DOI:
10.1039/C9RA06672A
(Paper)
RSC Adv., 2019,
9, 36707-36716
Toxicity testing of MWCNTs to aquatic organisms
Received
24th August 2019
, Accepted 31st October 2019
First published on 11th November 2019
Abstract
The increase in global production of carbon nanotubes (CNTs), as well as their use in polymer nanocomposites has raised concerns as to their possible effects on the marine environment that could ultimately affect human populations. Specifically, CNTs have already been tested in antifouling formulations for the prevention of biofouling, mainly to protect ships' hulls, as well as in composite materials that come in contact with seawater. At this point, it seems crucial to assess the possible effects of CNTs on aquatic organisms and assess their toxicity. Thus, in this study, three different model organisms were selected for toxicity testing: Daphnia magna water flea, Artemia salina nauplii and Danio rerio zebrafish. The CNTs that were tested have been produced in house via the chemical vapour deposition method and were fully characterised in order to understand the effect of their properties on the aquatic organisms. In this study pristine multiwalled carbon nanotubes (MWCNTs) as well as functionalised with carboxyl groups were used. Dispersion issues were evident in all tests, both for the pristine and functionalised carbon nanotubes, thus their toxicity could not be determined in relation to their concentration. To overcome this issue, optical observation of the organisms took place. MWCNT black aggregates were clearly observed in the intestine of A. salina. Following an additional 24 h in seawater the intestine appeared clean and restored to its normal appearance. This observation leads to the conclusion that MWCNTs did not prove to be fatal to D. magna and A. salina despite their presence in the digestive track of both non-target organisms. These results show that MWCNTs do not affect the non-target organisms in the short term, thus their use in antifouling coatings and composite materials for maritime applications can be further investigated.
Introduction
Carbon nanotubes (CNTs) are sheets of graphene that have been rolled to form a cylinder in nanoscale.1 They consist of elemental carbon with sp2 hybridization that forms hexagons. Single-walled carbon nanotubes (SWCNTs) are composed of a single cylindrical sheet of graphene, while multi-walled carbon nanotubes (MWCNTs) consist of several graphene sheets (Fig. 1).2 The diameter of CNTs typically ranges from 0.4 to 3 nm for SWCNTs and 1.4 to 100 nm for MWCNTs.3 Due to their excellent physico-chemical, electrical, and mechanical properties, they are applied in numerous innovative fields,4,5 including polymer nanocomposites,6 coatings7 and microelectronics.8
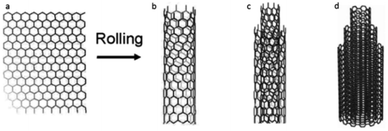 |
| Fig. 1 Carbon nanotubes (CNT) composed of (a) sheet of graphene (b) single (SWCNT), (c) (DWCNT) double and (d) multiple layers (MWCNT), following Jackson, et al.17 | |
At present, the potential hazardous impact of CNTs on the aquatic environment is not fully understood.9,10 A major difficulty in testing CNTs is due to their hydrophobic nature and tendency to spontaneously agglomerate in an aqueous medium.11–13 Previous studies have revealed a correlation between the toxicity of MWCNTs, their size, tendency to bind with metal catalysts, and surface functionalization.12 SWCNTs are considered as more toxic than MWCNTs, probably due to their higher surface area along with their lower tendency to agglomerate and higher hydrodynamic ability.14–16
The transfer of CNTs through marine food webs, and therefore its bioaccumulation at higher trophic levels, is of major concern, especially when the organism in question is used as a food source for human.17 For instance, hepatic oxidative stress and catalyst metals accumulation in goldfish exposed to different types of CNTs under various pH levels was studied by Wang et al. (2015).18
The fish embryos toxicity test (FET)19 is considered an imperative standard for testing chemicals prior to their introduction into the market.20 This test monitors embryonic development under different concentrations of the antifouling compounds.21 It also avoids the need to test adult fish, which involves ethical considerations. In the framework of conventional ecotoxicity testing, fish constitute an indispensable component of the integrated toxicity testing strategy in the aquatic environment.19
Nauplii of the brine shrimp, Artemia salina, have been considered a simple and suitable model system for acute toxicity tests.22 The nauplii feature a higher sensitivity to toxic agents compared to the adult Artemia. They should preferably be aged to Instar II, which is known as more sensitive to toxicity compared to Instar I.22
The water flea, Daphnia magna, is also an important model organism in ecotoxicological studies.23 D. magna filters large amounts of water and suspended particles, thus making it an adequate model system for toxicity tests.24 Specifically, Wang et al. (2016) have tested the toxicity of metals (cadmium25 and arsenic26) synergistically with the presence of CNTs to D. magna. It was demonstrated that CNTs could enhance the toxicity of the metals to D. magna, especially due to the presence of catalyst impurities, high adsorption capacity of oxygen-functionalised MWCNTs and accumulation that occurred. According to the above and since D. magna is a freshwater inhabitant27 which can mitigate the dispersion of CNTs, it has been used in the current study to test CNTs toxicity.
Experimental
Materials and methods
Carbon nanotubes synthesis. CNTs were synthesized in the Research of Advanced, Composite, Nanomaterials and Nanotechnology, of the National Technical University of Athens, in a Thermal, Catalytic Chemical Vapor Deposition (TC–CVD) reactor. Through the TC–CVD process, CNTs with tailored properties can be achieved, since their geometric characteristics, as well as their structure and purity, can be defined. In order to prepare structures of high purity, the floating catalyst approach was utilized, as first reported in Trompeta, et al.28 Adjustments were made on the reactor, which consists of a cylindrical furnace that can be heated up to 1200 °C and forms the main part of the TC–CVD system. As reaction chamber, a clean metallic tube of stainless steel was used (3.4 mm inner diameter and 1.10 cm long), due to the high pressure that is reached in the system. The reaction took place in the thermal zone of the furnace which is 50 cm, under inert conditions that were maintained with a continuous flow of nitrogen (380 mL min−1). Specifically, a Si wafer substrate of 〈100〉 orientation was placed in the middle of the thermal zone, after its sterilization with ethanol and ultrasonication, on which the deposition of the CNTs is achieved. As carbon source, camphor was used (96% purity in weight, Sigma Aldrich), as an eco-friendly precursor, in combination with ferrocene (98% purity in weight, Sigma Aldrich), which offers the catalytic action for the decomposition of camphor and the build-up of the CNTs (mass ratio: 20
:
1, respectively). When using camphor (C10H16O) as a carbon precursor, the grown CNTs are more vulnerable to functionalization, due to the oxygen contained in the molecule. The aforementioned mixture was heated up to its boiling point (∼260 °C) and the produced vapours were carried in the reactor via the nitrogen flow, which was reduced to 300 mL min−1, during the reaction that was carried out at 850 °C. After the depletion of the precursors mixture, the system was left to room temperature to cool down, maintaining the inert atmosphere. The resulting nanomaterial was collected in the form of CNT forest, that consists of vertically aligned MWCNTs (Fig. 2).
