DOI:
10.1039/C9RA06745K
(Paper)
RSC Adv., 2019,
9, 36940-36950
Efficiencies and mechanisms of the chemical cleaning of fouled polytetrafluoroethylene (PTFE) membranes during the microfiltration of alkali/surfactant/polymer flooding oilfield wastewater†
Received
27th August 2019
, Accepted 27th October 2019
First published on 13th November 2019
Abstract
The chemical cleaning of fouled polytetrafluoroethylene (PTFE) membranes with different reagents after the microfiltration of alkali/surfactant/polymer (ASP) flooding oilfield wastewater was examined in this study. Foulant analyses, cleaning efficiencies of different reagents and conditions and cleaning mechanisms were investigated. The results showed that anionic polyacrylamide (APAM) and crude oil were the main membrane foulants accompanied by organic–inorganic–organic/membrane aggregate foulants formed by bridging inorganic ions and organic species. Cleaning efficiency of 93% was acquired through mixed cleaning with 0.04 N NaClO + 200 mg L−1 NaOH, which was found to be better than individual cleaning. Moreover, consecutive cleaning with NaClO + NaOH–HCl restored 98% of the membrane flux, suggesting that HCl cleaning contributed to flux recovery. Additionally, the cleaning temperature and time were set as 40 °C and 3 h, respectively, considering economy and membrane lifespan. Finally, the mechanism of membrane cleaning and analyses of membrane properties were described in this paper, aiming to provide a future direction for production practices. Considering that the cleaning reagents used in this study are easy to obtain and use, consecutive cleaning with NaClO + NaOH–HCl is recommended to clean the PTFE membranes fouled by ASP flooding oilfield wastewater.
1. Introduction
In recent decades, alkali/surfactant/polymer (ASP) flooding oilfield wastewater is being increasingly produced during the oil recovery process.1–3 This wastewater needs to be processed prior to getting discharged into the environment to avoid the risk of severe pollution caused by its organic and inorganic components.1 Many countries that possess oilfields are seeking efficient and cost effective methods to manage this wastewater.4,5 In some oilfields, conventional techniques have been used to treat the wastewater; however, the emulsified and soluble oils in the wastewater cannot be effectively disposed.6 Membrane separation has emerged as a promising alternative for oilfield wastewater treatment due to its superior properties, including good selectivity, strong adaptability, and chemical composition retention.7–9 Microfiltration (MF) is considered to be one of the most effective membrane applications among the membrane separation processes for oilfield wastewater treatment.2,3,10 However, the pH of the wastewater has been observed to exceed the tolerable limit of commonly used MF membranes, which significantly shortens the membrane lifespan and increases operating costs. As an MF membrane, polytetrafluoroethylene (PTFE) is considered to be the best due to its high resistance to strong acids and alkalis, high and low temperatures, and corrosion. Thus, it is preferred for ASP flooding oilfield wastewater treatment.1,2,10–12 However, one of the obstacles impeding the large-scale development of MF is membrane fouling, which accounts for the decrease in membrane permeability or a rise in membrane resistance, resulting in the increase in operation costs and cleaning frequency.13 Although many methods have been adopted to prevent and alleviate membrane fouling, it remains inevitable during the MF process.14–18 Consequently, chemical cleaning becomes a necessary step in the MF process to resume the membrane flux and performance by removing foulants with chemical reagents.19–21
Thus, it is essential to select cost-effective chemical cleaning reagents, which are safe to use, do not produce new foulants, and most importantly do not damage the membrane.22 The commonly used inexpensive acids, bases, and oxidants cause some damage to normal MF membranes, while they cause little damage to the PTFE membranes.2,21 Zhao et al.23 immersed PTFE and polyvinylidene fluoride (PVDF) MF membranes in hydrochloric acid (HCl), sodium hydroxide (NaOH), hydrogen peroxide (H2O2), and sodium hypochlorite (NaClO) solutions for 20 d, 40 d, and 60 d at 40 °C. The results showed that the PTFE membranes exhibited better resistance to chemicals compared to the PVDF membranes and maintained better membrane structural integrity.
Chemical reagents are generally divided into six categories: alkalis, acids, metal chelating agents, surfactants, oxidants, and enzymes.19,20 NaOH is an alkaline cleaning agent, which can saponify fats and dissolve proteins to remove organic foulants, such as grease and pectin.24 Acidic cleaning agents, such as HCl, nitric acid (HNO3), oxalic acid, and citric acid, are mainly used to remove the oxides and hydroxides of calcium and magnesium as well as carbonates and silicates among others.19 NaClO is a low-cost oxidant that is effective in cleaning large molecular foulants, such as polysaccharides and polymers.25 Metal chelating reagents, such as ethylenediaminetetraacetic acid (EDTA), can complex with inorganic ions in foulants to form highly soluble substances, thus reducing the fouling of the deposited salts and adsorbates.26 In addition, surfactants, such as sodium dodecyl sulfate (SDS), are used to remove organic foulants.27
The cleaning efficiencies of a membrane can be affected by individual cleaning reagents or combined cleaning reagents as well as cleaning conditions, which are determined by the properties of different cleaning reagents and their reaction mechanisms with membrane foulants.28 The changes in the quality of raw wastewater can also lead to different membrane fouling situations.29 For example, recent studies have shown that the composition of membrane foulants is significantly different during seawater desalination and wastewater reuse.30 Recently, some studies reported the chemical cleaning of MF membranes.21,31–33 However, many of these studies focused on optimizing the concentration of cleaning reagents, reaction time, and temperature. Research on consecutive cleaning with chemical reagents, especially the cleaning mechanisms for the PTFE membranes used in ASP flooding oilfield wastewater treatments, is lacking.
The main goal of this study was to determine the composition of membrane foulants, investigate the appropriate chemical reagents and their cleaning efficiencies, and discuss the involved cleaning mechanisms of the foulants disengaged from the membrane. The findings from this study can help in resolving the issues related to the chemical cleaning of MF membranes for ASP flooding oilfield wastewater treatment.
2. Materials and methods
2.1. The flat membrane module
The flat membrane module used in this study is shown in Fig. 1, and its characteristics are listed in Table 1.
