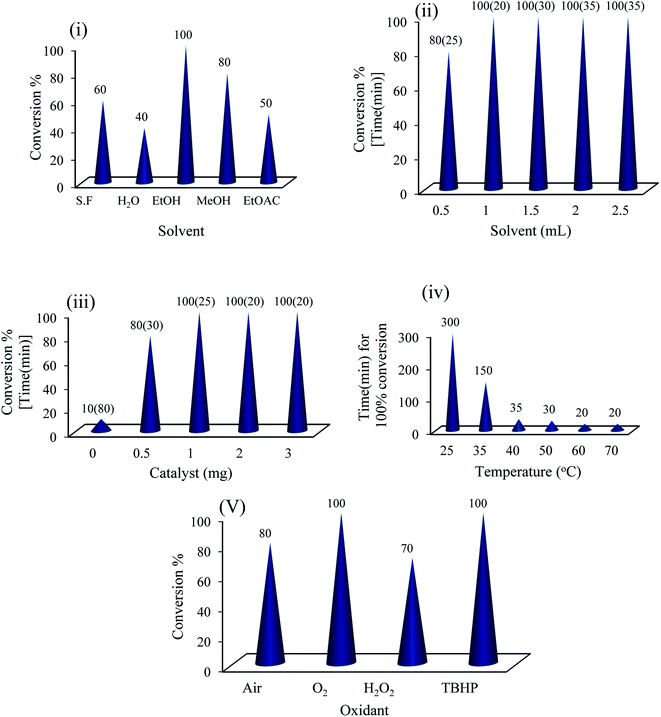 |
| Fig. 1 The screening of (i) solvent nature (ii) solvent amount (iii) catalyst dose (iv) temperature and (v) various oxidant on synthesis of benzimidazole from 4-chlorobenzaldehyde and o-phenylenediamine with molar ratio of 1/1.2 catalyzed by amorphous {Mo72Fe30}. | |
Table 1 Effect of substituent (on aromatic ring) on the reaction rate of benzimidazoles synthesis a
Finally, different common oxidants were screened and both molecular oxygen and TBHP showed to be the best (v). Nevertheless, clear environmental and economic benefits of using O2 as the terminal oxidant as well as the possible disintegration of capsule in the presence of TBHP should be taken into account. Under these optimized aerobic conditions, the catalytic system showed a wide applicability for a range of substrates for the synthesis of various benzimidazole derivatives. A variety of benzaldehydes and o-phenylenediamines carrying different substituents reacted successfully together under the catalytic influence of amorphous {Mo72Fe30} and good to excellent product yields were obtained (Table 1).
The environmental impact of the catalytic protocol which measures the amount of waste generated during a synthetic process was assessed through E-factor calculations (E-factor = total waste (kg)/total product (kg)).29 It should be noted that the mass of {Mo72Fe30} cluster has been omitted from the E-factor calculations, because of efficient recovery during the process for further usage. The calculated E-factor values for the synthesis of all the benzimidazole derivatives given in Table 1, are very small ranging between 0.11 and 0.33. These results highlight the green nature of the protocol resulting from minimum waste generation using this aerobic protocol employing ethanol as reaction medium in the presence of highly active recyclable heterogeneous {Mo72Fe30} nanocluster. However, the reaction performance was greatly decreased by introduction of electron withdrawing substituents on the aromatic ring of diamine molecule. E-factor values for nitro containing benzimidazoles formation resulting from 4-nitro-1,2-phenylenediamine increased to 1.7 and 2.28 for condensation with 4-chlorobenzaldehyde and 4-methylbenzaldeyde respectively (entries 11 and 14). As expected from the first step of mechanism (vide infra) which is nucleophilic attack of o-phenylenediamine on aromatic aldehyde, electron withdrawing nitro group decreases the nucleophilicity of –NH2 group of o-phenylenediamine retarding the formation of C
N bonds, and consequently declines the conversion and selectivity of desired benzimidazoles.