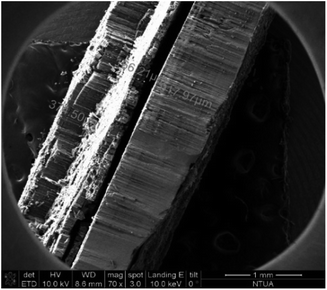 |
| Fig. 2 CNT forest as received by the TC–CVD. | |
Carbon nanotubes purification and functionalization process. The as-received MWCNTs were milled to be transformed from a solid, compact forest to a fine powder. However, due to dispersion issues in saline water, it was decided to test also surface functionalized MWCNTs, that are easier to be dispersed. For this reason, a step by step chemical functionalization procedure took place. Initially, the fine powder of MWCNTs was exposed in air atmosphere at 400 °C for 1 h, in order to remove amorphous carbon residues. Afterwards, MWCNTs were extracted with hydrochloric acid (5 M) in a Soxhlet apparatus, to remove the Fe particles that were embedded in the hollow core of the MWCNTs. Subsequently, washing with distilled water took place in the Soxhlet extractor, till the neutralization of the pH.Mineral acids have been extensively used for surface functionalisation of CNTs, generally as a first simple step of attachment of polar groups to CNT surfaces. By functionalization, the colloidal stability of MWCNTs is increased in polar media like water. Among acids, nitric acid is the most common, in combination with other mineral acids, such as sulfuric, leading to –OH and –COOH attachment to CNT surfaces.29 Thus, to activate the surface of the MWCNTs and enable their dispersion in aqua media, an acid solution mixture of strong acids was utilized, in order to anchor oxygen containing groups (–COOH). Specifically, a mixture of 6 M HNO3
:
H2SO4 with a volume ration of 1
:
3 respectively, was used. The ideal ratio for an acceptable functionalization of MWCNTs was concluded to be 0.15 g CNTs to 10 mL acid solution. This mixture was stirred at 80 °C for 48 h. The functionalized material (MWCNT-COOH) was filtered and the solid was washed with distilled water several times, until neutralization. Finally, washing with ethanol and acetone took place, to dry the material in room temperature.
Carbon nanotubes characterisation methods
The morphology of CNTs was studied via scanning electron microscopy (SEM) and transmission electron microscopy (TEM). For SEM analysis, a Nova NanoSEM 230 (FEI company) microscope with W filament was used, while for TEM analysis, a Tecnai G2 Spirit Twin 12 microscope (FEI) was exploited. A Bruker D8 Advance Twin X-ray diffractometer equipped with a Cu Kα radiation source was used for the crystallinity assessment of CNTs, at a wavelength of 1.5418 Å. Thermogravimetric analysis (TGA) was executed in a Netzsch 409 EP instrument (atmospheric air flow: 120 mL min−1, heating rate: 5 °C min−1) to determine the purity of the produced material. XPS measurements were carried out in a PHI 5000 Versa Probe (Physical Electronics) system, using Al Kα X-ray radiation (hν = 1486.6 eV). Survey and high-resolution spectra were acquired from a 100 μm spot size. The atomic compositions and deconvolution procedures were obtained by Multipak 9.6 software.
Toxicity assessment
The current study tested the toxicity of two CNTs compounds (Table 1). For dissolution of the compounds in seawater or freshwater, sonication was applied (Transsonic 460/H, Elma) and a range of concentrations was then obtained by a series of dilutions. The toxicity experiments applied a range of concentrations, based on a dose–response approach, obtained following a preliminary test using at least five concentrations, each of a different order of magnitude.30 Compounds were weighed and dispersed in an appropriate medium according to the concentration of their active ingredient. For example, in order to achieve 1 mg L−1 compound containing 45% of active ingredient, 1 mg was multiplied by 100/45 = 2.222 and the 2.222 mg was weighed and then dissolved in 1 L of medium. Preliminary tests have been necessary in order to understand the order of magnitude in which the compounds are toxic. Following the preliminary tests, a series of dilutions from the stock-solution were prepared with a dilution factor of no more than two, in order to conduct range-finding tests.30
Table 1 Tested compounds and their chemical specification. Percentage of active compounds is given in parentheses if less than 100% (+ conducted test, − no test conducted)
Compound abbreviation |
Chemical specification |
Toxicity tests |
D. rerio |
A. salina |
D. magnia |
MWCNTs |
Multi-wall carbon nanotubes |
+ |
+ |
− |
MWCNTs-COOH |
Functionalized multi-wall carbon nanotubes, with carboxyl groups |
+ |
+ |
+ |
The following toxicity laboratory experiments were conducted in an incubator (LE-509, mrc) under a 12
:
12 light
:
dark cycle, unless otherwise stated, and using artificial seawater (Instant Ocean®) or freshwater when applicable.