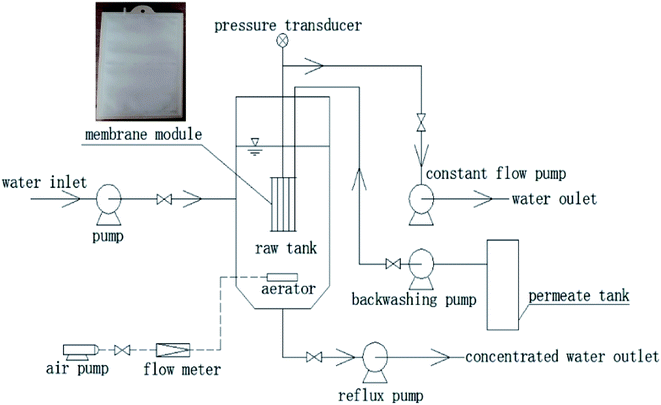 |
| Fig. 1 The schematic of the experimental apparatus. | |
Table 1 Parameters and characteristics of the flat membrane module
Parameter |
Value |
Texture of the membrane |
PTFE |
Texture of the support layer |
Polyethylene terephthalate (PET) |
Manufacturer |
VALQUA Industries (Japan) |
Average pore diameter (μm) |
0.1 |
Effective length and width (cm) |
28 × 18 |
Zeta potential (mV) |
−22.1 |
Contact angle (°) |
120.1 |
2.2. Experimental apparatus
The schematic of the experimental apparatus is shown in Fig. 1. It mainly consisted of a raw wastewater tank of volume 0.06125 m3, a membrane tank of volume 0.018 m3, an effluent tank, constant flow pumps, pressure transducers, and a data acquisition system. The flat membrane modules were vertically put in the membrane tank, which was installed inside the raw wastewater tank. The permeate was sucked from the raw wastewater tank by constant flow pumps (BT600-2J, Longer, China). The transmembrane pressure (TMP) was monitored by pressure transducers (PTP708, Tuopo Electric, Foshan, China), and the data were constantly recorded by a data acquisition software (Siemens).
2.3. Composition of the raw wastewater
The raw wastewater was obtained from an oil production plant in Daqing, China, and its composition is shown in Table S1.† The raw wastewater contained a mass of anionic polyacrylamide (APAM) with the concentration of 749 ± 33 mg L−1, which was used as oil displacement. It also contained organic matter in large quantity, suspended solids (SS), and salts, with the concentrations of 1083 ± 65 mg L−1 of COD, 83 ± 17 mg L−1 of SS, and 5984 ± 450 mg L−1 of TDS, respectively, which might have caused serious fouling on the PTFE membrane. In addition, the pH and temperature of the raw wastewater were 12.6 ± 0.3 and 40 ± 0.7 °C, respectively.
2.4. Membrane fouling and cleaning
The operating parameters (operating flux, backwashing flux, and intensity of gas washing) of MF experiments were kept consistent to subject the membranes to the same degree of fouling. Additionally, MF processes were not terminated until the TMP reached a predetermined value (70 kPa). At the end of the experiments, NaOH, NaClO, HCl, HNO3, SDS, and EDTA solutions with a concentration of 0.5% (wt%) were used separately for immersion at 40 °C for 3 h to chemically clean the fouled PTFE membrane used for ASP flooding oilfield wastewater treatment. Based on preliminary screening, NaClO (0.01–0.05 N), NaOH (50–250 mg L−1), and HCl (0.1–1%) were selected as chemical cleaners for follow-up studies. Furthermore, the effects of cleaning sequence (NaClO + NaOH–HCl or HCl–NaClO + NaOH), temperature (20–50 °C), and time (0.5–5 h) on the cleaning efficiencies were also investigated. After each cleaning cycle, deionized water was filtered again to determine the flux recovery (FR); the calculation process used is shown in eqn (1). |
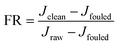 | (1) |
Here, Jclean, Jfouled, and Jraw are the membrane fluxes of the same membrane (or the same membrane module) in its original state, after fouling, and after cleaning, respectively, with the unit L m−2 h−1.
2.5. Analytical methods
The micromorphology of the membrane surface was observed and photographed by scanning electron microscopy (SEM, S-4800, Hitachi, Japan), and the main metal and non-metal elements on the fouled layer of the membrane surface were qualitatively evaluated using the energy dispersive X-ray spectrometer (EDX) on SEM. To retain the spatial morphology of the membrane to the maximum extent possible, the membranes were dried to a freezing state. Before observing the membrane sample, a conductive layer of gold, of approximately 2 nm thickness, was plated on the membrane surface by sputtering (Gatan, USA).1
The functional groups on PTFE membranes were observed by Fourier transform infrared spectroscopy (FTIR, Nicolet 560, Thermo Electron Corp., USA), and the measured wavenumber range was 4000–650 cm−1.34
3. Results and discussion
3.1. Foulant analyses
EDX scanning was performed on the surface of PTFE membranes, as shown in Fig. 2. The mass percentages of the elements found on the membranes are listed in Table S2.† C, O, and F were found to be the basic elements of the PTFE membrane, of which C and F came from the framework of the membrane and O belonged to the hydrophilic groups. The contents of C and O on the fouled membrane compared to that for the original membrane were observed to increase from 36.35% to 42.60% and 8.42% to 11.51%, respectively; the content of F significantly decreased from 55.23% to 21.36%. This indicated that the PTFE membrane was covered by many organic foulants, which may be crude oil, APAM, and surfactants contained in the raw wastewater. Furthermore, many inorganic elements appeared on the fouled membrane. The APAM molecules in the wastewater are negatively charged due to their ionized carboxyl groups. Therefore, positively charged metal ions can bind to the APAM molecules via electrostatic attraction and complexation bridging.35 Another possibility is that the alkalinity of ASP flooding oilfield wastewater was relatively high because it contained a large amount of OH− ions, which might have resulted in the formation of inorganic precipitates, such as Fe(OH)3, Ca(OH)2, and Mg(OH)2.