Mechanistic aspects of catalysis with {Mo72Fe30}
To clarify the catalytic role of {Mo72Fe30}, the effect of the simple salts used in the synthesis of Keplerate was investigated. Inspection of the results in Table 2 demonstrated the superiority of Keplerate in terms of activity and particularly selectivity. We also replaced the amorphous {Mo72Fe30} by crystalline counterpart to compare their catalytic performance. As shown in Table 1 (entries 2, 4 and 7) and Table 2, the use of crystalline {Mo72Fe30} lengthened the reaction with a factor of 1.5–2, while maintaining the selectivity. The larger specific surface area and particularly pore volume of amorphous cluster (Table 3, Fig. S10 and S19†) caused by its low crystallinity and formation of blackberry structures resulting from aggregation of nanoparticles (evidenced by SEM images; Fig. S8 and S17†) may be reasonable for this exhibition.30 Furthermore, greater disorders in the structure of amorphous materials providing more unsaturated sites for adsorption of molecules onto the solid surface, increase their catalytic activity.31,32 It should be noted that, metal-oxide {Mo9O9} pores resulting from structural organization of the pentagons and linkers in Keplerates provides access to the capsule's interior just for very small molecules.14 Thus, considering the above mentioned structural properties, it is reasonable to accept that the reaction taking place on the surface of the {Mo72Fe30} nanocapsule and not inside it. As depicted in Scheme 2, the MoVI sites in the {Mo72Fe30} activate the carbonyl carbon of the aromatic aldehyde for nucleophilic attack by –NH2 group of o-phenylenediamine. The resulting imine is further attacked by second –NH2 to form dihydrobenzimidazole (benzimidazoline) followed by aromatization in the presence of molecular oxygen mediated by FeIII centers to give 2-aryl-1H-benzimidazoles as product. Conducting the reaction under anoxic conditions (Ar) removed the oxidation product (benzimidazole) and just 20% by-product was observed accompanied with imine as main product. This result along with the lack of 1,2-disubstituted benzimidazole products are reasonable to suggest an oxidative mechanism for this reaction under catalytic influence of {Mo72Fe30} as presented in Scheme 2.33 Deprotonation of water molecules ligated to FeIII centers in the amorphous cluster generating 16OH− groups,30 facilitates the inner sphere interaction of the metal ion with oxygenic species,34 which accelerates the oxidative aromatization process at final step of reaction.
Table 2 The comparison of catalytic activity of amorphous {Mo72Fe30} with its simple saltsa
Catalyst |
Conversion% |
Benzimidazole selectivity% |
Time (min) |
Reaction conditions: 4-chlorobenzaldehyde: o-phenylenediamine molar ratio is 1/1.2, catalyst 0.05 mol%, EtOH (1 mL) at 60 °C. The simple salts were used according to their stoichiometry in {Mo72Fe30}. |
FeCl3·6H2O |
80 |
30 |
35 |
Na2MoO4·2H2O |
90 |
40 |
80 |
FeCl3·6H2O + Na2MoO4·2H2O |
80 |
40 |
10 |
Amorphous {Mo72Fe30} |
100 |
100 |
20 |
Crystalline {Mo72Fe30} |
100 |
100 |
40 |
Table 3 Structural properties of {Mo72Fe30} nanoclustersa
Catalyst |
SBETb (m2 g−1) |
VBJHc (cm3 g−1) |
RBJHd (nm) |
BET hysteresis curves are given in Fig. S10 and S19. Specific surface area. Pore volume. Average pore diameters. |
Amorphous {Mo72Fe30} |
15.38 |
0.2777 |
72.206 |
Crystalline {Mo72Fe30} |
5.9 |
0.0098 |
6.6 |
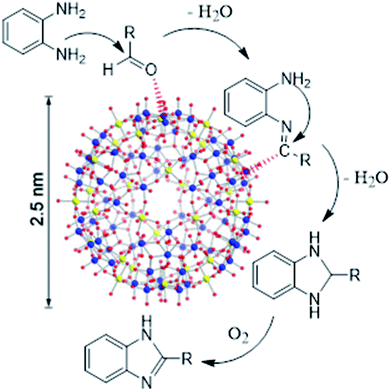 |
| Scheme 2 Proposed mechanism for catalytic action of {Mo72Fe30} nanoclusters. | |
The stability and reusability of Keplerate
To elucidate the heterogeneity of the solid catalyst, hot filtration test in association with AAS for elemental analysis of Mo and Fe was performed. The amount of elements in filtrate were trace and not detectable using this analysis method (<1 ppm) confirming the real heterogeneity of the reaction and structural stability of catalyst. More evidence for this issue was observed by comparing the FT-IR spectra of used catalyst with fresh one (Fig. 2). The characteristic fingerprint of the icosahedral structure (1000–500 cm−1) as well as the typical bands for bridging acetate ligands at 1534 and 1416 cm−1 remained intact.15 After the completion of the reaction, ethanol was added, and the catalyst was separated by centrifugation and decantation of the reaction mixture, dried and then reused. The recovered heterogeneous catalyst exhibited the same activity and selectivity and just a 4 percentage reduction in conversion was observed after five runs (90% compared to entry 2 in Table 1), demonstrating the reusability of catalyst under the reaction conditions.