Daphnia magna water flea tests. The toxicity of MWCNTs dispersed in freshwater was tested on D. magna seven days post-hatching (modified from 31). All tested animals were offspring of a similar clutch and maintained in an ADaM medium32 under 21–22 °C and 8
:
16 light
:
dark cycle. The toxicity of MWCNTs-COOH was tested under a concentration range of 0.1–100 mg L−1 and a negative control of ADaM medium, following an hour of sonication of the stock solution.33 One animal was placed in each well of the 12-well tissue-culture plates (JET BIOFIL®), filled with 5 mL of the test solution, with twelve replicates per concentration. The plates were placed on an orbital shaker (70 rpm) in order to maximize dispersion of the MWCNTs while being maintained in an incubator (21 °C and 8
:
16 light
:
dark cycle). The animals were fed daily with Scenedesmus gracilis algae during the experiment (2 million algae per animal) and examined daily under a dissecting microscope for determination of mortality. Three additional plates with animals were set as follows: ADaM medium as control, ADaM medium with S. gracilis algae, and MWCNTs-COOH dispersed in ADaM medium. D. magna, 14 days post-hatching, were individually placed in each well, filled with 5 mL of the test solutions. The plates were placed in an incubator on an orbital shaker (70 rpm) in order to maximize dispersion of the MWCNTs (21 °C and 8
:
16 light
:
dark cycle). During the experiment the animals were fed with S. gracilis (3 million algae per well) and examined after 24 hours under a dissecting microscope. The digestive track of the animals was dissected and their content was preserved in 4% glutaraldehyde for further SEM examination (10 animals per treatment). The samples were stored at 4 °C and then dehydrated in a graded series of ethanol (70, 80, 90 and 100%).34 The samples were dried overnight at 60 °C, glued to stubs, and examined under an Environmental Scanning Electron Microscope (ESEM) (Quanta 200 FEG™, Thermo Scientific™).
Artemia salina nauplii tests. Toxicity tests on A. salina nauplii were conducted on the MWCNT compounds (Table 1) following Vanhaecke et al.22 and Nunes et al.35 The nauplii were obtained by incubating cysts (G 450, Aquazone) in a PVC container filled with 2 L of artificial seawater (salinity of 40 ppt) at room temperature. Each well of the 6-well tissue-culture plates (Costar®, Corning) was filled with 10 mL of the relevant test solution with three replicates per concentration. After 40 hours of incubation the Instar II nauplii were placed in the wells, 10 animals per well.36 The nauplii were tested under a concentration range of 0.0001–100 mg L−1 of the tested compounds dissolved in artificial sea water; with the latter as a negative control. The plates were maintained at 26 °C in an incubator and examined under a dissecting microscope after 24 hours. When no motility was observed for ten seconds, mortality was determined.Toxicity tests of MWCNTs and MWCNTs-COOH were conducted on A. salina nauplii under a concentration range of 0.01–100 mg L−1. During the toxicity tests, nanoparticles in the digestive track of the brine shrimp were visually noted, and therefore, a subsequent experiment was designed in order to examine possible bioaccumulation.37,38 The experiment comprised of three 6-well tissue-culture plates (Costar®, Corning). One was filled with 10 mL of artificial seawater as a control; the second with a dispersion of 100 mg L−1 MWCNTs in artificial seawater; and the third with a dispersion of 100 mg L−1 MWCNTs-COOH in artificial seawater. Following 24 hours of incubation at 26 °C, the plates were examined under a dissecting microscope. Selected animals were preserved in 4% glutaraldehyde for further SEM studies. The rest of the animals were placed in artificial seawater for a 24 h recovery period and then examined under a dissecting microscope as detailed above.
Danio rerio zebrafish tests. The toxicity of the compounds (Table 1) was tested on D. rerio embryos at five concentrations with a negative control of artificial freshwater and a positive control of 3,4-dichloroaniline. The tests were conducted in 24-well tissue culture plates (Costar®, Corning), using a separate plate for each concentration. An internal control was performed in four of the 24 wells and an additional control plate was used for each compound (Fig. 3, adapted from ref. 19 and 20). The wells were filled with 2 mL of the test solutions 24 h prior to onset of the experiments. Solutions of the five concentrations (see: range of concentrations) were introduced into the wells, followed by the positive and the negative controls, and the plates were then placed in an incubator.
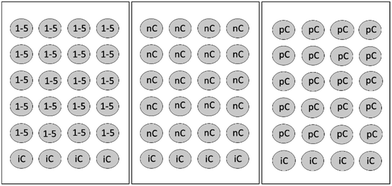 |
| Fig. 3 FET test array: 1–5 = five test concentrations, nC = negative control, iC = internal plate control, pC = positive control (3,4-dichloroaniline 4 mg L−1). Total number of plates: 7 (adapted from ref. 15 and 16). | |
The fertilization procedure followed the OECD protocol.39 Male and female zebrafish at a ratio of 2
:
1 were transferred into spawning tanks, separated by a transparent partition a few hours before the onset of darkness one day prior to the test. Subsequently, mating, spawning, and fertilization took place within 30 min of lights-on and removal of the partitions. Following collection of the fertilized eggs 60 minutes post-fertilization, the embryos were at no more than the 16 cell-stage.
Prior to introducing the embryos into the wells, they were examined under a dissecting microscope to select those that have been fertilized and are developing. These were then transferred into 60 × 15 mm Petri dishes (Nunclon®, Delta) filled with the test solutions (20 embryos per concentration or control). Individual embryos were retrieved from each Petri dish and introduced into the appropriate wells. The plates were incubated under 28 °C (modification of ref. 20). FET lasted for 96 h, with a daily examination of the embryos under a dissecting microscope. Mortality of the embryos was determined according to the following four criteria (indicators): coagulation of embryos; lack of somite formation; non-detachment of the tail; and lack of heart beat (see also OECD 236 protocol). In case of sub-lethal effect of the compounds the embryos were preserved in glutaraldehyde 4% for further microscopic examination. The test was considered valid only when the mortality in the negative control plate was <10% at 96 h of the experiment.