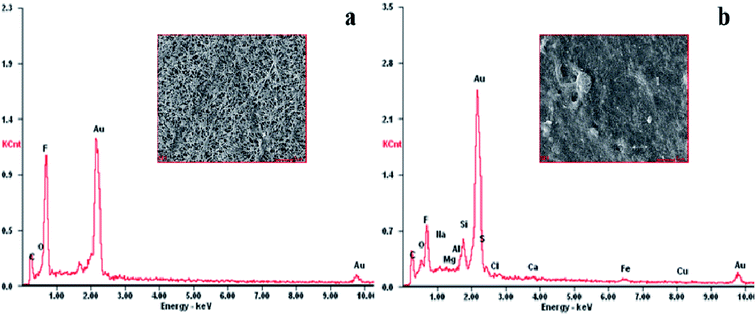 |
| Fig. 2 EDX analyses of the (a) original membrane and (b) fouled membrane. | |
The FTIR spectra of the PTFE membranes are represented in Fig. 3. The infrared absorption spectra of the fouled membrane show several new absorption peaks compared to that of the original membrane, where the peak at 3285 cm−1 represents the N–H bond of amide species, revealing that APAM assembled on the membrane surface.1 Furthermore, the observed absorption peaks of the C–H bond of alkanes, C
C bond of olefins, and methylene groups appeared at 2923 cm−1, 1641 cm−1, and 1412 cm−1, respectively, suggesting the presence of crude oil on the fouled membrane.36 The results obtained here were consistent with those of EDX analyses, implying that APAM and crude oil were the main membrane foulants.
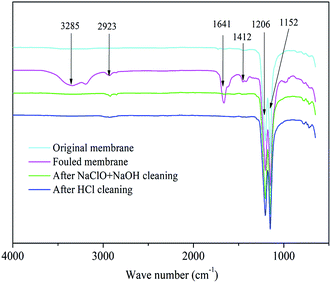 |
| Fig. 3 FTIR spectra of various PTFE membranes. | |
3.2. Comparison of the cleaning efficiencies among different reagents
The membrane fouling of the PTFE membranes used for ASP flooding oilfield wastewater treatment involves a combination of organic and inorganic species with regards to the type of foulants. Moreover, the foulants and the membranes are closely bound by complexation and hydrogen bonding. On-line physical cleaning (backwashing, aeration, etc.) is found to be difficult to completely restore the membrane performance; therefore, chemical cleaning with acids, bases, surfactants, metal chelators, and oxidants is required to remove the foulants that are difficult to wipe off the membrane surface.
3.2.1 Cleaning efficiencies of different individual reagents. The fouled membrane was chemically cleaned by immersing it in NaOH, NaClO, HCl, HNO3, SDS, and EDTA solutions with a finite concentration at 40 °C for 3 h. The flux recoveries with different individual reagents are shown in Fig. 4a. It was observed that these six cleaning reagents could restore the membrane flux, while the HNO3 solution exhibited the worst cleaning efficiency with a flux recovery of only 44.68%. The cleaning results of the HCl, SDS, and EDTA solutions were found to be normal, with the flux recoveries of 52.71%, 49.37%, and 47.32%, respectively. Additionally, the cleaning effects of the NaClO and NaOH solutions were observed to be particularly prominent, with the flux recovery greater than 70%. NaOH and NaClO have been noted to saponify fats and dissolve polymers to remove organic foulants.24,25 However, they are not known to be effective in removing inorganic foulants and metal-containing deposits.37,38 In this study, it was proven that organic fouling was more serious than inorganic fouling on the membrane surface, which is consistent with the conclusion reported in Section 3.1.
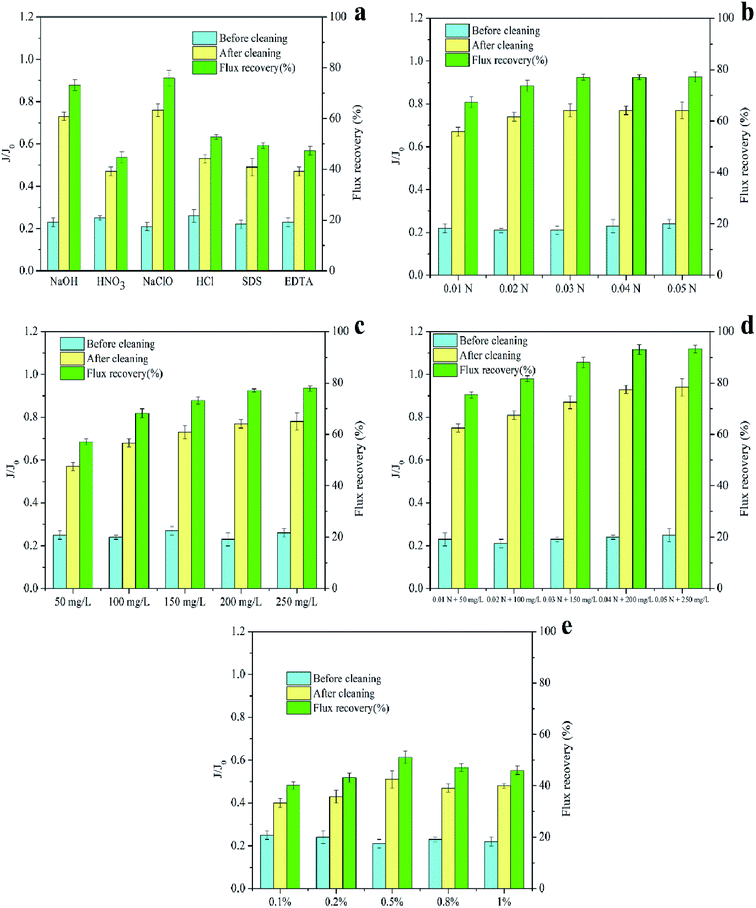 |
| Fig. 4 Cleaning efficiencies of different cleaning reagents (a), NaClO solutions with different concentrations (b), NaOH solutions with different concentrations (c), NaClO + NaOH mixed solutions with different concentrations (d), and HCl solutions with different concentrations (e). | |
Furthermore, the NaClO solution exhibited a better cleaning performance compared to the NaOH solution, which was consistent with the results reported by Ahmad et al.39 and Zhang et al.40 Zhen et al.41 adopted NaOH, SDS, EDTA, HCl, citric acid, and oxalic acid to chemically clean the ultrafiltration membrane treated with oilfield produced water, and the results indicated that NaOH exhibited the best cleaning efficiency. Since the cleaning efficiency of the NaClO solution was not studied, no direct comparison can be drawn with the results of this experiment. Additionally, Wei et al.42 and Grélot et al.43 confirmed that NaClO exhibited the best results for flux recovery among all tested cleaning agents (e.g., NaOH, HCl, citric acid, and EDTA). Furthermore, the cleaning efficiency of alkalis was found to be better than that of acids since the membrane foulants were mainly organic, which was consistent with the results by Norazman et al.44
It was also observed that individual cleaning reagents could not completely remove all membrane foulants; thus, they could not achieve efficient cleaning. Therefore, NaClO, NaOH, and HCl solutions with better cleaning effects were selected as the chemical cleaning reagents in the following study. They were mixed for the consecutive cleaning of fouled membranes to obtain higher flux recovery and regeneration of the fouled membranes.
