DOI:
10.1039/C9RA06038C
(Paper)
RSC Adv., 2019,
9, 29873-29887
CoFe2O4/Cu(OH)2 magnetic nanocomposite: an efficient and reusable heterogeneous catalyst for one-pot synthesis of β-hydroxy-1,4-disubstituted-1,2,3-triazoles from epoxides†
Received
3rd August 2019
, Accepted 13th September 2019
First published on 20th September 2019
Abstract
A magnetically separable CoFe2O4/Cu(OH)2 nanocomposite was prepared and characterized by various techniques such as FESEM, EDS, TEM, XRD, VSM and FT-IR. This novel composite was used as a heterogeneous catalyst for the regioselective synthesis of β-hydroxy-1,4-disubstituted-1,2,3-triazoles from sodium azide, terminal alkynes and structurally different epoxides in water at 60 °C. The formation of the product proceeds in one pot through a mechanism that involves an in situ generated organic azide intermediate, followed by rapid ring closure with the alkyne component. The simple procedure, short reaction times, perfect regioselectivity, high product yields, and use of a benign solvent and nontoxic catalyst are among the considerable advantages of this protocol. Furthermore, the catalyst was easily separated using an external magnet and reused several times without any significant loss of catalytic activity or magnetic properties.
1. Introduction
1,2,3-Triazoles are significant heterocyclic intermediates for the manufacture of important compounds such as pharmaceuticals, agrochemicals, dyes and corrosion retarding agents.1–4 They also show vital biological activities such as anticancer,5 anti HIV,6 antiviral, antiallergic,7 antifungal,8 antibacterial,9 antitubercular,10 anti-inflammatory,11 antimalarial,12 and antioxidant activities.13
The Huisgen 1,3-dipolar cycloaddition of alkynes and organic azides has been developed as the principal method for synthesis of 1,2,3-triazoles.14–22 Recently, multicomponent one-pot synthesis of β-hydroxy-1,2,3-triazoles has been carried out through in situ click reaction of azides, alkynes and epoxides in the presence of heterogeneous copper catalysts such as carbon supported copper nanoparticles,23,24 porphyrinatocopper nanoparticles,25 CuSO4·5H2O/sodium ascorbate,26 copper(I)-zeolite,27 Cu[N2,N6-bis(2-hydroxyphenyl)pyridine-2,6-dicarboxamidate]/ascorbic acid,28 copper ferrite nanoparticles,29 copper(I)@phosphorated SiO2,30 Cu(OAc)2·H2O,31 (Cu/Cu2O) nanoparticles,32 Cu(II)-azide complexes,33 Cu(II)-DA@nano AlPO4,34 1,4-dihydroxyanthraquinone-copper(II) nanoparticles immobilized on silica gel,35 4′-phenyl-2,2′:6′,2′′-terpyridine copper(II) complex immobilized on activated multiwalled carbon nanotubes,36 CuI37 and NiFe2O4-glutamate-Cu.38 Ramachary–Bressy–Wang cycloaddition of enolate intermediates and organic azides is the other basic method for one-pot preparation of 1,4-disubstituted-1,2,3-triazoles.39–43
The reduction of work-up procedures and purification steps, high purity of desired products, short reaction times, high to excellent yields are among the significant benefits of multicomponent one-pot reactions.44,45 Furthermore, the reaction involving ring opening of epoxides with organic azides in water solvent occurs with perfect regioselectivity and takes advantage of green and benign conditions.
In recent years, magnetic nanoparticles (MNPs) due to their facile preparation, easy separation using an external simple magnet leading to avoid traditional filtration processes, recoverability and reusability,46,47 high catalytic activity,48 thermal and chemical stability49,50 have been widely utilized as green and efficient nanocatalysts in the synthesis of organic compounds. Nevertheless, the nano-ferrites often suffer from high tendency of self-aggregation and low quantity of functional groups on the surface.51,52 The coating of nano-ferrites with a functionalized shell, not only reduces the aggregation of the nanoparticles in the solution, but also improves the efficiency of the catalyst by increasing the amount of functional groups on the surface.53 Recently, these coated nanostructures have received special attention as air-stable materials and easy separable catalysts in organic transformations, drug targeting, and magnetic cell separation.54–57
In connection with our recent works on nano-ferrites,58–63 herein we wish to report an efficient one-pot three-component protocol for the synthesis of β-hydroxy-1,2,3-triazoles from sodium azide, terminal alkynes, and various epoxides in the presence of CoFe2O4/Cu(OH)2 magnetic nanoparticles as a novel and green heterogeneous catalyst in water (Scheme 1).
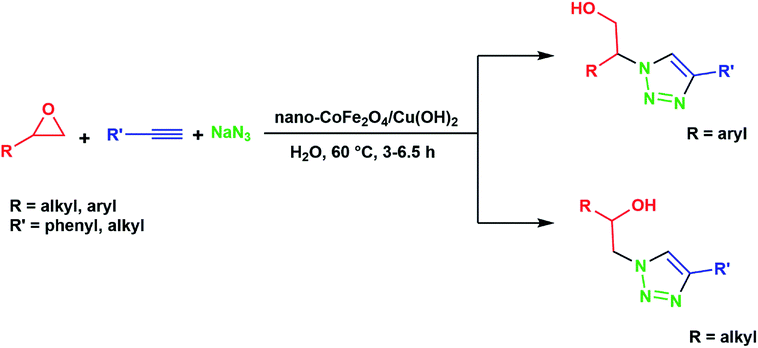 |
| Scheme 1 One-pot synthesis of triazoles from epoxides catalyzed by CoFe2O4/Cu(OH)2 nanoparticles. | |
2. Experimental
2.1. Instruments and materials
All materials were purchased from the Merck and Aldrich Chemical Companies with the best quality and they were used without further purification. The synthesized nanocatalyst was characterized by XRD on a Bruker D8-Advanced diffractometer with graphite-monochromatized Cu Kα radiation (λ = 1.54056 A°) at room temperature. Magnetic property of synthesized nanocatalyst was measured using a VSM (Meghnatis Daghigh Kavir Co., Kashan Kavir, Iran) at room temperature. TEM image was recorded using an EM10C-100 kV series microscope from the Zeiss Company, Germany. FESEM images were determined using FESEM-TESCAN. The energy dispersive X-ray spectrometer (EDS) analysis was taken on a MIRA3 FE-SEM microscope (TESCAN, Czech Republic) equipped with an EDS detector (Oxford Instruments, UK). IR and 1H/13C NMR spectra were recorded on Thermo Nicolet Nexus 670 FT-IR and 400 MHz Bruker Avance spectrometers, respectively. The products were characterized by their spectra data and comparison with the reported data in literature. All yields refer to isolated pure products.
2.2. Preparation of CoFe2O4 nanoparticles
CoFe2O4 nanoparticles were prepared by a solid-state procedure according to our previous research.58 Briefly, in a mortar, CoCl2, Fe(NO3)3·9H2O, NaOH, and NaCl were mixed with the desired molar ratio (1
:
2
:
8
:
2) and ground together for 55 min. The reaction started quickly along with the release of heat. The mixture color changed from yellow to chocolate brown after 4 min. Next, in order to remove the additional salts, the produced mixture was washed with distilled water for several times. The product was dried at 80 °C for 2 h and it was then calcined at 900 °C for 2 h to obtain the final cobalt nanoferrite as a dark brown powder.