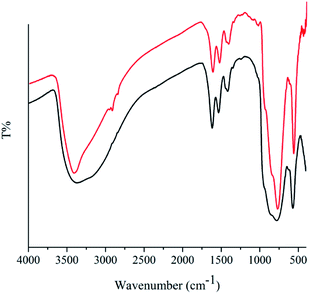 |
| Fig. 2 FT-IR spectra of fresh (black) and used catalyst (red). | |
Comparison with other catalytic systems
To show the merit of title methodology for the synthesis of 2-aryl-1H-benzimidazole derivatives, the reaction conditions and the catalytic performance of a number of catalysts in literature, are summarized in Table 4 in comparison with this work.35–46 Most of the catalysts given in Table 4, suffer seriously from toxicity and high catalyst dose. Additionally, they require toxic solvents and tedious work-up procedures or long reaction times. Although, the transition metal oxide nanoparticles demonstrated high efficiency and recoverability, in some cases such as LaCl3,6 and Pt@TiO2,44 the cost factor cannot be ignored for the industrial implementation. The present system employing {Mo72Fe30} nanocluster as an easy-made biocompatible heterogeneous catalyst in ethanol green solvent under oxygen atmosphere is found to minimize the environmental impacts evidenced by very small E-factor values and consequently qualify all requirements of an efficient catalytic system for applied goals. The high activity, environment benignity, cost effectiveness, recyclability and ease of product separation highlights the potential of this protocol in industry and make it amenable to scalability readily. When the reaction performed under a semi scale up procedure (10 mmol), 90% of the corresponding benzimidazole (Table 1, entry 2) was secured at the same time and conditions mentioned in Table 1.
Table 4 Comparison of catalytic performance of present catalytic system with literature reports for the synthesis of 2-substituted benzimidazoles
Entry |
Catalyst |
Catalyst dose/mol% |
Time/h |
Solvent |
T/°C |
Yield/% |
Ref. |
Entry 2 in Table 1. |
1 |
{Mo72Fe30} |
0.025 (0.05) |
0.5 (0.3) |
EtOH |
40 (60) |
91 (94) |
This worka |
2 |
VOSO4 |
3 |
1 |
EtOH |
r.t. |
92 |
35 |
3 |
TiCl3OTf |
10 |
0.8 |
EtOH |
r.t. |
86 |
36 |
4 |
Ce(NO3)3·6H2O |
30 |
1.5–2 |
DMF |
80 °C |
93 |
37 |
5 |
PhSiH3 |
4 equiv. |
2 |
DMF |
120 °C |
95 |
38 |
6 |
CuFe2O4 NPs |
20 |
24 |
Toluene/O2 |
110 °C |
89 |
39 |
7 |
Fe3O4–SiO2–(NH4)6Mo7O24 |
220 mg |
0.5 |
EtOH/H2O2 |
r.t. |
90 |
40 |
8 |
α-MoO3 nanobelts |
2 |
0.5 |
S·F/TBHP |
50 °C |
93 |
41 |
9 |
CuI Nps |
10 |
1 |
CH3CN/O2 |
r.t. |
96 |
42 |
10 |
TiO2–Fe2O3 |
20 mg |
3 |
H2O/O2 |
40 °C |
97 |
43 |
11 |
Pt/TiO2 |
1 |
1 |
Mesitylene |
Reflux |
78 |
44 |
12 |
H2O2/SiO2–FeCl3 |
100 mg |
0.5 |
Solvent-free |
150 °C |
25 |
45 |
13 |
LaCl3 |
10 |
2–4 |
CH3CN |
r.t. |
85–95 |
6 |
14 |
p-TsOH |
10 |
0.2–1 |
DMF |
80 °C |
Trace-85 |
46 |
Conclusion
In conclusion, Keplerate {Mo72Fe30} as a safe nanoscopic icosahedral polyoxometalate was demonstrated as a robust, efficient, and recyclable solid molecular catalyst for aerobic synthesis of benzimidazoles under mild conditions. Amorphous {Mo72Fe30} exhibited superior catalytic activity in the synthesis of benzimidazole derivatives than that of crystalline one. The larger specific surface area and particularly pore volume of amorphous cluster evidenced by BET analysis resulting from aggregation of nanoparticles is a key factor for such an exhibition. A variety of benzimidazole derivatives could be synthesized in good to excellent yields with catalyst dose as low as 0.02 to 0.05 mol% in ethanol green solvent under oxygen atmosphere. These significant conditions for a system employing a safe and biocompatible catalyst minimize the environmental impact of process opening up a promising perspective within the scope of metal cluster-catalyzed organic reactions and transformations in future.