Statistical analysis. Toxicity experimental curves, based on the dose–response method, were calculated and presented according to the OECD guideline.40 Mortality of the fish embryos and crustaceans during exposure to the tested compounds was used to calculate the median lethal concentrations (LC50). LC50 values were determined, following data normalization to the control mean percentage using Abbots formula.41 Afterwards, the percentage values were log transformed using GraphPad Prism® software v.5, through non-linear regression analysis. For each compound and model system non-linear regression equation that best fit the data was selected, considering the R2 value and the 95% confidence intervals.40
Results and discussion
Carbon nanotube properties
After the synthesis, purification and functionalization of MWCNTs, the structure, chemical composition and purity degree were investigated. In Fig. 4a and b the morphology of the synthesized nanostructures is depicted, which has been studied with SEM and TEM, respectively. The SEM results revealed that the produced material consists mainly of long (over 50 μm), wavy and oriented MWCNTs with diameter distribution between 60–100 nm. On the other hand, with the TEM analysis, it was possible to detect the hollow inner structure of the MWCNTs, as well as the iron particles that were embedded in their core, prior the purification process. In Fig. 5 the morphology of the functionalized MWCNTs is depicted. When MWCNTs are functionalized using oxidizing acid mixtures (sulfuric and nitric acids), shortening of their length occurs in relation to the functionalization time;29 for this reason, it was important to define the functionalisation parameters during the experimental procedure, in order to avoid any “cutting” of the CNTs and deterioration of their characteristics. As it can be noticed, despite the fact that MWCNTs appear more entangled, their main structure and orientation have remained. Shortening was not evident, since their length is still above 50 μm.
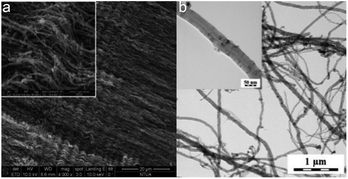 |
| Fig. 4 MWCNTs morphology (a) SEM image in two magnifications, (b) TEM image in two magnifications. | |
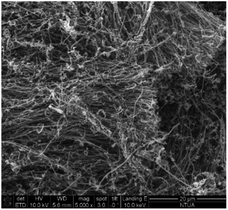 |
| Fig. 5 MWCNTs morphology after functionalization procedure. Length over 50 μm. | |
The initial Fe content of the as received MWCNTs sample was 3.08 wt% according to the Energy Dispersive X-ray Spectroscopy (EDS) analysis (Table 2 and Fig. 6a). The purification procedure that was followed reduced the content of Fe (Fig. 6b) and only 1.27 wt% (0.1% in total) is evident in the sample (Table 2). The oxygen content was notably increased from 3.11 wt% to 8.00 wt%, indication also for the successful introduction of oxygen groups. It has been also indicated that chemical treatments are generally effective in removing non-nanotube carbonaceous species present in the sample. For this reason, the C content was also reduced from 12% to 9.3, since amorphous carbon impurities were existing in the sample.
Table 2 Elemental quantification in terms of weight, atomic percentage and total percentage of pristine and functionalized MWCNTs
Element |
wt% |
at% |
% |
Pristine MWCNTs |
C |
93.17 |
96.60 |
12.0 |
O |
3.11 |
2.42 |
1.3 |
Fe |
3.08 |
0.69 |
0.1 |
Al |
0.64 |
0.30 |
0.1 |
![[thin space (1/6-em)]](https://www.rsc.org/images/entities/char_2009.gif) |
Purified MWCNTs |
C |
90.16 |
93.24 |
9.3 |
O |
8.00 |
6.21 |
1.0 |
Fe |
1.27 |
0.28 |
0.1 |
Al |
0.57 |
0.26 |
0.1 |
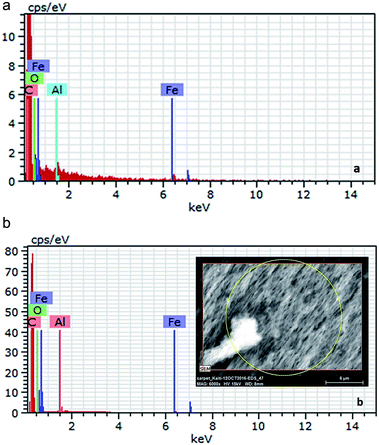 |
| Fig. 6 EDS analysis of as received (a) and purified (b) MWCNTs. | |
From TGA the thermal stability, carbon content and the purity degree of the produced CNTs were also evaluated (Fig. 7). The initial weight loss of 2.01% observed at temperatures up to 480 °C is assigned to the burning of amorphous carbon material. The residual weight% at the end of the thermal oxidative curve was 3.27 and corresponds to the iron catalyst particles, which is in accordance to the EDS analysis. The differential thermogravimetric analysis (DTA) curve showed two peaks at 579.4 °C and 778.36 °C, indicating the high thermal stability in air atmosphere and the uniform graphitized structure of the CNTs produced. The overall purity of the as received material is proved to be around 95 wt%, which increased up to 98% after the purification procedure.
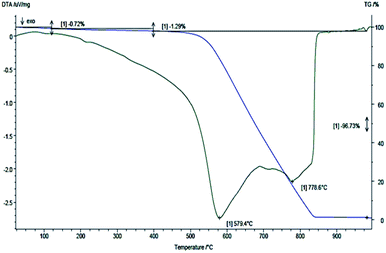 |
| Fig. 7 TGA/DTG curves of pristine MWCNTs obtained under 120 mL min−1 atmospheric air flow and 5 °C min−1 heating rate. | |
Using XPS, the identity and concentration of oxygen containing functional groups formed as a result of MWCNT oxidation can be obtained from spectral deconvolution of the C(1s) XPS regions.42,43 High resolution spectra have been acquired to understand which kind of bonds are involved between C and O (see Fig. 8). The C–C component (sp2 allotropic form) at 284.5 eV is abundant in all the samples and decreases with the chemical functionalisation. The π–π* component is a characteristic shake-up line for carbon in aromatic compounds, generated by the ring excited by photoelectrons.44 The rest components refer to the oxygen-containing groups (–COOH, C–O, C
O). Taking into account all type of bonds, it may be remarked that the relative concentration of O after chemical functionalization increases from 8.4 at% to 20.7 at%, which also confirms the results from the survey spectra (Table 3).