2.3. Synthesis of CoFe2O4/Cu(OH)2 nanocomposite
In a round-bottomed flask equipped with a magnetic stirrer, a solution of CuCl2·2H2O (0.8 g, 4.7 mmol) in distilled water (30 mL) was prepared and then CoFe2O4 (1.01 g, 4.31 mmol) was added. The mixture was stirred vigorously for 10 min at room temperature and followed by dropping of NaOH solution (6 mL, 1 M) in order to basify the mixture up to pH ∼ 13. The stirring of alkali mixture was continued at room temperature for 24 h. The black nanoparticles of CoFe2O4/Cu(OH)2 were separated using a magnet, washed with distilled water and then dried under air atmosphere within two days.
2.4. One-pot synthesis of β-hydroxytriazoles from epoxides catalyzed by CoFe2O4/Cu(OH)2 in water: a general procedure
In a round-bottomed flask equipped with a magnetic stirrer and condenser, a solution of the epoxide (1 mmol), alkyne (1 mmol) and sodium azide (0.078 g, 1.2 mmol) in H2O (5 mL) was prepared. CoFe2O4/Cu(OH)2 nanocomposite (0.032 g, 0.1 mmol) was then added to the solution and the resulting mixture was stirred magnetically for 3–6.5 h at 60 °C. The progress of the reaction was monitored by TLC using CCl4
:
Et2O (10
:
2) as an eluent. After completion of the reaction, the magnetic nanocatalyst was separated using an external magnet and collected for the next run. The reaction mixture was extracted with EtOAc (3 × 10 mL) and then dried over anhydrous Na2SO4. After evaporating the organic solvent, the crude β-hydroxytriazoles were obtained. Removal of the solvent under vacuum, followed by recrystallization with EtOH/H2O (3
:
1) or purification on silica gel using CCl4
:
Et2O (10
:
2) as the eluent afforded the pure β-hydroxytriazoles derivatives in 75–95% yield (Table 2). The collected CoFe2O4/Cu(OH)2 nanoparticles were washed with distilled water and dried for the next cycle. All products are known compounds and were characterized by comparison of their spectra (FT-IR, 1H NMR and 13C NMR) with those of valid samples;23,25,26,29,30,38,45 these data are given in the ESI.†
3. Results and discussion
3.1. Synthesis and characterization of CoFe2O4/Cu(OH)2 magnetic nanocatalyst
The coating of CoFe2O4 nanoparticles was carried out with Cu(OH)2 in order to reduce the self-aggregation and also increase the amount of hydroxyl functional groups on the surface of nano-ferrite which makes the catalyst more active than the bare cobalt ferrite. The use of CoFe2O4 nanoparticles as catalyst support not only donated sufficient magnetic property for the easy separation of nanocatalyst, but also enhanced the efficiency of the catalyst by increasing the recoverability and reusability of the novel nanocomposite.
The magnetic nanoparticles of CoFe2O4/Cu(OH)2 was synthesized in a two-step process. CoFe2O4 was prepared using solid-state reaction of CoCl2, Fe(NO3)3·9H2O, NaCl and NaOH in an agate mortar (Scheme 2). After calcination of crude powder at 900 °C, the CoFe2O4 nanoparticles were obtained with high crystallinity, phase purity and increased saturation magnetization (Ms). The calcination process at high temperatures improves the efficiency of CoFe2O4, and also leads to decompose the excess salts used in the preparation of these nanoparticles.64 Afterwards, the collected pure CoFe2O4 nanoparticles were added to an aqueous solution of CuCl2 and followed by dropping of the NaOH solution under intense stirring. Eventually, the black precipitate was accumulated through magnetic separation, washed with deionized water, and dried at room temperature (Scheme 3). The prepared CoFe2O4/Cu(OH)2 nanocatalyst was characterized by vibration sample magnetometer (VSM), FT-IR, X-ray diffraction (XRD), transmission electron microscopy (TEM), field emission scanning electron microscope (FESEM), and energy dispersive X-ray spectrometer (EDS) techniques.
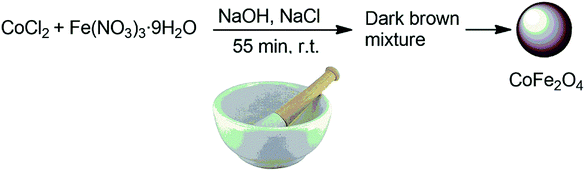 |
| Scheme 2 Synthesis of CoFe2O4 nanoparticles. | |
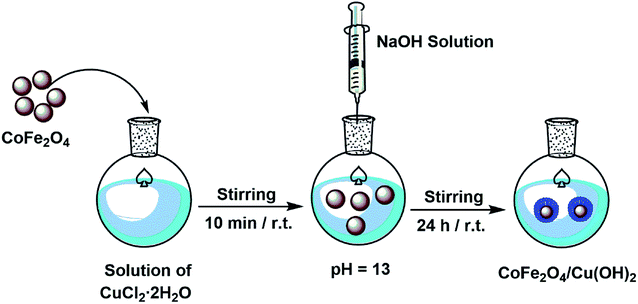 |
| Scheme 3 Synthesis of CoFe2O4/Cu(OH)2 nanocomposite. | |
3.1.1. Vibration sample magnetometer (VSM). The hysteresis loops, saturation magnetization (Ms) and switching field (Hc) of the prepared magnetic nanoparticles were measured at room temperature using vibrating sample magnetometer. The hysteresis loops of CoFe2O4 and CoFe2O4/Cu(OH)2 nanoparticles are shown in Fig. 1. The wide cycles and the hysteresis loops show the behavior of hard ferromagnetic particles with high coercivity. CoFe2O4/Cu(OH)2 nanoparticles show lower magnetization saturation (18 emu g−1) than the uncoated cobalt ferrite nanoparticles (128 emu g−1). This is due to the effect of Cu(OH)2 shell coating where each particle was separated from its neighbors by the coated layer leading to decrease the magnetostatic coupling between the particles.
