DOI:
10.1039/C8SC04162H
(Edge Article)
Chem. Sci., 2019,
10, 1144-1149
Ni-catalyzed migratory fluoro-alkenylation of unactivated alkyl bromides with gem-difluoroalkenes†
Received
19th September 2018
, Accepted 8th November 2018
First published on 9th November 2018
Abstract
We describe a nickel-catalyzed highly regio- and stereoselective migratory fluoro-alkenylation of unactivated alkyl bromides. A unique catalytic cycle merging alkyl nickel chain-walking and defluorinative coupling enables the introduction of a broad array of fluoroalkenyl moieties into carbon chains. Control experiments with other halogenated alkenes demonstrated the essential role of fluorine atoms in this reaction. Notably, the reaction proceeds under mild conditions and allows for the synthesis of a variety of valuable monofluoroalkenes.
Introduction
Cross-electrophile coupling has found wide application in the construction of C–C bonds and serves as a powerful and reliable alternative to the classical nucleophile/electrophile procedures.1 In particular, C(sp2)–C(sp3) cross-coupling has been thoroughly explored using the combination of aryl/alkenyl and alkyl electrophiles, wherein β-hydride elimination is inhibited to minimize olefin by-products.2 Conversely, transition-metal-catalyzed remote functionalization exploits iterative β-hydride elimination and metal-H insertion, namely the “chain-walking” process, allowing introduction of functionality into an otherwise unreactive aliphatic position.3 Recently, significant progress in this field has been achieved by the groups of Martin,4 Zhu,5 and Yin.6 Remote arylation and carboxylation of unactivated alkyl halides have been well established (Scheme 1a). However, the reported reactions are still restricted to a relatively limited number of coupling partners, thus limiting the resulting molecular diversity of the products. A notable limitation remains: the corresponding alkenylation of unactivated alkyl halides has not been reported yet, although various alkenes are frequently found in pharmaceuticals or other functional molecules. The reason for the lack of a precedent probably lies in the potential addition of disassociated metal-H to the olefinic coupling component and even the alkene product during the chain-walking process.5,7 In this regard, the development of efficient and practical routes for metal-hydride-involving remote alkenylation has remained as a formidable challenge.
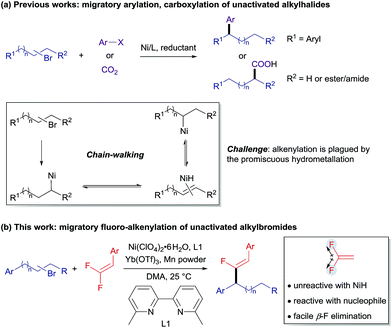 |
| Scheme 1 Migratory functionalization of unactivated alkyl halides. | |
To circumvent the problem of promiscuous hydrometallation, an alkenyl coupling partner inherently inert to metal-H would be required in a successful migratory alkenylation. Recently, gem-difluoroalkenes have been demonstrated by Cao, Toste, Fu, our group, and others as efficient fluoro-alkenylating reagents to access a wide range of monofluoroalkenes using radical or ionic manifolds.8 The strong electron-withdrawing nature of the two fluorine atoms renders gem-difluoroalkenes highly reactive toward nucleophilic attack on the fluorinated sp2-carbon, and fluoro-alkenylation could be accomplished through facile β-fluoride elimination. Furthermore, we considered that the fluorine-based polarization and p–π interaction would make the fluorinated olefins less favored in the hydrometallation compared with the non-fluorinated alkene intermediates and therefore amenable in the migratory alkenylation reactions.9 It could then be envisaged that gem-difluoroalkene 2 can coordinate to thermodynamically stable benzyl nickel V (generated through oxidative addition of the C–Br bond to the Ni0 and single-electron reduction followed by chain-walking) to form intermediate VI, which undergoes regioselective migratory insertion to produce a carbo-nickelation adduct (VII).5 Subsequently, β-fluoride elimination could lead to the formation of the monofluoroalkene 3.10 Reduction of Ni–F (VIII) could then regenerate the catalytically active Ni0 species (I) (Scheme 2). However, we anticipated that a few challenges would have to be overcome to realize this strategy: (1) a nickel catalyst must be able to mediate all the elementary steps including chain-walking and defluorinative coupling; (2) the resulting monofluoroalkene product should be unreactive towards the Ni–H as well as Ni-alkyl species; (3) the migration of Ni–H along the carbon chain should be much faster than the alkenylation step to ensure good regioselectivity. In this report, we disclose such a convenient synthetic route for the migratory fluoro-alkenylation of unactivated alkyl bromides with high regio- and stereocontrol (Scheme 1b). Remarkably, by virtue of this operationally simple methodology a variety of structurally privileged monofluoroalkenes11 could be obtained from easily available materials.