3.2.2 Cleaning efficiencies of NaClO solutions. The cleaning efficiencies of NaClO solutions having different concentrations for the fouled membrane are shown in Fig. 4b. The flux recoveries were observed to increase from 67.33 ± 2.13% to 77.23 ± 1.93% on increasing the NaClO concentrations. Furthermore, when an MF membrane, fouled by microalgae biomass, was chemically cleaned, the NaClO solution exhibited an excellent cleaning effect.45 NaClO, as a commonly used oxidant, oxidizes the functional groups of organic foulants into ketones, aldehydes, and carboxylic acids to remove organic foulants, especially APAM.28 APAM was one of the major membrane foulants (Section 3.1), which interacted with NaClO. The process of the interaction is expressed in eqn (2)–(6), which can be summarized into three stages: (a) chlorination of the nitrogen atoms in amide groups; (b) separation of hydrogen ions and rearrangement of the N–Cl anions; and (c) conversion of formyl groups into amino groups in polymers.46 |
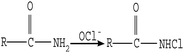 | (2) |
|
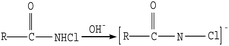 | (3) |
|
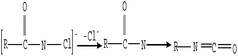 | (4) |
|
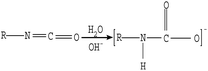 | (5) |
|
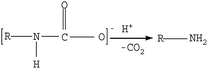 | (6) |
3.2.3 Cleaning efficiencies of NaOH solutions. The flux recoveries were observed to increase to 57.16%, 68.22%, 73.16%, 77.12%, and 77.89% on increasing the NaOH concentrations to 50 mg L−1, 100 mg L−1, 150 mg L−1, 200 mg L−1, and 250 mg L−1, respectively, as shown in Fig. 4c. It removed the organic foulants on the membrane through hydrolysis and solubilization. The hydroxide ions in the alkaline solution promoted the disintegration of the fouled layer mainly by breaking the chemical bonds between the membrane and foulants.28 Moreover, the alkali reacted with crude oil by saponification to form water-soluble micelles.47 During the cleaning process, NaOH primarily acted as a bulking agent and protein solubilizer. The carboxyl groups and the phenolic substances were deprotonated by increasing the solubility and pH of the solution, thus increasing the negative charge of the organic foulants and causing repulsion with the PTFE membrane, which resulted in the separation of the foulants from the membrane.48,49
3.2.4 Cleaning efficiencies of NaClO + NaOH mixed solutions. Fig. 4d shows that the cleaning efficiencies of the NaClO and NaOH mixture with the flux recovery of 93.23% at a concentration of 0.05 N NaClO + 250 mg L−1 NaOH are superior to their individual cleaning efficiencies. Mixing NaClO and NaOH substantially increased the membrane flux to 150% compared to being cleaned individually with NaClO (60%) and NaOH (<10%).47 This was because NaOH changed the configuration of the organic foulants on the membrane and loosened the fouled layer through hydrolysis and solubilization.47 Moreover, our previous studies confirmed that APAM could cause a “shield effect” on crude oil fouling on the PTFE membrane, which implied that APAM was closely bound to the membrane, while the crude oil was present above the APAM fouling layer.35 In such a case, the membrane outer fouling layer was first attacked with the NaOH solution, which then allowed NaClO to react with the innermost APAM relatively easily.50 Additionally, little difference was observed in the flux recoveries between the solutions of 0.04 N NaClO + 200 mg L−1 NaOH and 0.05 N NaClO + 250 mg L−1 NaOH, both of which were found to be above 93%. Considering the expense and membrane lifespan, it was considered more suitable to adopt the concentration of 0.04 N NaClO + 200 mg L−1 NaOH.
3.2.5 Cleaning efficiencies of HCl solutions. The cleaning efficiencies of different concentrations of HCl solutions on fouled membranes are depicted in Fig. 4e. The removal targets of the HCl solution were the inorganic foulants on the fouled membrane. Here, the inorganic foulants included scaling substances, such as CaCO3, Mg(OH)2, SiO2, and Fe oxides, accumulated on the membrane during long-term operation. They also included high-valent metal ions that combined with organics (mainly carboxylic acid groups, sulfonic acid groups, etc.) by forming ionic bonds. The latter played a crucial bridging role in the formation and development of fouling layers on the membrane, especially the gel layer.51–53 Al-amoudi et al.49 verified that the flux of a membrane cleaned with an HCl solution (9.2 kg m−2 h−1) was higher than that of the membrane cleaned without an HCl solution (5.6 kg m−2 h−1). When the concentration of the HCl solution was <0.5%, the flux recoveries were observed to increase from 40.16% to 52.71% on increasing the concentrations of the HCl solution. However, when the concentrations of the HCl solution increased above 0.5%, the flux recoveries showed a decreasing trend. This might be due to the reaction between cleaning reagents and foulants during the cleaning process, which produced some by-products and caused secondary fouling of the membrane.54
3.3. Cleaning efficiencies under different conditions
The flux recovery is not only affected by the type and the concentration of the cleaning reagent, but is also restricted by the cleaning conditions, such as the sequence, temperature, and time.28 Therefore, this section focuses on the effect of different cleaning conditions on flux recoveries.