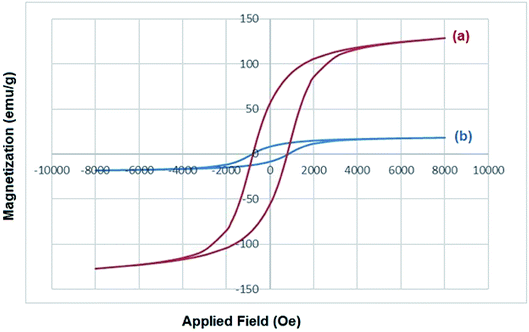 |
| Fig. 1 Magnetization curves of (a) CoFe2O4 (b) CoFe2O4/Cu(OH)2 nanoparticles. | |
3.1.2. Fourier transform infra-red (FT-IR) spectrum. The FT-IR spectrum of synthesized CoFe2O4, Cu(OH)2 and CoFe2O4/Cu(OH)2 nanoparticles are shown in Fig. 2. A strong absorption band at 596 cm−1 is corresponded to the stretching vibration of metal–oxygen (M–O) indicating the formation of spinel ferrite structure. The absorption peaks at 1608 cm−1 and 3390 cm−1 are related to bending and stretching vibrations of O–H group in surface absorbed H2O in the sample, respectively.65 A sharp and intense absorption band at 3568 cm−1 is related to the O–H stretching vibrations in crystal structure of Cu(OH)2 and the peak at 941 cm−1 indicates Cu–O–H bending vibrations.66
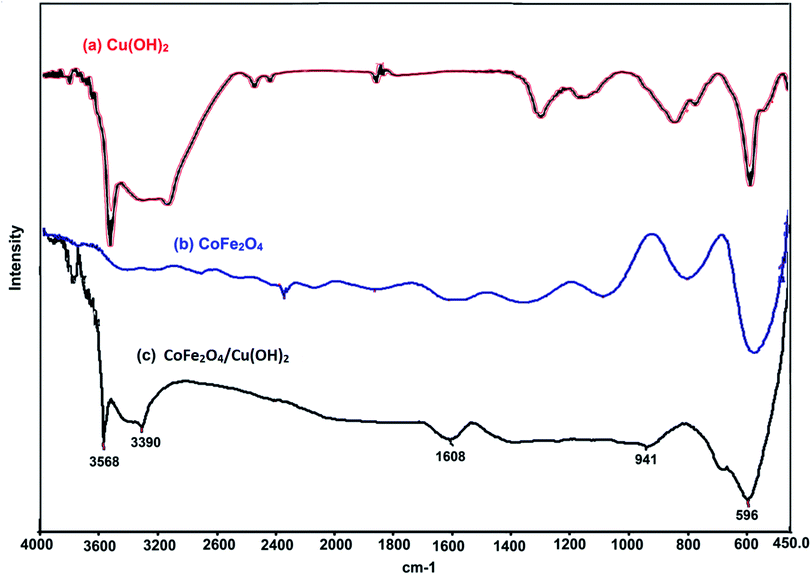 |
| Fig. 2 FT-IR (KBr) spectrum of (a) Cu(OH)2, (b) CoFe2O4 and (c) CoFe2O4/Cu(OH)2. | |
3.1.3. X-ray diffraction (XRD). Fig. 3 displays the X-ray diffraction (XRD) patterns of CoFe2O4, Cu(OH)2 and CoFe2O4/Cu(OH)2 nanoparticles. In the XRD pattern of CoFe2O4/Cu(OH)2, all the peaks of CoFe2O4 and Cu(OH)2 are detectable. The lines (111), (220), (311), (222), (400), (422), (511), (440), (620), (533) and (622) related to 2θ = 18.36°, 30.18°, 35.54°, 37.18°, 43.17°, 53.55°, 57.09°, 62.68°, 71.13°, 74.13° and 75.20° respectively, are assigned to the diffraction of CoFe2O4 crystals and indicate that the synthesized CoFe2O4 nanoparticles are pure and high crystalline. These peaks are consistent with the standard data (JCPDS card no. 22-1086).67 The peaks at 2θ = 16.81°, 23.89°, 34.09°, 38.24°, and 39.77° correspond to orthorhombic Cu(OH)2 which are in good agreement with JCPDS no. (13-420).68 The peak at 53.52° for copper hydroxide overlaps with the 53.55° peak of CoFe2O4. The average crystallite size of CoFe2O4/Cu(OH)2 nanoparticles is calculated using the Scherrer's formula (40 nm).
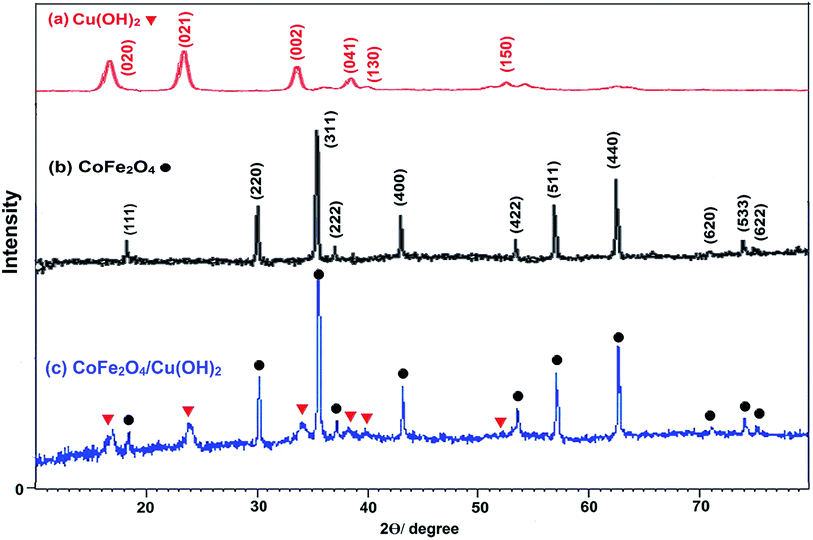 |
| Fig. 3 The X-ray diffraction patterns of (a) nano-Cu(OH)2, (b) nano-CoFe2O4 and (c) CoFe2O4/Cu(OH)2 nanocomposite. | |
3.1.4. TEM, FESEM and EDS of CoFe2O4/Cu(OH)2 nanocomposite. The morphology and size distribution of the synthesized nanocatalyst have been investigated by TEM and FESEM techniques. Fig. 4 shows the TEM images of the CoFe2O4/Cu(OH)2 nanocomposite. The TEM images with different magnifications clearly reveal the wire shape of the Cu(OH)2 nanoparticles, and also show that many nanowires come together to form a bundle-like morphology. The CoFe2O4 nanoparticles are spherical and have been uniformly surrounded by Cu(OH)2 nanowires, indicating the successful coating of CoFe2O4 surface with Cu(OH)2. The images also demonstrated that the agglomeration of CoFe2O4 nanoparticles has not occurred. The cobalt ferrite particles are clearly distinguishable as black spherical segments with diameter 40 nm. The gray particles of Cu(OH)2 are also obviously detectable in TEM images.
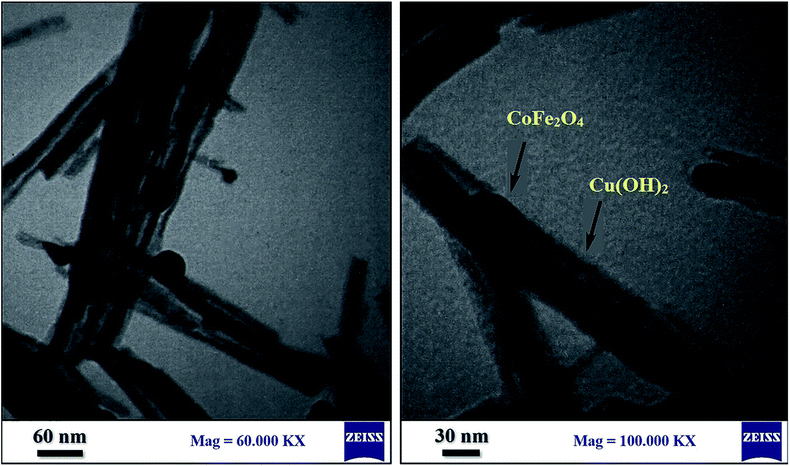 |
| Fig. 4 TEM images of CoFe2O4/Cu(OH)2. | |
The FESEM images confirm the nanowire structure of the catalyst containing the spherical cobalt ferrite nanoparticles, and show that the diameter of wires lies in the range of 28 to 40 nm. Each nanobelt with an average diameter of 32 nm is composed of tiny nanostructured grains that were created during the reaction in solution but has a uniform shape (Fig. 5). The obtained results are in good agreement with TEM and XRD data.