Conflicts of interest
There are no conflicts to declare.
Acknowledgements
Support for this work by Research Council of University of Birjand is highly appreciated.
References
- Z. Xie, J. Peng and Q. Zhu, Org. Chem. Front., 2016, 3, 82–86 RSC.
- S. Lei, Y. Mai, C. Yan, J. Mao and H. Cao, Org. Lett., 2016, 18, 3582–3585 CrossRef CAS.
- M. J. Akhtar, Y. M. Shahar, V. K. Sharma, A. A. Khan, Z. Ali, M. R. Haider and A. Pathak, Curr. Med. Chem, 2019 DOI:10.2174/0929867326666190808122929.
- R. J. Perry and B. D. Wilson, J. Org. Chem., 1993, 58, 7016–7021 CrossRef CAS.
- D. Vourloumis, M. Takahashi, K. B. Simonsen, B. K. Ayida, S. Barluenga, G. C. Winters and T. Hermann, Tetrahedron Lett., 2003, 44, 2807–2811 CrossRef CAS.
- Y. Venkateswarlu, S. R. Kumar and P. Leelavathi, Org. Med. Chem. Lett., 2013, 3, 7 CrossRef.
- Y. Kim, M. R. Kumar, N. Park, Y. Heo and S. Lee, J. Org. Chem., 2011, 76, 9577–9583 CrossRef CAS.
- F. Rajabi, S. De and R. Luque, Catal. Lett., 2015, 145, 1566–1570 CrossRef CAS.
- C. Zheng, Z. Qunfeng, X. Xiaoliang and L. Xiaonian, Chin. J. Org. Chem., 2015, 35, 1189–1203 CrossRef.
- M. M. Heravi, S. Sadjadi, H. A. Oskooie, R. H. Shoar and F. F. Bamoharram, Catal. Commun., 2008, 9, 504–507 CrossRef CAS.
- S.-S. Wang and G.-Y. Yang, Chem. Rev., 2015, 115, 4893–4962 CrossRef CAS.
- A. Müller and P. Gouzerh, Chem.–Eur. J., 2014, 20, 4862–4873 CrossRef.
- A. A. Ostroushko, I. G. Danilova, I. F. Gette, S. Y. Medvedeva, M. O. Tonkushina, A. V Prokofieva and M. V Morozova, J. Biomater. Nanobiotechnol., 2011, 2, 557–560 CrossRef CAS.
- A. Müller, S. Sarkar, S. Q. N. Shah, H. Bögge, M. Schmidtmann, S. Sarkar, P. Kögerler, B. Hauptfleisch, A. X. Trautwein and V. Schünemann, Angew. Chem., Int. Ed., 1999, 38, 3238–3241 CrossRef.
- K. Kuepper, M. Neumann, A. J. M. Al-Karawi, A. Ghosh, S. Walleck, T. Glaser, P. Gouzerh and A. Müller, J. Cluster Sci., 2014, 25, 301–311 CrossRef CAS.
- R. Mokhtari, A. Rezaeifard, M. Jafarpour and A. Farrokhi, Catal. Sci. Technol., 2018, 8, 4645–4656 RSC.
- N. V Izarova, O. A. Kholdeeva, M. N. Sokolov and V. P. Fedin, Russ. Chem. Bull., 2009, 58, 134–137 CrossRef.
- A. Rezaeifard, R. Haddad, M. Jafarpour and M. Hakimi, J. Am. Chem. Soc., 2013, 135, 10036–10039 CrossRef CAS.
- A. Rezaeifard, M. Jafarpour, R. Haddad and F. Feizpour, Catal. Commun., 2017, 95, 88–91 CrossRef CAS.
- A. Rezaeifard, R. Haddad, M. Jafarpour and M. Hakimi, ACS Sustainable Chem. Eng., 2014, 2, 942–950 CrossRef CAS.
- A. Rezaeifard, A. Khoshyan, M. Jafarpour and M. Pourtahmasb, RSC Adv., 2017, 7, 15754–15761 RSC.
- F. Jalilian, B. Yadollahi, M. R. Farsani, S. Tangestaninejad, H. A. Rudbari and R. Habibi, RSC Adv., 2015, 5, 70424–70428 RSC.