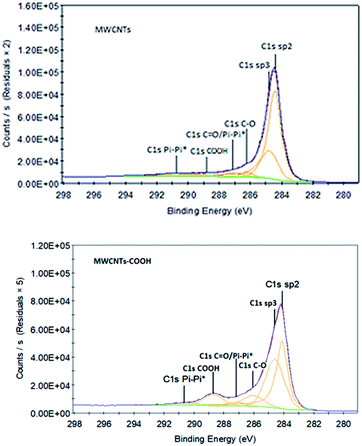 |
| Fig. 8 XPS C 1s high resolution spectra pristine and –COOH functionalized MWCNTs. | |
Table 3 XPS analysis of pristine and –COOH functionalized MWCNTs
Peak |
MWCNTs |
MWCNTs-COOH |
Survey spectra |
C 1s |
97.1 |
79.1 |
O 1s |
2.8 |
18.8 |
![[thin space (1/6-em)]](https://www.rsc.org/images/entities/char_2009.gif) |
C(1s) peak fitting results (type of bonds) |
C 1s sp2 |
65.2 |
39.6 |
C 1s sp3 |
22.2 |
37.3 |
C 1s Pi–Pi* |
4.3 |
2.5 |
C 1s C–O |
2.5 |
9.2 |
C 1s C O/Pi–Pi* |
3.6 |
2.9 |
C 1s COOH |
2.3 |
8.6 |
C–O bonds (%) |
8.4 |
20.7 |
Finally, the crystallinity of the MWCNTs-COOH has been studied with XRD (Fig. 9). The main features of XRD patterns of CNTs are close to those of graphite; a typical XRD pattern consisting of a few broad bands located near the (002), (100) and (110) reflections of graphite. The first peak at 2θ ∼ 26° is attributed to the (002) reflection of graphite while an asymmetric diffraction peak at 2θ ∼ 43° is assigned to (100) reflection of graphite, which is typically observed for MWCNTs.
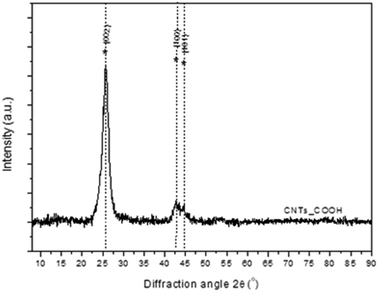 |
| Fig. 9 XRD pattern of functionalized MWCNTs synthesized by CVD. | |
Toxicity tests
Daphnia magna water flea tests. The dissolution of MWCNTs-COOH in artificial seawater even following sonication was very poor and, subsequently, aggregates of the compound appeared on the bottom of the stock solution bottle. No relationship between the survival of D. magna and the MWCNT-COOH concentration was noted and almost all animals survived for 144 h (Fig. 10). These nanoparticles were found in the gut of D. magna, visually noted due to their black color during the test.
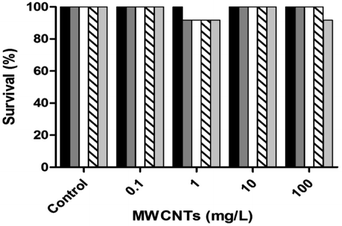 |
| Fig. 10 Percentage survival of Daphnia magna under different concentrations of MWCNTs-COOH over time (n = 12 animals per concentration, black bars – 24 h, gray – 48 h, blank – 72 h, striated – 96 h, and bright gray – 144 h). | |
SEM examination of MWCNTs in the gut of the treated animals revealed none in those exposed only to ADaM medium or in those previously fed with S. gracilis algae (Fig. 11).
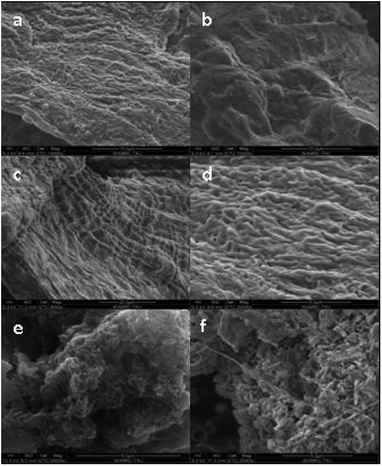 |
| Fig. 11 SEM micrographs of intestine surface of Daphnia magna. (a and b) Under ADaM medium, (c and d) under ADaM medium with Scenedesmus gracilis algae, and (e and f) under MWCNTs-COOH. | |
Artemia salina nauplii tests. The acute A. salina toxicity tests did not exhibit a concentration-dependent mortality. Indeed, CNTs displayed no toxicity up to the highest concentration tested of 100 mg L−1. The nanomaterials tended to sink to the bottom of the well, the MWCNTs notably more than the MWCNTs-COOH. MWCNTs black aggregates were clearly observed in the intestine of A. salina. Following an additional 24 h in seawater the intestine appeared clean and restored to its normal appearance (Fig. 12).
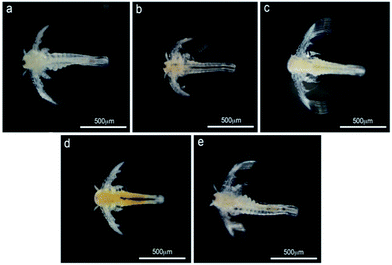 |
| Fig. 12 Artemia salina under different treatments. (a) Control conditions. (b) MWCNTs at concentration of 100 mg L−1, and (c) following 24 h of recovery. (d) MWCNTs-COOH at concentration of 100 mg L−1 and (e) following recovery of 24 h. | |
Danio rerio zebrafish tests. Both MWCNTs and MWCNTs-COOH did not dissolve well in Instant Ocean® seawater, even following sonication, which only slightly improved their dispersion. No relationship was found between the concentration of the compounds and the percentage mortality of embryos under the tested concentrations (Fig. 13).