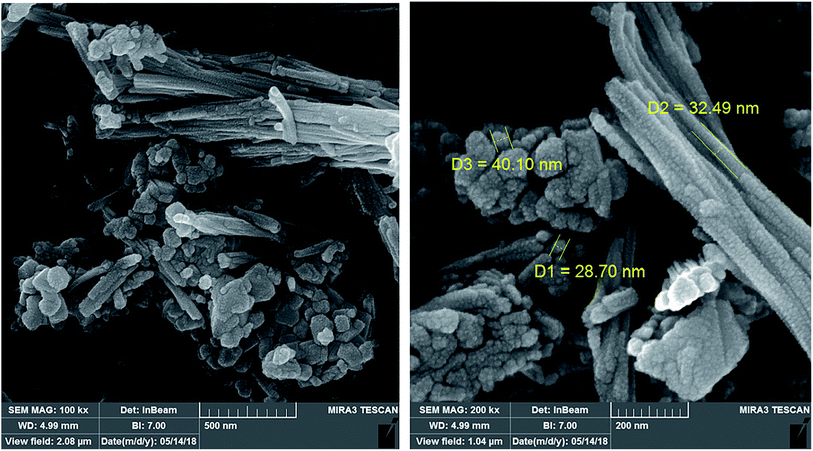 |
| Fig. 5 FESEM images of CoFe2O4/Cu(OH)2. | |
The chemical composition of CoFe2O4/Cu(OH)2 was approved with EDS analysis. In this analysis, Cu, Co, Fe, and O signals are detectable (Fig. 6).
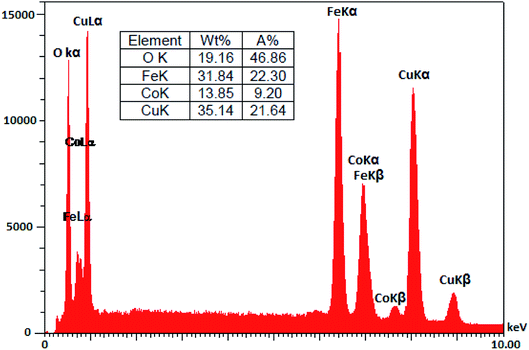 |
| Fig. 6 EDS of CoFe2O4/Cu(OH)2. | |
3.1.5. Structure of CoFe2O4/Cu(OH)2 nanocomposite. The possible structure of CoFe2O4/Cu(OH)2 nanocomposite was proposed based on the characterization results (Scheme 4). In the presented catalytic system, copper plays the key role in the [3 + 2] cycloaddition between alkyne and azide components, and the use of nano-CoFe2O4 as a magnetic support facilitates the separation, accumulation and recycling of nanoparticles.
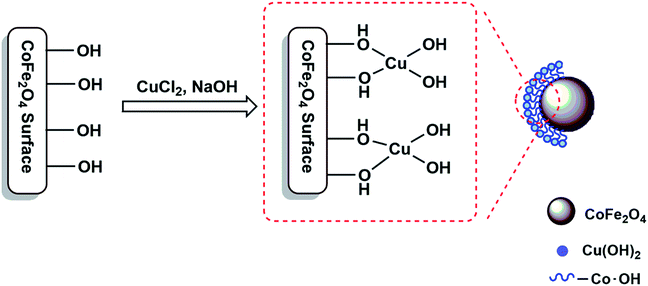 |
| Scheme 4 Structure of CoFe2O4/Cu(OH)2 as a surface modified nanocatalyst. | |
3.2. Catalytic activity of CoFe2O4/Cu(OH)2 for the synthesis of 1,4-disubstituted β-hydroxy-1,2,3-triazoles
The optimization of reaction conditions was conducted with styrene oxide, phenylacetylene and sodium azide as a model reaction. The quantity of catalyst, temperature, time and solvents were investigated as the main optimization factors (Table 1). The desired result was obtained using styrene oxide (1 mmol), phenylacetylene (1 mmol) and sodium azide (1.2 mmol) in the presence of CoFe2O4/Cu(OH)2 (0.032 g, 0.10 mmol, 10 mol%) in water at 60 °C (entry 5). As can be seen, the use of catalytic amount of nanocatalyst was essential to perform the reaction and in the absence of catalyst, no cyclization product was generated after 10 h (entry 1). The model reaction was tested using the various amounts of CoFe2O4/Cu(OH)2 (0.01, 0.02, 0.025, 0.032 and 0.045 g), and the best result was achieved using (0.032 g) of catalyst (entries 2–6). The enhancement of catalyst amount from 0.01 to 0.032 g, not only increased the reaction speed, but also led to raise the product yield significantly. Applying the more amounts of nanocatalyst did not ameliorate the product yield (entry 6).
Table 1 Nano-CoFe2O4/Cu(OH)2-catalysed reaction of styrene oxide with phenylacetylene and sodium azide under different conditionsa
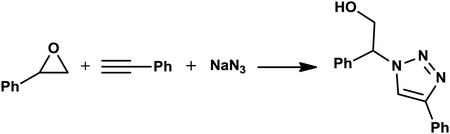
|
Entry |
CoFe2O4/Cu(OH)2 (g) |
Solvent |
Time (h) |
Temperature (°C) |
Yieldb (%) |
All reactions were carried out with styrene oxide (1 mmol), phenylacetylene (1 mmol) and sodium azide (1.2 mmol). Isolated yields. Catalysed by CoFe2O4. Catalysed by Cu(OH)2. |
1 |
— |
H2O |
10 |
60 |
0 |
2 |
0.01 |
H2O |
8 |
60 |
25 |
3 |
0.02 |
H2O |
8 |
60 |
55 |
4 |
0.025 |
H2O |
6 |
60 |
85 |
5 |
0.032 |
H2O |
3.3 |
60 |
94 |
6 |
0.04 |
H2O |
3 |
60 |
94 |
7 |
0.032 |
EtOH |
4 |
78 |
50 |
8 |
0.032 |
CH3CN |
4 |
82 |
35 |
9 |
0.032 |
EtOAc |
4 |
77 |
60 |
10 |
0.032 |
MeOH |
4 |
65 |
50 |
11 |
0.032 |
DMF |
4 |
100 |
45 |
12 |
0.032 |
THF |
24 |
60 |
0 |
13 |
0.032 |
n-Hexane |
24 |
68 |
0 |
14 |
0.032 |
H2O |
8 |
25 |
50 |
15 |
0.032 |
H2O |
5 |
40 |
65 |
16c |
0.032 |
H2O |
5 |
100 |
Trace |
17d |
0.032 |
H2O |
3.5 |
60 |
90 |
The effect of solvents was also studied by performing the cyclization reaction in various solvents. It was observed that the reaction was carried out in polar solvents, such as water, ethanol, acetonitrile, ethyl acetate, methanol and dimethylformamide whereas no reaction took place in nonpolar solvents (entries 7–13). The reaction was accomplished efficiently in H2O and in comparison with water, the product yields were lower in all other solvents; hence, this solvent was selected as a green and eco-friendly option for synthesis of triazoles.