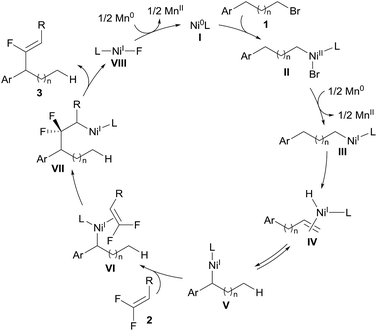 |
| Scheme 2 Reaction design. | |
Results and discussion
To test our hypothesis, we selected gem-difluoroalkene 2a as an alkenylating reagent to react with 1-bromo-2-phenylethane 1a (Table 1). After careful evaluation of the reaction parameters, we found that a combination of inexpensive and bench-stable Ni(ClO4)2·6H2O as a pre-catalyst, 6,6′-dimethyl-2,2′-bipyridyl (L1) as a ligand, and Mn as a reducing agent to generate Ni0 in DMA at 25 °C gave the benzylic fluoro-alkenylation product 3a with Z-configuration in 28% NMR yield within 12 hours (entry 1).12 Pleasingly, the product was obtained with excellent regioselectivity and stereoselectivity. Furthermore, it was found that introduction of MgCl2 as an additive13 gave an improved yield (44%), thus indicating that the Lewis acid has a positive effect on the reaction yield. Encouraged by this result, extensive screening of Lewis acid additives was conducted (Table S1†), and Yb(OTf)3 led to a favorable result (70% yield). The application of YbCl3 afforded 3a in comparable yield (63%), reflecting the importance of the ytterbium cation (entry 4). On the other hand, changing the reducing agents to Zn, B2pin2 or HCOONa had a deleterious effect on the outcome of the reaction (Table S1†). Moreover, Ni(ClO4)2·6H2O proved to be the optimal catalyst after examination of various nickel salts (Table S1†). Subsequently, the ligand was further optimized. Increasing the steric profile of the substituent at the ortho position to the nitrogen in the bipyridyl scaffold (L2) hampered the reactivity of this alkenylation reaction, and no product was detected when the reaction was treated with bipyridyl (L3) as a ligand, suggesting the essential role of such substituents (entries 5 and 6). The structural analogue neocuproine (L4) also exhibited high catalytic efficiency while bathocuproine (L5) was less effective (entries 7 and 8). Slightly higher yields of 3a were obtained when the reaction was treated with fewer equivalents of Mn (entry 9). Of particular note, the process is readily scaled up, the reaction of 1a (2.5 mmol) with 2a (1.0 mmol) gave 3a in 70% yield (entry 10).
Table 1 Optimization of reaction conditionsa
Unless otherwise noted, reactions were carried out with 0.5 mmol of 1a, 0.2 mmol of 2a, 5 mol% of Ni(ClO4)2·6H2O, 6 mol% of ligand, 0.5 mmol of Mn powder and 0.1 mmol of additive in 1 mL of DMA at 25 °C for 12 h.
Determined by 1H NMR versus an internal standard.
The regioisomeric ratio was higher than 100 : 1 according to 19F NMR of the crude product.
0.48 mmol of Mn powder was used.
Isolated yield.