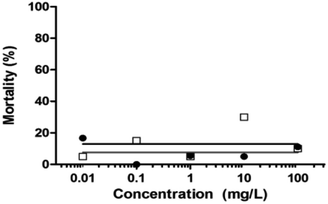 |
| Fig. 13 Percentage mortality of zebrafish embryos under different concentrations of unloaded MWCNTs (black dots) and loaded MWCNT-COOH (blank squares) at 96 h (n = 20 embryos per concentration). | |
The highest mortality occurred under the three highest concentrations of the unloaded MWCNTs after 24 hours (Fig. 14), with that under the lowest concentration occurring after 48 hours. The mortality of the embryos tested under functionalized MWCNTs-COOH did not exceed 40% (Fig. 14b) but was higher than that of the pristine MWCNTs (Fig. 14a). The maximum mortality, at the four highest concentrations, under MWCNTs-COOH was achieved only after either 48 or 72 h.
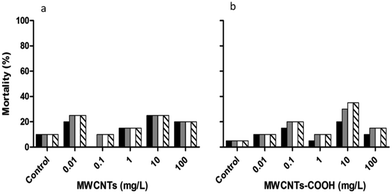 |
| Fig. 14 Percentage mortality of zebrafish embryos under different concentrations of (a) pristine MWCNTs and (b) MWCNT-COOH at different time intervals (n = 20 embryos per concentration, black – 24 h, gray – 48 h, white – 72 h, diagonals – 96 h). | |
The high survival rate of D. magna (Fig. 10) and D. rerio (Fig. 14) under MWCNTs-COOH may indicate their lack of toxicity derived from their poor dissolution, despite the carboxylic groups having improved their dispersion. In the current study, the digestive system of D. magna following MWCNTs-COOH exposure, revealed CNTs during the toxicity tests, and therefore emphasize the need for a more profound visualization of the gut of the tested D. magna (Fig. 12). Similarly, the digestive system of A. salina nauplii featured CNTs, which disappeared after 24 h of recovery (Fig. 13). Petersen and Henry,45 also using microscopic methods, indicated that large masses of CNTs were in the digestive system of numerous organisms, although no study to date has demonstrated the substantial absorption of CNTs in any aquatic organism, which remains in the organism's digestive system, without being excreted. Nonetheless, CNTs might pose a risk to the marine environment in other ways than bioaccumulation.46,47 For example, they are capable of competitively adsorbing metal cations25,26 and aromatic contaminants/pollutants.48 CNTs consequently function as carriers of toxic metal cations whether for uptake by the organisms or through immobilizing cations in the sediment.14 In addition, organisms may directly modify the dispersion of CNTs by ingesting and then excreting them as granules. CNTs can be filtered into D. magna's digestive system and following their excretion have been found to remove the lipid coating used to increase their water solubility, consequently making CNTs less water-soluble and featuring a greater tendency to sink to the sediment.9
Conclusions
In this study, the toxicity of MWCNTs was tested in three model aquatic organisms. The toxicity of MWCNTs could not be determined in relation to their concentration due to their lack of dispersion in the aquatic medium, despite the successful chemical functionalisation that introduced oxygen groups on the surface of the MWCNTs. It was notable, that both kinds of MWCNTs did not lead to mortality of D. magna and A. salina despite their presence in the digestive track of both these non-target organisms. Following an additional 24 h in seawater the intestine appeared clean and restored to its normal appearance. These results show that MWCNTs do not affect the non-target organisms in the short term. However, extensive chronic toxicity testing is a prerequisite so that MWCNTs to be used as a compound in antifouling coatings or nanocomposite materials. In order to use the CNTs as an antifoulant, further research is needed to elucidate the uncertainty of its toxicity, as most importantly, it is already being incorporated into paint prototypes.
Ethical statement
All animal procedures were performed in accordance with the Guidelines for Care and Use of Laboratory Animals of “Tel Aviv University” and Experiments were approved by the Animal Ethics Committee of the “Faculty of Life Sciences” (Approval ID for Zebrafish: 04-16-049).
Conflicts of interest
There are no conflicts to declare.
Acknowledgements
This work was partially funded by the EU FP7 Project “Low-toxic cost-efficient environment-friendly antifouling materials” (BYEFOULING) under Grant Agreement no. 612717 and the H2020 Project “Modified cost effective fibre based structures with improved multi-functionality and performance” (MODCOMP) under Grant Agreement no. 685844. The authors acknowledge members of Prof. Y. Gothilf for assisting in zebrafish mating and embryos collection, M. Weis for technical assistance, V. Wexler for digital editing and N. Paz for editorial assistance. Also, the Carbon Group of Applied Sciences and Technology Department – Politecnico di Torino, under the supervision of Dr Alberto Tagliaferro is greatly acknowledged for the CNTs characterisation data (TGA and XPS analysis).
References
- S. Iijima, Helical Microtubules of Graphitic Carbon, Nature, 1991, 354, 56 CrossRef CAS.
- A. M. Elhissi, W. Abdelbary, I. U. Hassan, V. R. Dhanak and A. D'Emanuele, Carbon Nanotubes in Cancer Therapy and Drug Delivery, J. Drug Delivery, 2012, 2012, 837327 Search PubMed.
- N. Tagmatarchis and M. Prato, Functionalization of carbon nanotubes via 1,3-dipolar cycloadditions, J. Mater. Chem., 2004, 14, 437–439 RSC.
- M. F. De Volder, S. H. Tawfick, R. H. Baughan and A. J. Hart, Carbon Nanotubes: Present and Future Commercial Applications, Science, 2013, 535, 535–540 CrossRef.
- C. A. Charitidis, P. Georgiou, M. A. Koklioti, A. F. A. Trompeta and V. Markakis, Manufacturing nanomaterials: from research to industry, Manuf. Rev., 2014, 1, 11 Search PubMed.
- A. F. A. Trompeta, E. P. Koumoulos, S. G. Stavropoulos, T. G. Velmachos, G. Psarras and C. A. Charitidis, Assessing the Critical Multifunctionality Threshold for Optimal Electrical, Thermal, and Nanomechanical Properties of Carbon Nanotubes/Epoxy Nanocomposites for Aerospace Applications, Aerospace, 2019, 6, 7 CrossRef.