3.3.1 Effect of sequence on cleaning efficiencies. To improve the efficiency of chemical cleaning, cleaning reagents are often used sequentially, and the cleaning sequence impacts the flux recovery. In this study, fouled membranes were cleaned with the sequence HCl (0.5%)–NaClO + NaOH (0.04 N + 200 mg L−1) and NaClO + NaOH–HCl within 3 h of cleaning with the cleaning duration of each section being 1.5 h. The cleaning efficiencies are shown in Fig. 5. The final cleaning efficiency of the fouled membrane after 3 h for the cleaning sequence NaClO + NaOH–HCl was found to be 99.12%, while for the cleaning sequence HCl–NaClO + NaOH, it was 94.22%. It is important to note that carrying out NaClO + NaOH cleaning first helped in destroying the configuration of the fouling layer and removing the organic foulants in large quantity. Thereafter, the residual metal ions formed due to bridging were removed by HCl cleaning. Afterwards, the organic foulants connected with them fell off from the membrane, thus improving the efficiency of the subsequent HCl cleaning. In contrast, when the fouled membrane was first cleaned with the HCl solution, the compact fouling layer was not easily destroyed by the HCl solution since it could only elute some inorganic scaling substances. Thus, the flux recovery would have been essentially consistent with that of NaClO + NaOH cleaning. Wang et al.28 proved that the cleaning efficiency of consecutive NaClO-citric cleaning was higher than that of the reverse method.
 |
| Fig. 5 Effect of the cleaning sequence on cleaning efficiencies. | |
3.3.2 Effect of temperature and time on cleaning efficiencies. Consecutive cleaning with NaClO + NaOH–HCl was carried out to study the cleaning performance at different cleaning temperatures and times on the fouled membrane, as shown in Fig. 6. It has been shown that the reaction kinetics of the oxidation process and the solubility of the foulants or reaction products as well as the distribution of reagents in a polymer matrix increase on increasing the temperature.28 The flux recoveries were found to be 85.16%, 93.22%, 98.44%, and 99.13% at the cleaning temperatures of 20 °C, 30 °C, 40 °C, and 50 °C, respectively. Zhang et al.55 showed that an increase in temperature was beneficial to the recovery of membrane flux when conducting chemical cleaning of a fouled membrane while treating the wastewater of a banknote printing factory. Bartlett et al.56 found that for two types of membranes, the recoveries of membrane flux increased as the temperature increased from 30 °C to 50 °C under the optimal concentrations of cleaning reagents. However, as the temperature increased, different cleaning reagents performed differently for the MF membrane cleaning. For instance, a commercial cleaner (SurfactronCD50) did not significantly affect flux recoveries at temperatures above 45 °C.57 Therefore, it was reasonable to choose the cleaning temperature of 40 °C in this study.
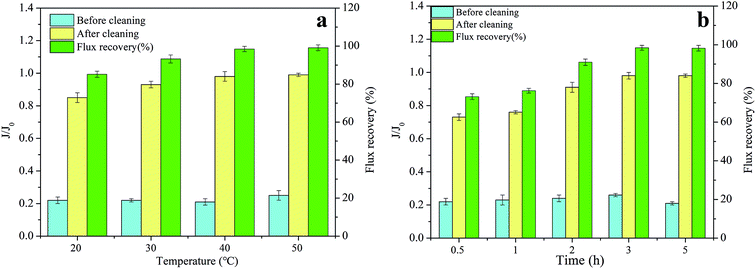 |
| Fig. 6 Effect of the cleaning temperature (a) and time (b) on cleaning efficiencies. | |
The flux recoveries were found to be 73.16%, 76.23%, 90.98%, 98.47%, and 98.24% at the cleaning times of 0.5 h, 1 h, 2 h, 3 h, and 5 h, respectively, indicating that the cleaning efficiencies improved with an extension in cleaning time. However, little difference was noted in the cleaning efficiencies at the cleaning times of 3 h and 5 h. This was because the prolonged cleaning time could only remove the foulants loosely bound to the membrane during the cleaning process and could not elute the foulants tightly adsorbed on the membrane.21 Furthermore, much longer immersion of the membrane in chemical cleaning reagents showed a definite impact on its properties and structure, and shortening the cleaning time helped in extending its lifespan. Thus, setting the cleaning time to 3 h was proven to be more appropriate.
3.4. Proposed mechanism of chemical cleaning
3.4.1 SEM-EDX analysis. The morphology and structure of the PTFE membranes are depicted in Fig. 7. The surface of the original membrane was relatively flat but rough with irregular membrane pores (Fig. 7a). After the MF experiments, the membrane surface was covered with a bumpy gel layer, with most of the membrane pores being blocked (Fig. 7b). However, after 1.5 h of cleaning with 0.04 N NaClO + 200 mg L−1 NaOH, most foulants on the membrane surface were removed (Fig. 7c). According to Table S2,† some metal ions and organics remain on the membrane surface, which might be due to metal ions attaching organic foulants to the membrane through bridging (Section 3.3.1). After 1.5 h of cleaning with 0.8% HCl, the surface morphology of the membrane surface mostly recovered to being smooth and flat, and the membrane pores were clearly visible (Fig. 7d). Additionally, the foulants on the membrane surface were mostly eliminated (Table S2†).