1.0 mmol scale reaction.
|
|
By using the optimized reaction conditions, the scope of this Ni-catalyzed migratory fluoro-alkenylation of unactivated alkyl bromide with gem-difluoroalkenes was evaluated. As shown in Scheme 3, the present protocol shows a remarkably broad scope with respect to the gem-difluoroalkene coupling partner. 1-Aryl-2-bromoethane was initially coupled with various aryl-gem-difluoroalkene derivatives to afford the fluoro-alkenylation products (3a–r). The method tolerated a variety of substitution patterns on the phenyl group. In general, the reaction displayed a noticeable preference for substrates bearing electron-withdrawing groups, such as cyano, trifluoromethyl, ester and ketone, which is in sharp contrast to radical-associated alkylation wherein electron-rich gem-difluoroalkenes were favored.8i For example, para-substituted phenyl-gem-difluoroalkenes were converted into the corresponding products (3a, 3d, 3g, 3j and 3k), mostly in moderate to good yields. The use of meta-substituted phenyl-gem-difluoroalkenes was also explored, which led to moderate yields of products (3b, 3e and 3h). Notably, sterically demanding starting materials that bear ortho-substituents on the phenyl ring were amenable under the optimized reaction conditions; however, the yields of the desired products were reduced (3c, 3f and 3i). Chlorine and fluorine substituents were also compatible in the present reaction (3l and 3m). Moreover, aryl-gem-difluoroalkenes with electron-donating substitution, including OMe, OTs and even the protic amide NHAc, were employed, and these transformations took place smoothly, leading to the compounds 3n–3p with moderate yields. Additionally, nitrogen-containing heterocycle derived substrates could also be converted by the catalytic system (3q and 3r). We next aimed to extend the scope of alkyl bromides. A range of β-bromoethylarenes having Me, OMe, OTBS, Cl and CF3 groups on the phenyl ring were subjected to the alkenylation reaction with an acetyl or a methoxylcarbonyl phenyl-gem-difluoroalkene (2a and 2j). The products 3s–3z were obtained in useful yields with excellent regioselectivity and stereoselectivity. It is worth mentioning that an unprotected hydroxyl group was also tolerated, despite affording the product in decreased yield. To further expand the scope of the alkyl bromides, long range alkenylation was then examined. Alkenylation occurred favorably at the benzylic position, providing the corresponding fluoro-alkenylated propane, butane and even pentane derivatives (3aa–ac). The yields decreased progressively as the carbon chain increased which might be attributed to the increased bulkiness of the benzyl–nickel intermediate. The yields of other related regioisomers were only slightly increased according to 19F NMR analysis of the crude product. These results suggest that formation of the benzyl-Ni species is faster compared to the alkenylation step. Then the more challenging secondary alkyl bromide was subjected to an alkenylation reaction with 2j, and proved to be competent in this reaction, providing the expected benzylic fluoro-alkenylation product with good regioselectivity. The relatively lower yield of 3aa from the secondary alkyl bromide (35% and 30% from (2-bromobutyl)benzene and (3-bromobutyl)benzene, respectively) than the primary one (40% from (4-bromobutyl)benzene) may indicate that the oxidative addition of the alkyl bromide to nickel is sensitive to the steric bulkiness, thus attenuating the reaction efficiency.
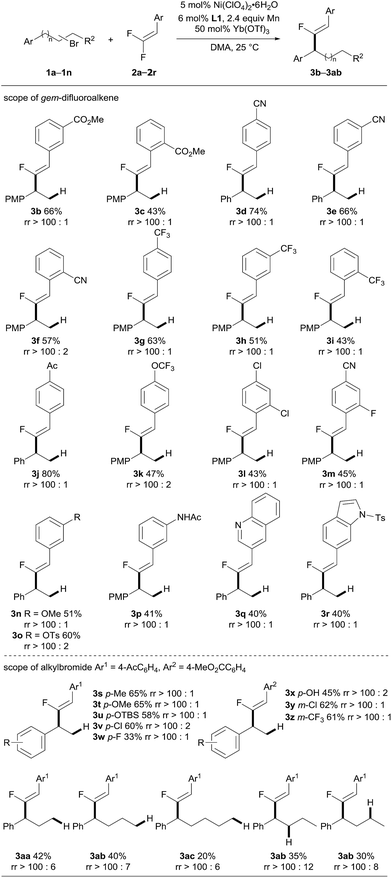 |
| Scheme 3 Substrate scope. See the ESI† for experimental details. Isolated yields are indicated. The regioisomeric ratio (rr, the ratio of the benzylic fluoro-alkenylation product to the other regioisomers) was determined by 19F NMR analysis of the crude product. PMP = p-methoxyphenyl. Ts = tosyl. TBS = tert-butyldimethylsilyl. | |
The synthetic utility of the fluoro-alkenylation product was exemplified by further transformations of 3a (Scheme 4). Hydrogenation of the fluoroalkene moiety contained within 3a was carried out (H2, Pd/C), yielding 4a. An epoxidation reaction using 3a also proceeded well to give fluoroepoxide 4b with high yield (88%). In addition, dibromination of the C
C double bond with bromine was executed and the addition product 4c was formed uneventfully. Moreover, treatment of 3a with a base to eliminate HF furnished the synthetically useful trisubstituted allene 4d in 70% yield. This sequence allows gem-difluoroalkenes to serve as a vinylidene source, and thereby an expedient migratory vinylidenation was achieved. Finally, we found that 3a could be converted into 1,2-diketone 4e by oxidation.