In order to determine the effect of temperature, the reaction was repeated at different temperatures (25 °C, 40 °C and 60 °C). At room temperature (25 °C), the reaction was carried out in 8 h affording the moderate yield of product (entry 14). But increasing the temperature encouragingly raised both the product yield and reaction speed, and the corresponding triazole was prepared in 65% yield after 5 h at 40 °C (entry 15). With further increase in temperature to 60 °C the yield increased to 94% after 3.3 h (entry 5).
Performing the mentioned reaction under the optimized conditions but in the presence of CoFe2O4 (without the copper hydroxide) produced a trace of the corresponding triazole after 5 h (entry 16). Cobalt ferrite without Cu(OH)2 was not capable of conducting the reaction. The reaction was also investigated using bare Cu(OH)2 nanoparticles under the same conditions (entry 17). The reaction was carried out within 3.5 h giving 90% yield of product. The presence of copper hydroxide significantly enhanced the catalytic capability. These results approved the essential catalytic role of copper hydroxide particles. It also showed that the interactions between the Cu(OH)2 and CoFe2O4 nanoparticles may increase the catalytic activity of CoFe2O4/Cu(OH)2 composite. Cobalt ferrite plays the role of a magnetic support that enhances the effectiveness of the catalyst by increasing the contact surface and easy separability.
In order to exhibit the diversity of the CoFe2O4/Cu(OH)2 catalyst, the optimized conditions were applied to epoxides containing either electron-donating or -withdrawing substituents, and cyclic epoxides as shown in Table 2. In addition, the reaction of aliphatic terminal alkynes with styrene oxide was also considered under mentioned conditions (entries 18–21). All reactions were carried out successfully within 3–6.5 h to give 1,2,3-triazoles in 75–95% yields.
Table 2 One-pot synthesis of β-hydroxy-1,2,3-triazoles from epoxides catalyzed by nano-CoFe2O4/Cu(OH)2a
Entry |
Epoxide (a) |
Alkyne |
Triazole (b) |
Time (h) |
Yieldb (%) |
All reactions were carried out with 1 mmol of epoxide in the presence of alkyne (1 mmol), sodium azide (1.2 mmol) and nano-CoFe2O4/Cu(OH)2 (0.032 g, 0.1 mmol, 10 mol%) in water at 60 °C. Yields refer to isolated pure products. |
1 |
 |
 |
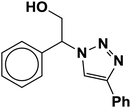 |
3.3 |
94 |
2 |
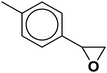 |
 |
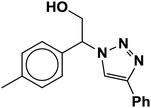 |
3 |
95 |
3 |
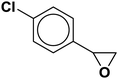 |
 |
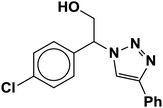 |
3.3 |
90 |
4 |
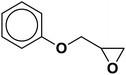 |
 |
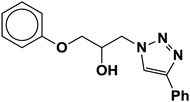 |
4.3 |
88 |
5 |
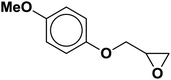 |
 |
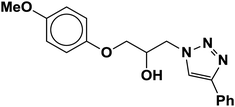 |
4.5 |
85 |
6 |
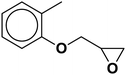 |
 |
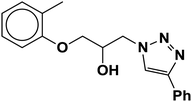 |
4.5 |
87 |
7 |
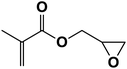 |
 |
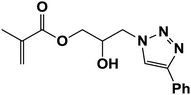 |
5.5 |
75 |
8 |
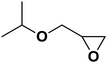 |
 |
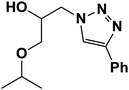 |
4.3 |
80 |
9 |
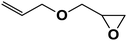 |
 |
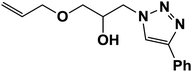 |
4.2 |
85 |
10 |
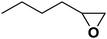 |
 |
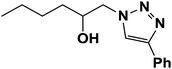 |
6.3 |
84 |
11 |
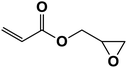 |
 |
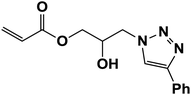 |
5 |
82 |
12 |
 |
 |
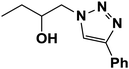 |
6 |
85 |
13 |
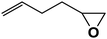 |
 |
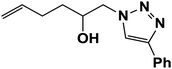 |
6 |
87 |
14 |
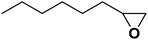 |
 |
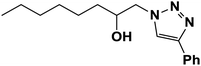 |
6.5 |
88 |
15 |
 |
 |
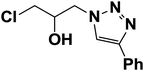 |
5 |
80 |
16 |
 |
 |
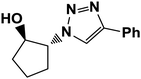 |
5 |
90 |
17 |
 |
 |
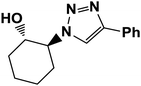 |
6 |
92 |
18 |
 |
 |
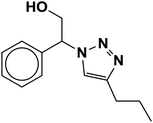 |
5.5 |
85 |
19 |
 |
 |
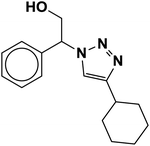 |
4 |
90 |
20 |
 |
 |
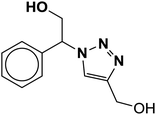 |
5 |
88 |
21 |
 |
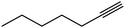 |
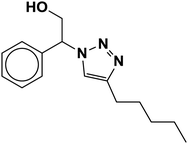 |
6.5 |
84 |
3.3. Recycling of CoFe2O4/Cu(OH)2 nanoparticles
The recyclability of nano-CoFe2O4/Cu(OH)2 was investigated in the synthesis of 3-chloro-1-(4-phenyl-1H-1,2,3-triazol-1-yl)propan-2-ol as halogen containing sample (Table 2, entry 15) and 2-hydroxy-3-(4-phenyl-1H-1,2,3-triazol-1-yl)propyl methacrylate with the lowest yield in the first run (Table 2, entry 7). After completion of the reaction, the reaction mixture was cooled to room temperature and the nanoparticles were easily collected by an external magnet, washed several times with distilled water and ethanol and dried at 70 °C for 1 h. The recovered catalyst was reused 6 times without any significant loss of activity, under the same conditions (Fig. 7). The structure of the recovered CoFe2O4/Cu(OH)2 nanocatalyst was confirmed using VSM, XRD, FESEM and TEM analyses after five runs (Fig. 8). Comparison of the results obtained from the recycled catalyst and the freshly prepared nanoparticles revealed that the magnetic properties, original structure and morphology of the catalyst remained unchanged after several reuses.