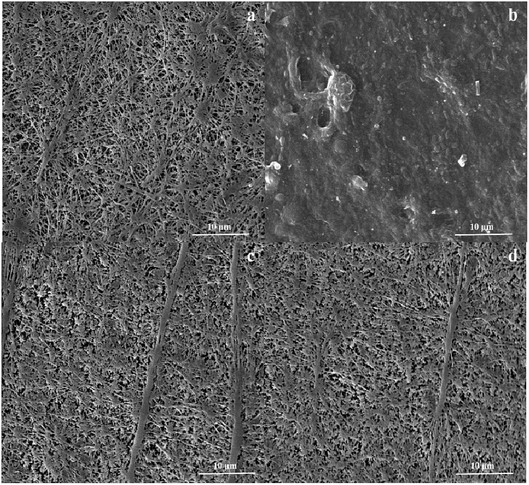 |
| Fig. 7 SEM images of PTFE membranes: (a) original membrane, (b) fouled membrane, (c) fouled membrane cleaned with NaClO (0.04 N) + NaOH (200 mg L−1) mixed solution, and (d) fouled membrane cleaned with HCl (0.8%) solution. | |
3.4.2 FTIR analysis. It can be observed from Fig. 3 that the spectral waveform of the membrane cleaned with NaClO + NaOH is essentially the same as that of the original membrane, indicating that a large proportion of the foulants was eluted and only a very small amount of crude oil remained on the membrane (2923 cm−1). Subsequently, after HCl cleaning of the metal ionic bonds on the membrane, the cleaned membrane looked new and closely resembled the original membrane, which is consistent with the previous results on flux recoveries and SEM (Sections 3.2.5 and 3.4.1), indicating that the cleaning method is very efficient.
3.4.3 Analyses of the membrane properties. The properties of the PTFE membranes obtained before and after chemical cleaning during the five cleaning cycles are displayed in Fig. 8. The determination of the contact angle (CA) is one of the methods used to analyze the characteristic changes in the membrane surface, which reflect the hydrophilicity/hydrophobicity of the membrane.35 Compared to CA of the original membrane, the CA of the fouled membrane became larger, indicating that the uppermost layer of the membrane foulants might consist of hydrophobic materials. According to the composition of raw wastewater (Table S1†), it was speculated that the top of the fouling layer consisted of crude oil, which is consistent with our previous study.35 Furthermore, CA of the PTFE membrane after chemical cleaning under optimum conditions was found to be similar to that of the original membrane (Fig. 8a). It should be especially noted that as the usage time of the membrane increased, irreversible fouling continued to accumulate, resulting in a decrease in the membrane performance. Moreover, repeated cleaning and long-term contact with chemical cleaning reagents would have damaged the membrane structure, increased the pore sizes, and reduced the tensile strength, leading to the failure of stable operation for the membrane reactor as expected by the design (e.g., the quality and yield of the effluent could not meet the design requirements). In addition to CA, the pore size and tensile strength of the cleaned membrane were not found to be significantly different from that of the original membrane (Fig. 8b and c), which illustrated that the chemical properties of the PTFE membrane were relatively stable during the treatment of ASP flooding oilfield wastewater.
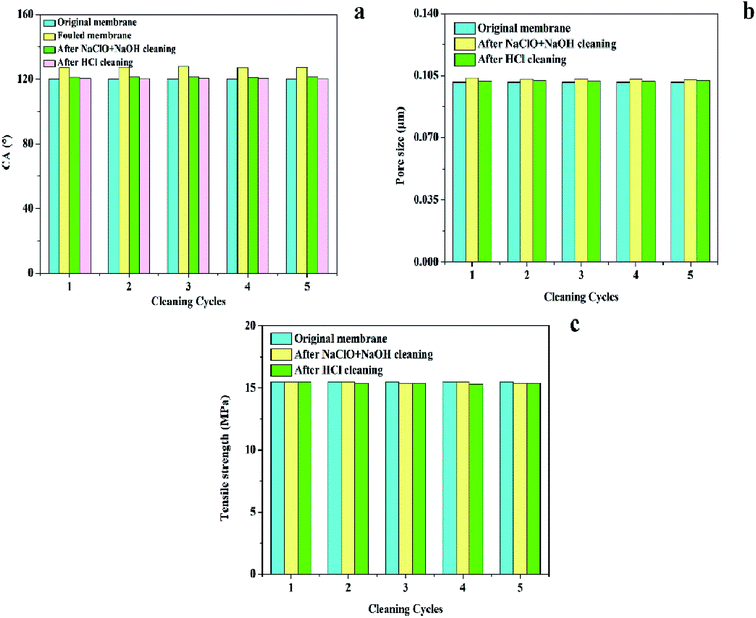 |
| Fig. 8 Changes in membrane properties: (a) contact angle (CA), (b) pore size, and (c) tensile strength. | |
3.4.4 Proposed cleaning mechanism. The proposed mechanisms for the chemical cleaning of the PTFE membrane for ASP flooding oilfield wastewater treatment are depicted in Fig. 9. In the case of the coexistence of APAM and crude oil, APAM first bound to the membrane at a higher concentration with a faster migration speed, and the crude oil covered the surface of the APAM fouling layer.35 Due to high TDS in raw wastewater (Table S1†), the negatively charged APAM combined with oppositely charged ions, which weakened the intramolecular and intermolecular electrostatic repulsions in the APAM molecules;58 thus, APAM on the fouled membrane appeared curly (Fig. 9a). Moreover, inorganic elements, such as Ca and Mg, could tightly bridge curly APAM to the membrane, making the fouling layer stronger. Furthermore, SDBS and precipitates were surrounded by foulants on the membrane and/or adsorbed on the inner wall of the membrane pores (Fig. 9a).
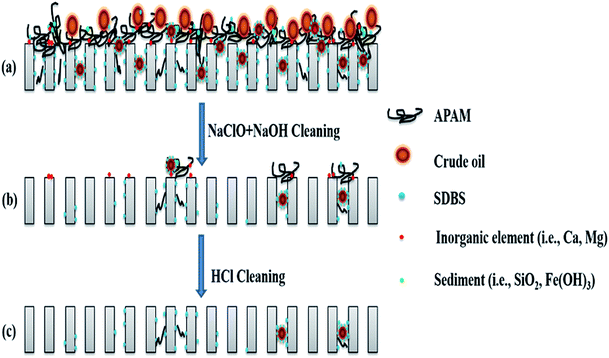 |
| Fig. 9 Schematic of the proposed mechanism of chemical cleaning of the PTFE membrane for ASP flooding oilfield wastewater treatment. | |
The microscopic analyses of the PTFE membranes (Fig. 3 and 7) revealed that consecutive cleaning with NaClO + NaOH–HCl could effectively remove foulants from the PTFE membrane. During the NaClO + NaOH cleaning process, NaOH, primarily as a bulking agent and protein solubilizer, first attacked the outermost membrane fouling layer. Afterwards, NaClO reacted more easily with the innermost APAM. The crude oil, SDBS, and encapsulated inorganic precipitate on the APAM fouling layer were separated from the membrane as the inner layer of APAM disintegrated (Fig. 9b). The residual inorganic elements due to bridging were removed by HCl cleaning. Afterwards, the organic foulants connected with them fell off from the membrane (Fig. 9c).