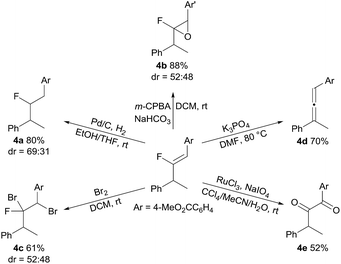 |
| Scheme 4 Synthetic applications. | |
To gain preliminary insight into the unique fluorine effects14 that enable the migratory fluoro-alkenylation reaction, several control experiments were then performed. First, we carried out the alkenylation reaction with a series of halogenated alkenes (Scheme 5a–c). As shown in Scheme 5a, gem-chloroalkene 5a and gem-dibromoalkene 5b did not lead to the desired halo-alkenylation product. Second, we examined monohaloalkenes under the standard conditions. Not surprisingly, in these experiments no alkenylation product was detected again upon the consumption of the starting materials 7a–c (Scheme 5b).15 The comparison with various halogenated alkenes highlights the prominent role of the two fluorine atoms which entail the unique reactivity in migratory fluoro-alkenylation. Regarding the C(sp2)–C(sp3) bond formation, an alternative pathway involving oxidative addition of the C(sp2)–F bond to the benzyl–Ni followed by reductive elimination is also possible.8c,e However, the 1-bromo-1-fluoroalkene 9 failed to produce the fluoro-alkenylation product which could tentatively rule out this mechanism (Scheme 5c).16 The use of deuterium labelled alkyl bromide D2-1a gave rise to the deuterium-shift product D2-3a exclusively, which strongly supports idea that a process involving β-hydride elimination and reinsertion is operative in the present transformation (Scheme 5d). Note that no significant further hydrogen/deuterium scrambling was found in D2-3a, revealing the thermodynamic preference of the benzylic-Ni intermediate in the migration process, which intrinsically dictates the regioselectivity of this transformation. Given the Ni-catalyzed chain-walking process does not require a Lewis acid additive,4–6 it is reasonable to attribute the role of Yb(OTf)3 in activating gem-difluoroalkene towards the nucleophilic addition17 or facilitating the reduction of Ni–F species.18 To obtain additional insight into the influence of Yb(OTf)3, reactions with a stoichiometric amount of Ni(ClO4)2·6H2O/L1 were carried out (see the ESI† for details). In the reaction with Yb(OTf)3, the desired product (3a) was attained in 55% yield which is much higher than the ytterbium-free reaction (16%), implying that the Lewis acid should take effect in the nucleophilic addition step rather than the Ni–F reduction. To evaluate the ability of Ni–H to recognize the non-fluorinated alkenes and gem-difluoroalkenes, styrene 10 was subjected to the fluoro-alkenylation reaction with 1-bromopropane as the hydride source (Scheme 5e).5,19 With the same catalytic system, the desired product 3a was formed in 40% NMR yield. This result clearly demonstrates the significant impact of fluorine substituents on the alkene moiety, which differentiate the two kinds of alkenes towards the Ni–H species as proposed in Scheme 1b.