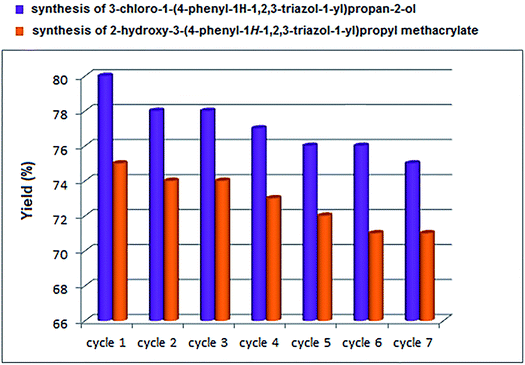 |
| Fig. 7 Recycling of nano-CoFe2O4/Cu(OH)2 in the synthesis of 3-chloro-1-(4-phenyl-1H-1,2,3-triazol-1-yl)propan-2-ol and 2-hydroxy-3-(4-phenyl-1H-1,2,3-triazol-1-yl)propyl methacrylate. | |
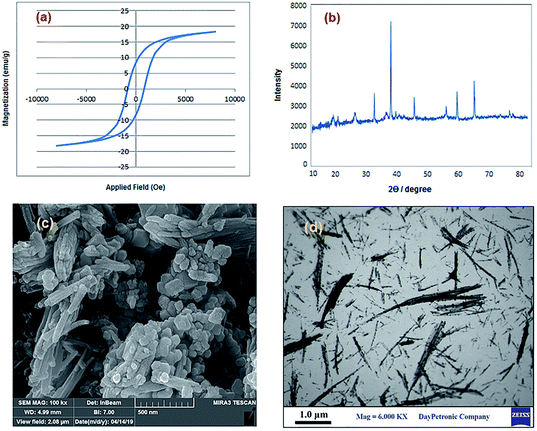 |
| Fig. 8 (a) Magnetization curve, (b) XRD pattern, (c) FESEM and (d) TEM images of CoFe2O4/Cu(OH)2 after five runs in the synthesis of 3-chloro-1-(4-phenyl-1H-1,2,3-triazol-1-yl)propan-2-ol. | |
The hot filtration test was used for confirming the heterogeneity of as-prepared catalyst. Accordingly, the filtration of the catalyst was carried out after 30 min at 80 °C and the filtrate was allowed to react for additional 2 hours, but the reaction due to the absence of copper did not take place, and no cycloaddition reaction was occurred.
3.4. Comparison of CoFe2O4/Cu(OH)2 catalytic activity with other catalysts
The comparison of the catalytic activity of the prepared CoFe2O4/Cu(OH)2 nanocomposite with various catalysts in the reaction of styrene oxide, phenylacetylene and sodium azide is summarized in Table 3. As can be seen, our proposed procedure is more efficient than the other methods in terms of product yield, and it is also comparable to the most of them from viewpoints of reaction time and temperature. The study of reported literature shows that magnetic nanocatalysts have been rarely used for one-pot synthesis of 1,2,3-triazoles from epoxides (entries 2 and 3), and the overwhelming majority of the reported cases have been carried out in the presence of non-magnetic catalysts (entries 4–11). On the other hand, in some cases, the use of acid or base alongside the catalyst is essential for the reaction progress (entries 10 and 11). Therefore, our method, due to the use of ferromagnetic nanocatalyst, takes advantage of both heterogeneity benefits and magnetic properties. In addition, performing of reaction in a green solvent under neutral conditions is really important privilege. Easy preparation and utilization of surface modified nanoparticles, simple separation just by an external magnet, convenient recovering, reusability after several cycles without loss of catalytic activity or magnetic property and subsequently facilitating the achievement to the goals of green chemistry are among the remarkable advantages of CoFe2O4/Cu(OH)2 catalytic system, which highlight its importance in comparison with the most reported catalysts. Furthermore, the one-pot reaction due to the reduction of work-up procedures and purification steps required compared to a more stepwise approach was used in order to conduct synthesis in a greener fashion.
Table 3 Comparison of CoFe2O4/Cu(OH)2 catalytic activity with various catalysts reported for the one-pot synthesis of 2-phenyl-2-(4-phenyl-1H-1,2,3-triazol-1-yl)ethanol from styrene oxide, phenylacetylene and sodium azidea

|
Entry |
Catalyst system |
NaN3 (mmol) |
Conditions |
Time (h) |
Yield (%) |
Ref. |
All reactions were carried out with styrene oxide (1 mmol) and phenylacetylene (1 mmol). bhppda = N2,N6-bis(2-hydroxyphenyl)pyridine-2,6-dicarboxamidato dianion. |
1 |
CoFe2O4/Cu(OH)2 (10 mol%) |
1.2 |
H2O/60 °C |
3.3 |
94 |
This work |
2 |
Nano CuFe2O4 (10 mol%) |
1.1 |
H2O/60 °C |
6 |
87 |
29 |
3 |
Nano Fe2O3 (10 mol%) |
1.2 |
H2O/100 °C |
5 |
81 |
45 |
4 |
Cu nanoparticles/C (0.5 mol%) |
1.1 |
H2O/70 °C |
8 |
93 |
23 |
5 |
Cu(I)@phosphorated SiO2 (0.64 mol%) |
1.1 |
H2O/60 °C |
1 |
93 |
30 |
6 |
Copper(I)–zeolite (8 mol%) |
1.2 |
H2O/r.t. |
20 |
77 |
27 |
7 |
CuI (5 mol%) |
1.1 |
PEG-400/r.t. |
16 |
83 |
37 |
8 |
CuCl2 (10 mol%) |
1 |
H2O/r.t. |
5 |
35 |
31 |
9 |
Cu(NO3)2·3H2O (10 mol%) |
1 |
H2O/r.t. |
5 |
36.8 |
31 |
10 |
[Cu(bhppda)H2O]b (5 mol%) |
1.2 |
Ascorbic acid/H2O/r.t. |
2 |
90 |
28 |
11 |
CuSO4·5H2O (10 mol%) |
1.2 |
Sodium ascorbate/H2O/r.t. |
4 |
92 |
26 |
3.5. The proposed mechanism for synthesis of 1,4-disubstituted β-hydroxy-1,2,3-triazoles catalyzed by CoFe2O4/Cu(OH)2 nanocomposite
The proposed mechanism for the formation of β-hydroxy-1,4-disubstituted-1,2,3-triazoles includes two pathways (A and B) in which CoFe2O4/Cu(OH)2 catalyzes both ring opening and 1,3-dipolar cycloaddition reactions.28 The pathway A demonstrates the formation of metal azide which is followed by epoxide activation via CoFe2O4/Cu(OH)2 catalyst. Ring opening of epoxide takes place through azide delivery from the catalyst and leads to the formation of 2-azido-2-arylethanol. The regioselective cleavage of oxiranes carrying alkyl and allyl substituents by azide ion is strongly favored from less hindered carbon of the epoxide ring via SN2 type of mechanism (β-cleavage); however, aryl-substituted epoxides prefer to be opened from the more sterically hindered position via SN1 type of mechanism (α-cleavage).
The pathway B displays the insertion of copper catalyst to the C–H bond of terminal alkyne in order to activate the phenylacetylene and produce the intermediate (I), which facilitates the [3 + 2] cycloaddition between carbon–carbon triple bond of the 2-azido-2-arylethanol and in situ generated intermediate (II), to give the Cu–C-triazole (IV). The alkyne consumption and also the generation and the disappearance of the 2-azido-2-alkylethanol intermediate, were monitored by gas chromatography (GC) analysis and thin layer chromatography (TLC) runs of the reaction mixture. Finally, protonolysis of the Cu–C bond of intermediate (IV) by aqueous media affords the corresponding β-hydroxy-1,2,3-triazole (V) (Scheme 5).