- E. P. Koumoulos, Th. Parousis, A. F. A. Trompeta, I. A. Kartsonakis and C. A. Charitidis, Investigation of MWCNT addition into poly-dimethylsiloxane-based coatings, Plast., Rubber Compos., 2016, 45, 106–117 CrossRef CAS.
- P. Bondavalli, M. B. Martin, L. Hamidouche, A. Montanaro, A. F. A. Trompeta and C. A. Charitidis, Nano-graphitic based non-volatile memories fabricated by dynamic spray-gun deposition method, Micromachines, 2019, 10, 95 CrossRef.
- J. d. S. Filho, E. Y. Matsubara, L. P. Franchi, I. P. Martins, L. M. Rivera, J. M. Rosolen and C. K. Grisolia, Evaluation of Carbon Nanotubes Network Toxicity in Zebrafish (Danio rerio) Model, Environ. Res., 2014, 134, 9–16 CrossRef.
- C. A. Charitidis, A. F. A. Trompeta, N. Vlachou and V. Markakis, Risk management of engineered nanomaterials in EU-The case of carbon nanotubes and carbon nanofibers: a review, Trans. Mater. Res. Soc. Jpn., 2016, 41, 1–11 CrossRef CAS.
- M. Allegri, D. K. Perivoliotis, M. G. Bianchi, M. C. A. Pagliaro, M. A. Koklioti, A. F. A. Trompeta, E. Bergamaschi, O. Bussolati and C. A. Charitidis, Toxicity Determinants of Multi-Walled Carbon Nanotubes: The Relationship between Functionalization and Agglomeration, Toxicol. Rep., 2016, 3, 230–243 CrossRef CAS PubMed.
- J. N. Mwangi, N. Wang, C. G. Ingersoll, D. K. Hardesty, E. L. Brunson, H. Li and B. Deng, Toxicity of carbon nanotubes to freshwater aquatic invertebrates, Environ. Toxicol. Chem., 2012, 31, 1823–1830 CrossRef CAS.
- B. Antizar-Ladislao, Environmental Levels, Toxicity and Human Exposure to Tributyltin (TBT)-Contaminated Marine Environment. a Review, Environ. Int., 2008, 34, 292–308 CrossRef CAS.
- S. Boncelet, J. Kyziol-Komosinska, I. Krzyzewska and J. Czupiol, Interactions of Carbon Nanotubes with Aqueous/aquatic Media Containing Organic/inorganic Contaminants and Selected Organisms of Aquatic Ecosystems – A Review, Chemosphere, 2015, 136, 211–221 CrossRef.
- A. R. Murray, E. R. Kisin, A. V. Tkach, N. Yanamala, R. Mercer, S. H. Young, B. Fadeel, V. E. Kagan and A. A. Shvedova, Factoring-in Agglomeration of Carbon Nanotubes and Nanofibers for Better Prediction of Their Toxicity versus Asbestos, Part. Fibre Toxicol., 2012, 9, 1–19 CrossRef.
- I. Schwyzer, R. Kaegi, L. Sigg and B. Nowack, Colloidal Stability of Suspended and Agglomerate Structures Ofsettled Carbon Nanotubes in Different Aqueous Matrices, Water Res., 2013, 47, 3910–3920 CrossRef CAS.
- P. Jackson, N. R. Jacobsen, A. Baun, R. Birkedal, D. Kühnel, K. A. Jensen, U. Vogel and H. Wallin, Bioaccumulation and Ecotoxicity of Carbon Nanotubes, Chem. Cent. J., 2013, 7, 154–165 CrossRef.
- X. Wang, R. Qu, Q. Huang, Z. Wei and Z. Wang, Hepatic oxidative stress and catalyst metals accumulation in goldfish exposed to carbon nanotubes under different pH levels, Aquat. Toxicol., 2015, 160, 142–150 CrossRef CAS.
- E. Lammer, G. J. Carr, K. Wendler, J. M. Rawlings, S. E. Belanger and Th. Braunbeck, Is the Fish Embryo Toxicity Test (FET) with the Zebrafish (Danio rerio) a Potential Alternative for the Fish Acute Toxicity Test?, Comp. Biochem. Physiol., Part C: Toxicol. Pharmacol., 2009, 149, 196–209 CAS.
- OECD, Guideline for the Testing of Chemicals, Water Solubility, 1995, vol. 1–7 Search PubMed.
- T. Braunbeck, M. Böttcher, H. Hollert, T. Kosmehl, E. Lammer, E. Leist, M. Rudolf and N. Seitz, Towards an Alternative for the Acute Fish LC(50) Test in Chemical Assessment: The Fish Embryo Toxicity Test Goes Multi-Species - an Update, Altex, 2005, 22, 87–102 Search PubMed.
- P. Vanhaecke, G. Persoone, C. Claus and P. Sorgeloos, Research on the Development of a Short Term Standard Toxicity Test with Artemia Nauplii, Agris, 2000, 1, 263 Search PubMed.
- I. Blinova, L. Kanarbik, N. Irha and A. Kahru, Ecotoxicity of Nanosized Magnetite to Crustacean Daphnia magna and Duckweed Lemna Minor, Hydrobiologia, 2017, 798, 141–149 CrossRef CAS.
- K. Tervonen, G. Waissi, E. J. Petersen, J. Akkanen and J. V. Kukkonen, Analysis of Fullerene-c60 and Kinetic Measurements for Its Accumulation and Depuration in Daphnia Magna, Environ. Toxicol. Chem., 2010, 29, 1072–1078 CAS.
- X. Wang, R. Qu, J. Liu, Z. Wei, K. Wang, S. Yang, Q. Huang and Z. Wang, Effect of different carbon nanotubes on cadmium toxicity to Daphnia magna: The role of catalyst impurities and adsorption capacity, Environ. Pollut., 2016, 208, 732–738 CrossRef CAS PubMed.
- X. Wang, R. Qu, A. A. Alam, J. Ajarem, Z. Wei and Z. Wang, Impact of carbon nanotubes on the toxicity of inorganic arsenic [AS(III) and AS(V)] to Daphnia magna: the role of certain arsenic species, Environ. Toxicol. Chem., 2016, 35, 1852–1859 CrossRef CAS.