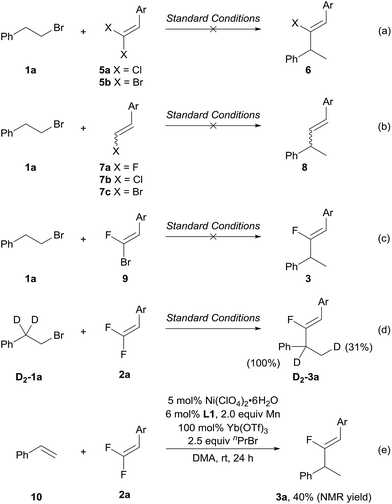 |
| Scheme 5 Control experiments (Ar = 4-MeOCOC6H4). | |
Conclusions
In conclusion, a general, Ni-catalyzed migratory fluoro-alkenylation of unactivated alkyl bromides with gem-difluoroalkenes has been developed, providing ready access to diversely functionalized monofluoroalkenes, which are valuable molecules in biological and materials science. More importantly, this work extends the boundaries of the highly attractive field of remote functionalization of unactivated alkyl electrophiles since it represents the first instance in which an alkene coupling partner has been used in a NiH-mediated process. It is also noteworthy that this C(sp2)–C(sp3) bond-forming reaction proceeds with excellent regio- and stereoselectivity under nonbasic conditions at room temperature, and an array of potentially reactive functional groups are tolerated.
Conflicts of interest
There are no conflicts to declare.
Acknowledgements
The work was supported by the “Thousand Talents Plan” Youth Program, the “Jiangsu Specially-Appointed Professor Plan”, the Natural Science Foundation of Jiangsu Province (BK20170984 and BK20170992), the Start-up Grant from Nanjing Tech University and the SICAM Fellowship from Jiangsu National Synergetic Innovation Center for Advanced Materials.
Notes and references
-
(a) D. A. Everson and D. J. Weix, J. Org. Chem., 2014, 79, 4793 CrossRef CAS PubMed;
(b) C. E. Knappke, S. Grupe, D. Gartner, M. Corpet, C. Gosmini and A. Jacobi von Wangelin, Chem.–Eur. J., 2014, 20, 6828 CrossRef CAS PubMed;
(c) D. J. Weix, Acc. Chem. Res., 2015, 48, 1767 CrossRef CAS PubMed.
-
(a) T. Moragas, A. Correa and R. Martin, Chem.–Eur. J., 2014, 20, 8242 CrossRef CAS PubMed;
(b) E. L. Lucas and E. R. Jarvo, Nat. Rev. Chem., 2017, 1, 65 CrossRef.
- For reviews, see:
(a) I. Franzoni and C. Mazet, Org. Biomol. Chem., 2014, 12, 233 RSC;
(b) A. Vasseur, J. Bruffaerts and I. Marek, Nat. Chem., 2016, 8, 209 CrossRef CAS PubMed;
(c) H. Sommer, F. Juliá-Hernández, R. Martin and I. Marek, ACS Cent. Sci., 2018, 4, 153 CrossRef CAS PubMed ; for selected examples, see:;
(d) L. Ilies, Q. Chen, X. Zeng and E. Nakamura, J. Am. Chem. Soc., 2011, 133, 5221 CrossRef CAS PubMed;
(e) E. W. Werner, T.-S. Mei, A. J. Burckle and M. S. Sigman, Science, 2012, 338, 1455 CrossRef CAS PubMed;
(f) T.-S. Mei, E. W. Werner, A. J. Burckle and M. S. Sigman, J. Am. Chem. Soc., 2013, 135, 6830 CrossRef CAS PubMed;
(g) T.-S. Mei, H. H. Patel and M. S. Sigman, Nature, 2014, 508, 340 CrossRef CAS PubMed;
(h) E. Larionov, L. Lin, L. Guénée and C. Mazet, J. Am. Chem. Soc., 2014, 136, 16882 CrossRef CAS PubMed;
(i) H. H. Patel and M. S. Sigman, J. Am. Chem. Soc., 2015, 137, 3462 CrossRef CAS PubMed;
(j) L. Lin, C. Romano and C. Mazet, J. Am. Chem. Soc., 2016, 138, 10344 CrossRef CAS PubMed;
(k) S. Aspin, A.-S. Goutierre, P. Larini, R. Jazzar and O. Baudoin, Angew. Chem., Int. Ed., 2012, 51, 10808 CrossRef CAS PubMed;
(l) S. Dupuy, K.-F. Zhang, A.-S. Goutierre and O. Baudoin, Angew. Chem., Int. Ed., 2016, 55, 14793 CrossRef CAS PubMed;
(m) A. J. Borah and Z. Shi, J. Am. Chem. Soc., 2018, 140, 6062 CrossRef CAS PubMed;
(n) Y. He, Y. Cai and S. Zhu, J. Am. Chem. Soc., 2017, 139, 1061 CrossRef CAS PubMed;
(o) J. Xiao, Y. He, F. Ye and S. Zhu, Chem, 2018, 4, 1645 CrossRef CAS;
(p) F. Zhou, J. Zhu, Y. Zhang and S. Zhu, Angew. Chem., Int. Ed., 2018, 57, 4058 CrossRef CAS PubMed.