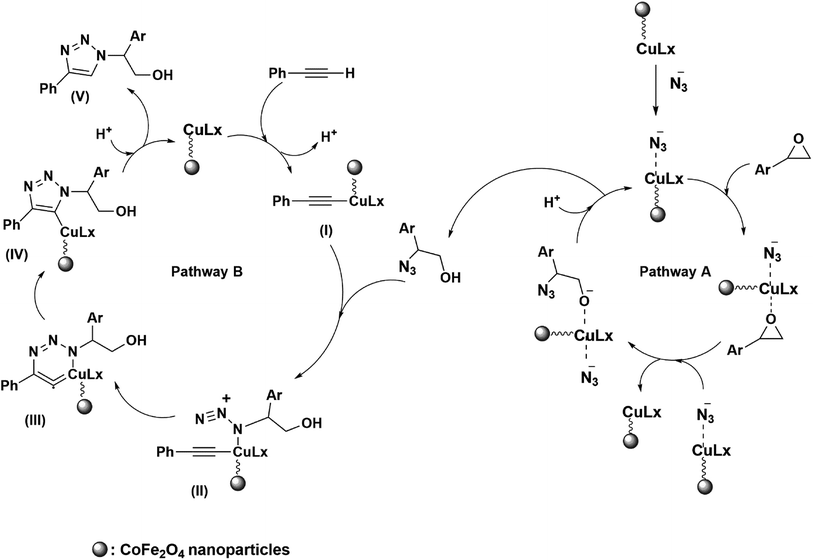 |
| Scheme 5 Reaction mechanism proposed for the one-pot synthesis of 1,4-disubstituted β-hydroxy-1,2,3-triazole catalyzed by CoFe2O4/Cu(OH)2 bifunctional catalyst. | |
4. Conclusions
In summary, the magnetic nanocatalyst of CoFe2O4/Cu(OH)2 has been successfully prepared through a simple method and it was then characterized using various techniques such as FESEM, EDS, TEM, XRD, VSM and FT-IR. This novel composite has been demonstrated to be an efficient catalyst for one-pot synthesis of a wide variety of 1,4-disubstituted-1,2,3-triazoles via three component reactions of sodium azide, terminal alkyne, and different epoxides in water. The simple procedure, short reaction times, perfect regioselectivity, high product yields, the use of benign solvent, easy separation of catalyst using an external magnet and efficient recycling are remarkable aspects of this proposed protocol that make it to be an important addition to the existing methodologies for synthesis of 1,4-disubstituted-1,2,3-triazoles.
Conflicts of interest
There are no conflicts to declare.
Acknowledgements
The financial support of this work by the Research Council of Payame Noor University is gratefully acknowledged.
References
- M. Whitting, J. Muldoon, Y. C. Lin, S. M. Silverman, W. Lindstrom, A. J. Olson, H. C. Kolb, M. G. Finn, K. B. Sharpless, J. H. Elder and V. V. Fokin, Angew. Chem., Int. Ed., 2006, 45, 1435 CrossRef PubMed.
- B. S. Holla, M. Mahalinga, M. S. Karthikeyan, B. Poojary, P. M. Akberali and N. S. Kumari, Eur. J. Med. Chem., 2005, 40, 1173 CrossRef CAS PubMed.
- V. Pande and M. J. Ramos, Bioorg. Med. Chem. Lett., 2005, 15, 5129 CrossRef CAS PubMed.
- W. Q. Fan and A. R. Katritzky, in Comprehensive Heterocyclic Chemistry II, ed. A. R. Katritzky, C. W. Rees and E. F. Scriven, Elsevier Science, Oxford, 1996, vol. 4, p. 1 Search PubMed.
- A. Kamal, S. Prabhakar, M. J. Ramaiah, P. V. Reddy, Ch. R. Reddy, A. Mallareddy, N. Shankaraiah, T. L. N. Reddy, S. N. C. V. L. Pushpavalli and M. Pal-Bhadra, Eur. J. Med. Chem., 2011, 46, 3820 CrossRef CAS PubMed.
- F. C. Silva, M. C. B. V. de Souza, I. I. P. Frugulhetti, H. C. Castro, S. L. d. O. Souza, T. M. L. de Souza, D. Q. Rodrigues, A. M. T. Souza, P. A. Abreu, F. Passamani, C. R. Rodrigues and V. F. Ferreira, Eur. J. Med. Chem., 2009, 44, 373 CrossRef PubMed.
- S. Palhagen, R. Canger, O. Henriksen, J. A. van Parys, M.-E. Riviere and M. A. Karolchyk, Epilepsy Res., 2001, 43, 115 CrossRef CAS PubMed.
- J. N. Sangshetti, R. R. Nagawade and D. B. Shinde, Bioorg. Med. Chem. Lett., 2009, 19, 3564 CrossRef CAS PubMed.
- G. Ravi, A. R. Nath, A. Nagaraj, S. Damodhar and G. N. Rao, Der Pharma Chem., 2014, 6, 223 Search PubMed.
- C. Menendez, A. Chollet, F. Rodriguez, C. Inard, M. R. Pasca, C. Lherbet and M. Baltas, Eur. J. Med. Chem., 2012, 52, 275 CrossRef CAS PubMed.
- T. W. Kim, Y. Yong, S. Y. Shin, H. Jung, K. H. Park, Y. H. Lee, Y. Lim and K. Y. Jung, Bioorg. Chem., 2015, 59, 1 CrossRef CAS PubMed.
- R. Raj, P. Singh, P. Singh, J. Gut, P. J. Rosenthal and V. Kumar, Eur. J. Med. Chem., 2013, 62, 590 CrossRef CAS PubMed.
- M. F. Mady, G. E. A. Awad and K. B. Jørgensen, Eur. J. Med. Chem., 2014, 84, 433 CrossRef CAS PubMed.
- A. Domling and I. Ugi, Angew. Chem., Int. Ed., 2000, 39, 3168 CrossRef CAS PubMed.
- R. Huisgen, Pure Appl. Chem., 1989, 61, 613 CAS.
- R. Huisgen, G. Szeimies and L. Moebius, Chem. Ber., 1965, 98, 4014 CrossRef CAS.
- C. S. Radatz, L. d. A. Soares, E. F. Vieira, D. Alves, D. Russowsky and P. H. Schneider, New J. Chem., 2014, 38, 1410 RSC.
- M. I. Mangione, R. A. Spanevello and M. B. Anzardi, RSC Adv., 2017, 7, 47681 RSC.
- H. X. Siyang, H. L. Liu, X. Y. Wu and P. N. Liu, RSC Adv., 2015, 5, 4693 RSC.
- W. D. Castro-Godoy, A. A. Heredia, L. C. Schmidt and J. E. Arguello, RSC Adv., 2017, 7, 33967 RSC.
- N. V. Sokolova and V. G. Nenajdenko, RSC Adv., 2013, 3, 16212 RSC.
- W. Yang, T. Miao, P. Li and L. Wang, RSC Adv., 2015, 5, 95833 RSC.
- F. Alonso, Y. Moglie, G. Radivoy and M. Yus, J. Org. Chem., 2011, 76, 8394 CrossRef CAS PubMed.
- F. Alonso, Y. Moglie, G. Radivoy and M. Yus, Adv. Synth. Catal., 2010, 352, 3208 CrossRef CAS.
- H. Sharghi, M. H. Beyzavi, A. Safavi, M. M. Doroodmand and R. Khalifeh, Adv. Synth. Catal., 2009, 351, 2391 CrossRef CAS.
- J. S. Yadav, B. V. S. Reddy, G. M. Reddy and D. N. Chary, Tetrahedron Lett., 2007, 48, 8773 CrossRef CAS.