4. Conclusion
In this study, foulant analyses, cleaning efficiencies of different reagents and conditions and mechanisms during MF of ASP flooding oilfield wastewater were studied. The following conclusions were drawn from the study:
(1) APAM and crude oil were the main membrane foulants accompanied by organic–inorganic–organic/membrane aggregate foulants formed by the bridging of inorganic ions.
(2) A cleaning efficiency of 93% was acquired through mixed cleaning with 0.04 N NaClO + 200 mg L−1 NaOH, which was found to be better than individual cleaning. Moreover, consecutive cleaning with NaClO + NaOH–HCl restored 98% of the membrane flux. Additionally, the cleaning temperature and time were set to 40 °C and 3 h, respectively.
(3) SEM-EDX and FTIR analyses displayed that NaClO + NaOH–HCl consecutive cleaning could effectively remove foulants from the membrane and restore membrane flux.
(4) The properties (CA, pore size, and tensile strength) of the cleaned membrane were not significantly different from that of the original membrane, which illustrated that the chemical properties of the PTFE membranes were relatively stable; also, consecutive cleaning using NaClO + NaOH–HCl was feasible.
Conflicts of interest
There are no conflicts to declare.
Acknowledgements
This work was supported by the National Key Research and Development Program of China (2016YFE0205600), National Natural Science Foundation of China (Grant No. 51778172), and the Innovation Group of New Technologies for Industrial Pollution Control of Chongqing Education Commission (CXQT19023).
Notes and references
- B. Zhang, W. Shi, S. Yu, Y. Zhu, R. Zhang and J. Hwa Tay, J. Colloid Interface Sci., 2019, 544, 303–311 CrossRef CAS PubMed.
- Y. Zhu, S. Yu, B. Zhang, J. Li, D. Zhao, Z. Gu, C. Gong and G. Liu, Sci. Total Environ., 2018, 642, 988–998 CrossRef CAS PubMed.
- B. Zhang, S. Yu, Y. Zhu, W. Shi, R. Zhang and L. Li, RSC Adv., 2016, 6, 62411–62419 RSC.
- A. Fakhru'l-Razi, A. Pendashteh, L. C. Abdullah, D. R. A. Biak, S. S. Madaeni and Z. Z. Abidin, J. Hazard. Mater., 2009, 170, 530–551 CrossRef PubMed.
- J. M. Dickhout, J. Moreno, P. M. Biesheuvel, L. Boels, R. G. H. Lammertink and W. M. de Vos, J. Colloid Interface Sci., 2017, 487, 523–534 CrossRef CAS PubMed.
- Y. Zhang, Y. Xu, S. Zhang, Y. Zhang and Z. Xu, Desalination, 2012, 299, 63–69 CrossRef CAS.
- C. Li, H. Zhang, F. Wang, H. Zhu, Y. Guo and M. Chen, RSC Adv., 2019, 9, 19205–19216 RSC.
- L. Ren, S. Yu, J. Li and L. Li, RSC Adv., 2019, 9, 11111–11122 RSC.
- D. Wu, W. Zhou, X. Cheng, C. Luo, P. Li, F. Zhang and Z. Ren, RSC Adv., 2019, 9, 20035–20043 RSC.
- A. Lin, S. Shao, H. Li, D. Yang and Y. Kong, J. Membr. Sci., 2011, 371, 286–292 CrossRef CAS.
- S.-C. Chen, S.-H. Lin, R.-D. Chien and P.-S. Hsu, J. Hazard. Mater., 2010, 179, 692–700 CrossRef CAS PubMed.
- B. Zhang, S. Yu, Y. Zhu, Y. Shen, X. Gao, W. Shi and J. Hwa Tay, Sep. Purif. Technol., 2019, 116212 Search PubMed.
- X. Sun, D. M. Kanani and R. Ghosh, J. Membr. Sci., 2008, 320, 372–380 CrossRef CAS.
- M. Peydayesh, T. Mohammadi and O. Bakhtiari, Sep. Purif. Technol., 2018, 194, 488–502 CrossRef CAS.
- S. Wang, K. Xiao and X. Huang, Sep. Purif. Technol., 2019, 210, 1008–1016 CrossRef CAS.
- Y. Chung, H. Kim, T.-S. Kim, Y. M. Kim and S. Kang, Sep. Purif. Technol., 2019, 219, 216–221 CrossRef CAS.
- W. Li, G. Ling, F. Lei, N. Li, W. Peng, K. Li, H. Lu, F. Hang and Y. Zhang, Sep. Purif. Technol., 2018, 190, 9–24 CrossRef CAS.
- J. Liu, K. He, S. Tang, T. Wang and Z. Zhang, Sep. Purif. Technol., 2019, 217, 118–127 CrossRef CAS.
- D. Zhao, L. Qiu, J. Song, J. Liu, Z. Wang, Y. Zhu and G. Liu, Sci. Total Environ., 2019, 652, 256–266 CrossRef PubMed.
- G. Liu, L. Li, L. Qiu, S. Yu, P. Liu, Y. Zhu, J. Hu, Z. Liu, D. Zhao and H. Yang, J. Membr. Sci., 2018, 545, 348–357 CrossRef CAS.
- B. Zhang, W. Shi, S. Yu, Y. Zhu, R. Zhang and L. Li, RSC Adv., 2015, 5, 104960–104971 RSC.
- J. Lindau and A.-S. Jönsson, J. Membr. Sci., 1994, 87, 71–78 CrossRef CAS.
- Y. Zhao, L. Hou and S. Yu, China Water & Wastewater, 2013, 29, 134–137 Search PubMed.
- Y. Song, B. Su, X. Gao and C. Gao, Desalination, 2013, 330, 61–69 CrossRef CAS.