-
(a) F. Juliá-Hernández, T. Moragas, J. Cornella and R. Martin, Nature, 2017, 545, 84 CrossRef PubMed;
(b) M. Gaydou, T. Moragas, F. Juliá-Hernández and R. Martin, J. Am. Chem. Soc., 2017, 139, 12161 CrossRef CAS PubMed.
- F. Chen, K. Chen, Y. Zhang, Y. He, Y.-M. Wang and S. Zhu, J. Am. Chem. Soc., 2017, 139, 13929 CrossRef CAS PubMed.
-
(a) L. Peng, Y. Li, Y. Li, W. Wang, H. Pang and G. Yin, ACS Catal., 2018, 8, 310 CrossRef CAS;
(b) L. Peng, Z. Li and G. Yin, Org. Lett., 2018, 20, 1880 CrossRef CAS PubMed.
-
(a) I. Pappas, S. Treacy and P. J. Chirik, ACS Catal., 2016, 6, 4105 CrossRef CAS;
(b) N. G. Léonard and P. J. Chirik, ACS Catal., 2018, 8, 342 CrossRef.
-
(a) H. Amii and K. Uneyama, Chem. Rev., 2009, 109, 2119 CrossRef CAS PubMed;
(b) X. Zhang and S. Cao, Tetrahedron Lett., 2017, 58, 375 CrossRef CAS;
(c) W. Dai, J. Xiao, G. Jin, J. Wu and S. Cao, J. Org. Chem., 2014, 79, 10537 CrossRef CAS PubMed;
(d) P. Tian, C. Feng and T.-P. Loh, Nat. Commun., 2015, 6, 7472 CrossRef PubMed;
(e) Y. Xiong, T. Huang, X. Ji, J. Wu and S. Cao, Org. Biomol. Chem., 2015, 13, 7389 RSC;
(f) R. T. Thornbury and F. D. Toste, Angew. Chem., Int. Ed., 2016, 55, 11629 CrossRef CAS PubMed;
(g) W. Dai, H. Shi, X. Zhao and S. Cao, Org. Lett., 2016, 18, 4284 CrossRef CAS PubMed;
(h) S.-H. Cai, L. Ye, D.-X. Wang, Y.-Q. Wang, L.-J. Lai, C. Zhu, C. Feng and T.-P. Loh, Chem. Commun., 2017, 53, 8731 RSC;
(i) X. Lu, Y. Wang, B. Zhang, J.-J. Pi, X.-X. Wang, T.-J. Gong, B. Xiao and Y. Fu, J. Am. Chem. Soc., 2017, 139, 12632 CrossRef CAS PubMed;
(j) L. Yu, M.-L. Tang, C.-M. Si, Z. Meng, Y. Liang, J. Han and X. Sun, Org. Lett., 2018, 20, 4579 CrossRef CAS PubMed;
(k) L. Yang, W.-W. Ji, E. Lin, J.-L. Li, W.-X. Fan, Q. Li and H. Wang, Org. Lett., 2018, 20, 1924 CrossRef CAS PubMed.
-
(a) S. Guo, P. Yang and J. Zhou, Chem. Commun., 2015, 51, 12115 RSC;
(b) T. Ahrens, M. Teltewskoi, M. Ahrens, T. Braun and R. Laubenstein, Dalton Trans., 2016, 45, 17495 RSC.