- T. Boningari, A. Olmos, B. M. Reddy, J. Sommer and P. Pale, Eur. J. Org. Chem., 2010, 2010, 6338 CrossRef.
- H. Sharghi, M. Hosseini-Sarvari, F. Moeini, R. Khalifeh and A. S. Beni, Helv. Chim. Acta, 2010, 93, 435 CrossRef CAS.
- B. S. P. Anil Kumar, K. Harsha Vardhan Reddy, G. Satish, R. Uday Kumar and Y. V. D. Nageswar, RSC Adv., 2014, 4, 60652 RSC.
- H. Naeimi and V. Nejadshafiee, New J. Chem., 2014, 38, 5429 RSC.
- K. Rajender Reddy, C. Uma Maheswari, K. Rajgopal and M. Lakshmi Kantam, Synth. Commun., 2008, 38, 2158 CrossRef.
- H. Esmaeili-Shahri, H. Eshghi, J. Lari and S. A. Rounaghi, Appl. Organomet. Chem., 2018, 32, e3947 CrossRef.
- N. Noshiranzadeh, M. Emami, R. Bikas and A. Kozakiewicz, New J. Chem., 2017, 41, 2658 RSC.
- H. Sharghi and I. Ghaderi, Org. Chem. Res., 2017, 3, 162 Search PubMed.
- H. Sharghi, A. Khoshnood, M. M. Doroodmand and R. Khalifeh, J. Iran. Chem. Soc., 2012, 9, 231 CrossRef CAS.
- H. Sharghi, S. Ebrahimpourmoghaddam, M. M. Doroodmand and A. Purkhosrow, Asian J. Org. Chem., 2012, 1, 377 CrossRef CAS.
- G. Kumaraswamy, K. Ankamma and A. Pitchaiah, J. Org. Chem., 2007, 72, 9822 CrossRef CAS PubMed.
- J. Lu, E. Q. Ma, Y. H. Liu, Y. M. Li, L. P. Mo and Z. H. Zhang, RSC Adv., 2015, 5, 59167 RSC.
- D. B. Ramachary, A. B. Shashank and S. Karthik, Angew. Chem., Int. Ed., 2014, 53, 1 CrossRef PubMed.
- D. B. Ramachary, K. Ramakumar and V. V. Narayana, Chem.–Eur. J., 2008, 14, 9143 CrossRef CAS PubMed.
- Z. E. Blastik, B. Klepetářová and P. Beier, ChemistrySelect, 2018, 3, 7045 CrossRef CAS.
- R. Sangwan, Javed, A. Dubey and P. K. Mandal, ChemistrySelect, 2017, 2, 4733 CrossRef CAS.
- H. Yuan, L. Zhang, Z. Liu, Y. Liu, J. Wang and W. Li, Org. Biomol. Chem., 2017, 15, 4286 RSC.
- F. Nasiri, A. Zolali and S. Asadbegi, J. Heterocycl. Chem., 2016, 53, 989 CrossRef CAS.
- M. Murty, M. R. Katiki and D. Kommula, Can. Chem. Trans., 2016, 4, 47 CAS.
- C. W. Lim and I. S. Lee, Nano Today, 2010, 5, 412 CrossRef CAS.
- M. Gawande, A. Rathi, P. Branco and R. Varma, Appl. Sci., 2013, 3, 656 CrossRef CAS.
- Z. Zhang, Y. Liu, G. Yao, G. Zu and Y. Hao, Int. J. Appl. Ceram. Technol., 2013, 10, 142 CrossRef CAS.
- A. H. Lu, E. L. Salabas and F. Schuth, Angew. Chem., Int. Ed., 2007, 46, 1222 CrossRef CAS PubMed.
- Y. Zhu, L. P. Stubbs, F. Ho, R. Liu, C. P. Ship, J. A. Maguire and N. S. Hosmane, ChemCatChem, 2010, 2, 365 CrossRef CAS.
- T. Wang, L. Zhang, H. Wang, W. Yang, Y. Fu, W. Zhou, W. Yu, K. Xiang, Z. Su, S. Dai and L. Chai, ACS Appl. Mater. Interfaces, 2013, 5, 12449 CrossRef CAS PubMed.
- J. Saiz, E. Bringas and I. Ortiz, J. Chem. Technol. Biotechnol., 2014, 89, 909 CrossRef CAS.
- B. Chen, Z. Zhu, J. Ma, M. Yang, J. Hong, X. Hu, Y. Qiu and J. Chen, J. Colloid Interface Sci., 2014, 434, 9 CrossRef CAS PubMed.
- V. Polshettiwar, R. Luque, A. Fihri, H. Zhu, M. Bouhrara and J.-M. Basset, Chem. Rev., 2011, 111, 3036 CrossRef CAS PubMed.
- Z. Shokri, B. Zeynizadeh and S. A. Hosseini, J. Colloid Interface Sci., 2017, 485, 99 CrossRef CAS PubMed.
- K. Bakhmutsky, N. L. Wieder, M. Cargnello, B. Galloway, P. Fornasiero and R. J. Gorte, ChemSusChem, 2012, 5, 140 CrossRef CAS PubMed.
- R. G. Chaudhuri and S. Paria, Chem. Rev., 2012, 112, 2373 CrossRef PubMed.
- S. Hassanzadeh, R. Eisavi and M. Abbasian, Appl. Organomet. Chem., 2018, 32, e4520 CrossRef.
- R. Eisavi and S. Alifam, Phosphorus, Sulfur Silicon Relat. Elem., 2017, 193, 211 CrossRef.
- R. Eisavi, S. Ghadernejad, B. Zeynizadeh and F. M. Aminzadeh, J. Sulfur Chem., 2016, 37, 537 CrossRef CAS.
- R. Eisavi, F. Ahmadi, B. Ebadzade and S. Ghadernejad, J. Sulfur Chem., 2017, 38, 614 CrossRef CAS.
- S. Hassanzadeh, R. Eisavi and M. Abbasian, J. Sulfur Chem., 2019, 40, 240 CrossRef CAS.
- M. Mahmoudzadeh, E. Mehdipour and R. Eisavi, J. Coord. Chem., 2019, 72, 841 CrossRef CAS.
- Z. P. Sun, L. Liu, D. Z. Jia and W. Pan, Sens. Actuators, B, 2007, 125, 144 CrossRef CAS.
- S. Sathiya, K. Parasuraman, M. Anbarasu and K. Balamurugan, Nano Vision, 2015, 5, 133 Search PubMed.
- J. M. Aguirre, A. Gutiérrezb and O. iraldo, J. Braz. Chem. Soc., 2011, 22, 546 CrossRef CAS.
- H. Iida, K. Takayannagi, T. Nakamishi and T. Osaka, J. Colloid Interface Sci., 2007, 314, 274 CrossRef CAS PubMed.
- L. Wang, K. Zhang, Z. Hu, W. Duan, F. Cheng and J. Chen, Nano Res., 2014, 7, 199 CrossRef CAS.
Footnote |
† Electronic supplementary information (ESI) available: Spectral data for β-hydroxy-1,2,3-triazoles. See DOI: 10.1039/c9ra06038c |
|
This journal is © The Royal Society of Chemistry 2019 |
Click here to see how this site uses Cookies. View our privacy policy here.