- M. S. Manna, P. Saha and A. K. Ghoshal, RSC Adv., 2015, 5, 71999–72008 RSC.
- Q. Li and M. Elimelech, Environ. Sci. Technol., 2004, 38, 4683–4693 CrossRef CAS PubMed.
- P. D. I. Fletcher, L. D. Savory, F. Woods, A. Clarke and A. M. Howe, Langmuir, 2015, 31, 3076–3085 CrossRef CAS PubMed.
- Z. Wang, J. Ma, C. Y. Tang, K. Kimura, Q. Wang and X. Han, J. Membr. Sci., 2014, 468, 276–307 CrossRef CAS.
- M. T. Khan, C.-L. d. O. Manes, C. Aubry and J.-P. Croué, Water Res., 2013, 47, 558–568 CrossRef CAS PubMed.
- M. T. Khan, M. Busch, V. G. Molina, A.-H. Emwas, C. Aubry and J.-P. Croue, Water Res., 2014, 59, 271–282 CrossRef CAS PubMed.
- M. Mouiya, A. Abourriche, A. Bouazizi, A. Benhammou, Y. El Hafiane, Y. Abouliatim, L. Nibou, M. Oumam, M. Ouammou, A. Smith and H. Hannache, Desalination, 2018, 427, 42–50 CrossRef CAS.
- M. M. Bazin, Y. Nakamura and N. Ahmad, J. Teknol., 2018, 80, 95–103 Search PubMed.
- H. Sun, H. Liu, S. Wang, F. Cheng and Y. Liu, Water Res., 2018, 146, 328–336 CrossRef CAS PubMed.
- J. Zhu, M. Tian, Y. Zhang, H. Zhang and J. Liu, Chem. Eng. J., 2015, 265, 184–193 CrossRef CAS.
- R. Zhang, W. Shi, S. Yu, W. Wang, Z. Zhang, B. Zhang, L. Li and X. Bao, Desalination, 2015, 373, 27–37 CrossRef CAS.
- H. Maekawa, G. Ballano, C. Toniolo and N.-H. Ge, J. Phys. Chem. B, 2011, 115, 5168–5182 CrossRef CAS PubMed.
- Y. C. Woo, J. J. Lee, L. D. Tijing, H. K. Shon, M. Yao and H.-S. Kim, Desalination, 2015, 369, 51–61 CrossRef CAS.
- Z. Zhang, M. W. Bligh, Y. Wang, G. L. Leslie, H. Bustamante and T. D. Waite, J. Membr. Sci., 2015, 475, 9–21 CrossRef CAS.
- A. L. Ahmad, N. H. Mat Yasin, C. J. C. Derek and J. K. Lim, J. Taiwan Inst. Chem. Eng., 2014, 45, 233–241 CrossRef CAS.
- X. Zhang, Q. Hu, M. Sommerfeld, E. Puruhito and Y. Chen, Bioresour. Technol., 2010, 101, 5297–5304 CrossRef CAS PubMed.
- X. Zhen, S. Yu, B. Wang and C. Liang, Water Wastewater Eng. Chinese, 2006, 32, 56–59 Search PubMed.
- C.-H. Wei, X. Huang, R. Ben Aim, K. Yamamoto and G. Amy, Water Res., 2011, 45, 863–871 CrossRef CAS.
- A. Grélot, C. Machinal, K. Drouet, A. Tazi-Pain, J. C. Schrotter, A. Grasmick and S. Grinwis, Water Sci. Technol., 2008, 58, 2041–2049 CrossRef.
- N. Norazman, W. Wu, H. Li, V. Wasinger, H. Zhang and V. Chen, Sep. Purif. Technol., 2013, 103, 241–250 CrossRef CAS.
- A. L. Ahmad, N. H. Mat Yasin, C. J. C. Derek and J. K. Lim, Desalination, 2012, 302, 65–70 CrossRef CAS.
- A. El Achari, X. Coqueret, A. Lablache-Combier and C. Loucheux, Macromol. Chem., 1993, 194, 1879–1891 CrossRef CAS.
- C. Liu, S. Caothien, J. Hayes, T. Caothuy, T. Otoyo and T. Ogawa, in Proc. AWWA Water Qual. Technol. Conf., Denver Co., 2000, pp. 1–25 Search PubMed.
- M. D. Kennedy, J. Kamanyi, S. G. S. Rodríguez, N. H. Lee, J. C. Schippers and G. Amy, Adv. Membr. Technol. Appl., 2008, 131–170 CrossRef CAS.
- A. Al-Amoudi and R. W. Lovitt, J. Membr. Sci., 2007, 303, 4–28 CrossRef CAS.
- H. Liang, W. Gong, J. Chen and G. Li, Desalination, 2008, 220, 267–272 CrossRef CAS.
- X. Wang and T. D. Waite, Environ. Sci. Technol., 2009, 43, 9341–9347 CrossRef CAS PubMed.
- K. Xiao, Y. Shen and X. Huang, J. Membr. Sci., 2013, 427, 139–149 CrossRef CAS.
- L.-F. Wang, D.-Q. He, W. Chen and H.-Q. Yu, Water Res., 2015, 81, 325–332 CrossRef CAS PubMed.
- S. S. Madaeni and S. Samieirad, Desalination, 2010, 257, 80–86 CrossRef CAS.
- G. J. Zhang, Z. Z. Liu, L. F. Song, J. Y. Hu, S. L. Ong and W. J. Ng, Desalination, 2004, 170, 271–280 CrossRef CAS.
- M. Bartlett, M. R. Bird and J. A. Howell, J. Membr. Sci., 1995, 105, 147–157 CrossRef CAS.
- S. H. D. Silalahi and T. Leiknes, Desalination, 2009, 236, 160–169 CrossRef CAS.
- G. Liu, S. Yu, H. Yang, J. Hu, Y. Zhang, B. He, L. Li and Z. Liu, Environ. Sci. Technol., 2016, 50, 1393–1402 CrossRef CAS PubMed.
Footnote |
† Electronic supplementary information (ESI) available. See DOI: 10.1039/c9ra06745k |
|
This journal is © The Royal Society of Chemistry 2019 |
Click here to see how this site uses Cookies. View our privacy policy here.