- F. Jalilian, B. Yadollahi, M. R. Farsani, S. Tangestaninejad, H. A. Rudbari and R. Habibi, Catal. Commun., 2015, 66, 107–110 CrossRef CAS.
- A. Khoshyan, M. Pourtahmasb, F. Feizpour, M. Jafarpour and A. Rezaeifard, Appl. Organomet. Chem., 2019, 33, e4638 Search PubMed.
- A. Nakhaei, A. Davoodnia and H. Nakhaei, J. Chem. Rev., 2019, 1, 139–153 Search PubMed.
- F. Feizpour, M. Jafarpour and A. Rezaeifard, Catal. Lett., 2018, 148, 30–40 CrossRef CAS.
- A. Eskandari, M. Jafarpour, A. Rezaeifard and M. Salimi, New J. Chem., 2018, 42, 6449–6456 RSC.
- J. Zhou, J. Hu, M. Li, H. Li, W. Wang, Y. Liu, R. E. Winans, T. Li, T. Liu and P. Yin, Mater. Chem. Front., 2018, 2, 2070–2075 RSC.
- K. Wilson, Appl. Organomet. Chem., 2007, 21, 1002 CrossRef CAS.
- R. Mekala, S. Supriya and S. K. Das, Inorg. Chem., 2016, 55, 12504–12507 CrossRef CAS.
- B. R. Goldsmith, B. Peters, J. K. Johnson, B. C. Gates and S. L. Scott, ACS Catal., 2017, 7, 7543–7557 CrossRef CAS.
- J.-F. Deng, H. Li and W. Wang, Catal. Today, 1999, 51, 113–125 CrossRef CAS.
- N. H. Cano, J. G. Uranga, M. Nardi, A. Procopio, D. A. Wunderlin and A. N. Santiago, Beilstein J. Org. Chem., 2016, 12, 2410–2419 CrossRef CAS.
- N. I. Kuznetsova, L. I. Kuznetsova and V. A. Likholobov, J. Mol. Catal. A: Chem., 1996, 108, 135–143 CrossRef CAS.
- C. S. Digwal, U. Yadav, A. P. Sakla, P. V. S. Ramya, S. Aaghaz and A. Kamal, Tetrahedron Lett., 2016, 57, 4012–4016 CrossRef CAS.
- J. Azizian, P. Torabi and J. Noei, Tetrahedron Lett., 2016, 57, 185–188 CrossRef CAS.
- G. M. Martins, T. Puccinelli, R. A. Gariani, F. R. Xavier, C. C. Silveira and S. R. Mendes, Tetrahedron Lett., 2017, 58, 1969–1972 CrossRef CAS.
- J. Zhu, Z. Zhang, C. Miao, W. Liu and W. Sun, Tetrahedron, 2017, 73, 3458–3462 CrossRef CAS.
- D. Yang, X. Zhu, W. Wei, N. Sun, L. Yuan, M. Jiang, J. You and H. Wang, RSC Adv., 2014, 4, 17832–17839 RSC.
- G. Bai, X. Lan, X. Liu, C. Liu, L. Shi, Q. Chen and G. Chen, Green Chem., 2014, 16, 3160–3168 RSC.
- M. Jafarpour, A. Rezaeifard, M. Ghahramaninezhad and T. Tabibi, New J. Chem., 2013, 37, 2087–2095 RSC.
- P. L. Reddy, R. Arundhathi, M. Tripathi and D. S. Rawat, RSC Adv., 2016, 6, 53596–53601 RSC.
- S. Roy, B. Banerjee, N. Salam, A. Bhaumik and S. M. Islam, ChemCatChem, 2015, 7, 2689–2697 CrossRef CAS.
- C. Chaudhari, S. M. A. H. Siddiki and K. Shimizu, Tetrahedron Lett., 2015, 56, 4885–4888 CrossRef CAS.
- A. Fazlinia, M. H. Mosslemin and H. Sadoughi, J. Korean Chem. Soc., 2010, 54, 579–581 CrossRef CAS.
- H. Xiangming, M. Huiqiang and W. Yulu, ARKIVOC, 2007, 13, 150–154 Search PubMed.
Footnote |
† Electronic supplementary information (ESI) available: FT-IR, DRS, Raman, XRD, TGA, EDS, VSM, FESEM, TEM and BET of as-prepared clusters. See DOI: 10.1039/c9ra06581d |
|
This journal is © The Royal Society of Chemistry 2019 |
Click here to see how this site uses Cookies. View our privacy policy here.