- D. Ebert, Evolution, Ecology, Epidemiology and Evolution of Parasitism in Daphnia, Bethesda (MD), National Library of Medicine (US), National Center for Biotechnology Information, 2005, available from: http://www.ncbi.nlm.nih.gov/entrez/query.fcgi?db=Books Search PubMed.
- A. F. A. Trompeta, M. A. Koklioti, D. K. Perivoliotis, I. Lynch and C. A. Charitidis, Towards a holistic environmental impact assessment of carbon nanotube growth through chemical vapour deposition, J. Cleaner Prod., 2016, 129, 384–394 CrossRef CAS.
- O. V. Kharissova, B. I. Kharisov and E. G. de Casas Ortiz, Dispersion of carbon nanotubes in water and non-aqueous solvents, RSC Adv., 2013, 3, 24812 RSC.
- F. Blaise, Small-Scale Freshwater Toxicity Investigation, 2005, Springer, http://link.springer.com/10.1007/1-4020-3120-3 Search PubMed.
- OECD, Test No. 202: Daphnia sp. Acute Immobilisation Test, OECD Guideline for the Testing of Chemicals, 2004, section 2, pp. 1–12, http://www.oecd-ilibrary.org/content/book/9789264069947-en Search PubMed.
- B. Klüttgen, U. Dülmer, M. Engels and H. T. Ratte, ADaM, an Artificial Freshwater for the Culture of Zooplankton, Water Res., 1994, 28, 743–746 CrossRef.
- T. Huang, Dispersion of Carbon Nanotubes: Mixing, Sonication, Stabilization, and Composite Properties, Polymers, 2012, 4, 275–295 CrossRef.
- M. Heinlaan, A. Kahru, K. Kasemets, B. Arbeille, G. Prensier and H. C. Dubourguier, Changes in the Daphnia magna Midgut upon Ingestion of Copper Oxide Nanoparticles: A Transmission Electron Microscopy Study, Water Res., 2011, 45, 179–190 CrossRef CAS.
- B. S. Nunes, F. D. Carvalho, L. M. Guilhermino and Van G. Stappen, Use of the Genus Artemia in Ecotoxicity Testing, Environ. Pollut., 2006, 144, 453–462 CrossRef CAS.
- F. Benijts, E. Van Voorden and P. Sorgeloos, Changes in the Biochemical Composition of the Early Larval Stages of the Brine Shrimp, Artemia salina L., Proceedings of the 10th European Symposium on Marine Biology, 1976, vol. 1, pp. 1–9 Search PubMed.
- A. Schierz, B. Espinasse, M. R. Wiesner, J. H. Bisesi, T. Sabo-Attwood and P. Lee Ferguson, Fate of single walled carbon nanotubes in wetland ecosystems, Environ. Sci.: Nano, 2014, 1, 574–583 RSC.
- R. Bjorkland, D. A. Tobias and E. J. Petersen, Increasing evidence indicates low bioaccumulation of carbon nanotubes, Environ. Sci.: Nano, 2017, 4, 747–766 RSC.
- OECD, Draft OECD Guideline for the Testing of Chemicals, 2012, pp. 1–22 Search PubMed.
- OECD, Current Approaches in the Statistical Analysis of Ecotoxicity Data: A Guidance to Application, 2006, vol. 54, pp. 1–147 Search PubMed.
- J. Bellas, K. Granmo and R. Beiras, Embryotoxicity of the Antifouling Biocide Zinc Pyrithione to Sea Urchin (Paracentrotus lividus) and Mussel (Mytilus edulis), Mar. Pollut. Bull., 2005, 50, 1382–1385 CrossRef CAS.
- A. Khan, P. Jagdale, M. Castellino, M. Rovere, Q. Jehangir, P. Mandracci and A. Tagliaferro, Innovative functionalized carbon fibers from waste: How to enhance polymer composites properties, Composites, Part B, 2018, 139, 31–39 CrossRef CAS.
- A. Tagliaferro, M. Giorcelli, A. F. Trompeta and C. A. Charitidis, Investigation og carbon nanotubes surface functionalisation through Raman and XPS analysis, 21st Edition of International Conference on Green Chemistry and Technology, Edinburgh, Scotland, November 12-13, 2018 Search PubMed.
- K. A. Wepasnick, B. A. Smith, J. L. Bitter and D. Howard Fairbrother, Chemical and structural characterization of carbon nanotube surfaces, Anal. Bioanal. Chem., 2010, 396, 1003–1014 CrossRef CAS.
- E. J. Petersen and T. B. Henry, Methodological Considerations for Testing the Ecotoxicity of Carbon Nanotubes and Fullerenes: Review, Environ. Toxicol. Chem., 2012, 31, 60–72 CrossRef CAS.
- P. Laux, C. Riebeling, A. M. Booth, J. D. Brain, J. Brunner, C. Cerrillo, O. Creutzenberg, I. Estrela-Lopis, T. Gebel, G. Johanson, H. Jungnickel, H. Kock, J. Tentschert, A. Tlili, A. Schäffer, A. J. A. M. Sips, R. A. Yokel and A. Luch, Challenges in characterizing the environmental fate and effects of carbon nanotubes and inorganic nanomaterials in aquatic systems, Environ. Sci.: Nano, 2018, 5, 48–63 RSC.
- X. He, W. G. Aker, J. Leszczynski and H. M. Hwang, Using a Holistic Approach to Assess the Impact of Engineered Nanomaterials Inducing Toxicity in Aquatic Systems, J. Food Drug Anal., 2014, 22, 128–146 CrossRef CAS.
- L. Wang, D. Zhu, J. Chen, Y. Chen and W. Chen, Enhanced adsorption of aromatic chemicals on boron and nitrogen co-doped single-walled carbon nanotubes, Environ. Sci.: Nano, 2017, 4, 558–564 RSC.
|
This journal is © The Royal Society of Chemistry 2019 |
Click here to see how this site uses Cookies. View our privacy policy here.