- For review, see:
(a) T. Ahrens, J. Kohlmann, M. Ahrens and T. Braun, Chem. Rev., 2015, 115, 931 CrossRef CAS PubMed ; for Ni-catalyzed β-F elimination, see:;
(b) T. Ichitsuka, T. Fujita, T. Arita and J. Ichikawa, Angew. Chem., Int. Ed., 2014, 53, 7564 CrossRef CAS PubMed;
(c) Y. Watabe, K. Kanazawa, T. Fujita and J. Ichikawa, Synthesis, 2017, 49, 3569 CrossRef CAS;
(d) T. Ichitsuka, T. Fujita and J. Ichikawa, ACS Catal., 2015, 5, 5947 CrossRef CAS;
(e) M. Ohashi, Y. Ueda and S. Ogoshi, Angew. Chem., Int. Ed., 2017, 56, 2435 CrossRef CAS PubMed;
(f) Y. Lan, F. Yang and C. Wang, ACS Catal., 2018, 8, 9245 CrossRef CAS.
-
(a)
J.-P. Bégué and D. Bonnet-Delpon, Bioorganic and Medicinal Chemistry of Fluorine, Wiley, Hoboken, 2008 CrossRef;
(b) G. Landelle, M. Bergeron, M.-O. Turcotte-Savard and J.-F. Paquin, Chem. Soc. Rev., 2011, 40, 2867 RSC;
(c) B. Malo-Forest, G. Landelle, J.-A. Roy, J. Lacroix, R. C. Gaudreault and J.-F. Paquin, Bioorg. Med. Chem. Lett., 2013, 23, 1712 CrossRef CAS PubMed;
(d) S. Osada, S. Sano, M. Ueyama, Y. Chuman, H. Kodama and K. Sakaguchi, Bioorg. Med. Chem., 2010, 18, 605 CrossRef CAS PubMed.
- The Z-configuration of 3a was determined by comparing the NMR data with structurally analogous compounds reported in the literature. F. Larnaud, J. Malassis, E. Pfund, B. Linclau and T. Lequeux, Org. Lett., 2013, 15, 2450 CrossRef CAS PubMed.
-
(a) T. León, A. Correa and R. Martin, J. Am. Chem. Soc., 2013, 135, 1221 CrossRef PubMed;
(b) F. B. Sayyed and S. Sakaki, Chem. Commun., 2014, 50, 13026 RSC.
-
(a) D. L. Orsi and R. A. Altman, Chem. Commun., 2017, 53, 7168 RSC;
(b) C. M. R. Volla, A. Das, I. Atodiresei and M. Rueping, Chem. Commun., 2014, 50, 7889 RSC;
(c) D. Crich and O. Vinogradova, J. Am. Chem. Soc., 2007, 129, 11756 CrossRef CAS PubMed;
(d) J.-S. Yu, Y.-L. Liu, J. Tang, X. Wang and J. Zhou, Angew. Chem., Int. Ed., 2014, 53, 9512 CrossRef CAS PubMed.
-
(a) J. Liu, Q. Ren, X. Zhang and H. Gong, Angew. Chem., Int. Ed., 2016, 55, 15544 CrossRef CAS PubMed;
(b) J. L. Hofstra, A. H. Cherney, C. M. Ordner and S. E. Reisman, J. Am. Chem. Soc., 2018, 140, 139 CrossRef CAS PubMed;
(c) A. H. Cherney and S. E. Reisman, J. Am. Chem. Soc., 2014, 136, 14365 CrossRef CAS PubMed.
- Considering the reaction exhibits complete Z-selectivity, Ni-catalyzed β-F elimination through intermediate VII is most likely the pathway for the monofluoroalkene formation as suggested by ref. 8d, f and i..
-
(a) G. B. Deacon, C. M. Forsyth, P. C. Junk and J. Wang, Chem.–Eur. J., 2009, 15, 3082 CrossRef CAS PubMed;
(b) A. M. Träff, M. Janjetovic, L. Ta and G. Hilmersson, Angew. Chem., Int. Ed., 2013, 52, 12073 CrossRef PubMed.
- M. Tobisu, T. Xu, T. Shimasaki and N. Chatani, J. Am. Chem. Soc., 2011, 133, 19505 CrossRef CAS PubMed.
- X. Wang, M. Nakajima, E. Serrano and R. Martin, J. Am. Chem. Soc., 2016, 138, 15531 CrossRef CAS PubMed.
Footnotes |
† Electronic supplementary information (ESI) available. See DOI: 10.1039/c8sc04162h |
‡ These two authors contributed equally. |
|
This journal is © The Royal Society of Chemistry 2019 |
Click here to see how this site uses Cookies. View our privacy policy here.