DOI:
10.1039/C8SC05685D
(Edge Article)
Chem. Sci., 2019,
10, 2693-2699
Catalytic β C–H amination via an imidate radical relay†
Received
20th December 2018
, Accepted 9th January 2019
First published on 17th January 2019
Abstract
The first catalytic strategy to harness imidate radicals for C–H functionalization has been developed. This iodine-catalyzed approach enables β C–H amination of alcohols by an imidate-mediated radical relay. In contrast to our first-generation, (super)stoichiometric protocol, this catalytic method enables faster and more efficient reactivity. Furthermore, lower oxidant concentration affords broader functional group tolerance, including alkenes, alkynes, alcohols, carbonyls, and heteroarenes. Mechanistic experiments interrogating the electronic nature of the key 1,5 H-atom transfer event are included, as well as probes for chemo-, regio-, and stereo-selectivity.
Introduction
At the frontier of organic synthesis, the selective replacement of an unbiased C–H bond with a more valuable chemical motif remains a vital challenge.1 Specifically, incorporation of a nitrogen atom by C–H amination is an especially important goal in medicinal chemistry.2,3 Among recent advances toward directed sp3 C–H functionalization of abundant alcohol derivatives,4 there remain few methods to synthesize β amino alcohols (a privileged motif in medicine)5 by C–H amination.6 To complement state-of-the-art, metal-catalyzed nitrenoid and C–H insertion pathways for remote C–H amination,7 we sought to employ a radical-based approach that entails δ selective, hydrogen atom transfer (HAT).8,9 Despite recent advances in δ C–H amination via HAT,10 there remain few catalytic examples of this transformation.11 Having recently disclosed the first method for directed β C–H amination of alcohols by a complementary imidate radical relay,12,13 we sought to develop an improved, catalytic strategy (Fig. 1).
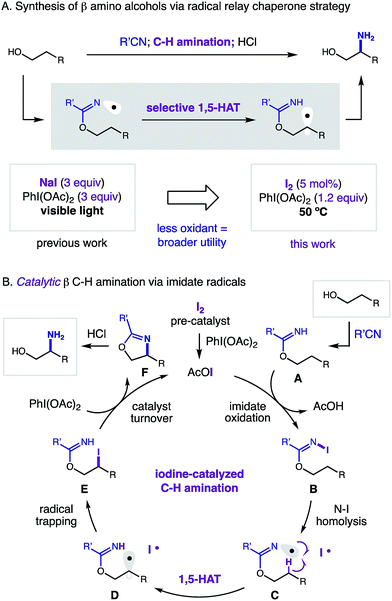 |
| Fig. 1 Radical relay strategy for β C–H amination of alcohols: (a) catalytic vs stoichiometric. (b) Iodine-catalyzed mechanism. | |
In our radical relay chaperone strategy, alcohols are readily converted to imidates by addition to nitriles (Fig. 1a). Upon combination with stoichiometric oxidant (NaI, PhI(OAc)2; 3 equiv. each), a transient sp2 N-centered radical14,15 is generated that undergoes selective 1,5-HAT to afford a C-centered radical – β to the imidate. Subsequent radical trapping and acidic hydrolysis yields β amino alcohols in a rapid, selective, and efficient fashion. With the hopes of expanding the synthetic utility of this new strategy, we proposed development of a catalytic variant (Fig. 1b). To complement our first-generation, photo-mediated method, we hypothesized the key, radical-generating iodine atom, which is not incorporated in the product, could be continually recycled and ultimately employed in a catalytic fashion.
In an alternate, thermally initiated sequence, we envisioned a substoichiometric quantity of I2 may undergo ligand substitution with PhI(OAc)2 (1 equiv. only) to generate AcOI. In the presence of an alcohol-derived imidate (A), selective formation of a weak N–I bond (B)16,17 would enable thermal homolysis to an N-centered radical (C). This transient species (typically accessed by photolysis)18 should undergo regio-selective 1,5-HAT to yield β radical (D). Rapid radical recombination (or chain-propagation with AcOI or N–I) would yield β alkyl iodide (E). In the presence of PhI(OAc)2 as a terminal oxidant, we proposed oxazoline (F) formation may accompany regeneration of the AcOI catalyst by one of two mechanisms: (1) iodide displacement by the imidate, and re-oxidation of I− to I+,19 or (2) alkyl hypervalent iodane formation, and amination via an I(III)/I(I) pathway.20 Importantly, we proposed thermal initiation of this catalytic cycle under low concentrations of I2 (or AcOI) may improve reaction efficiency and chemoselectivity by precluding byproduct-forming pathways associated with photolysis of these promiscuous oxidants.21
Results and discussion
In accord with our design, we were pleased to find the catalytic β C–H amination of imidate 1 by HAT is indeed possible with 5 mol% I2 and 1.2 equiv. PhI(OAc)2, affording 2 in 95% yield (Table 1). Crucially, this thermal protocol requires polar, aprotic solvents (e.g. DMF, MeCN), whereas other solvents (e.g. CH2Cl2, PhMe) afford inferior yields (entries 1–4). Although rigorous degassing is not essential, an N2 atmosphere was found to be superior to an aerobic one (entry 5). Although alkali iodide salts (e.g. NaI, CsI) are competent sources of iodine for this reaction, they are less efficient than more soluble I2 reagent (entries 6 and 7). Finally, photolysis (entry 8) or non-photolytic initiation at room temperature (entry 9) afford reactivity, albeit with less efficiency than standard thermal initiation at 50 °C.
Table 1 Development of a catalytic C–H amination of imidates
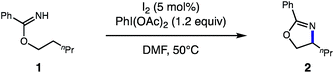
|
Entry |
Changes from standard conditions |
Yield (%) |
1 |
None |
95 |
2 |
CH2Cl2 instead of DMF |
31 |
3 |
PhMe instead of DMF |
33 |
4 |
MeCN instead of DMF |
94 |
5 |
Air atmosphere instead of N2 |
61 |
6 |
NaI instead of I2 |
67 |
7 |
CsI instead of I2 |
76 |
8 |
2 × 23 W CFL |
50 |
9 |
Dark, room temperature |
40 |
Interestingly, we noted this catalytic reaction is completed at a significantly faster rate than the first-generation, photo-initiated conditions. As shown in Fig. 2, our previous conditions, which are super-stoichiometric in NaI and PhI(OAc)2, required several hours for reaction completion (purple line). Conversely, 80% yield is observed in 30 minutes with 5–10% I2 (red and blue lines) or even in as little as 10 minutes with 20% I2 (green line). Although a mere 1% I2 provides full conversion in 6 hours, we found these longer reaction times to be less practical than the 1–2 hours needed for 5% catalyst loading. Given that less soluble sources of iodide (e.g. NaI, CsI) do not afford product as rapidly or efficiently (likely due to slower, incomplete generation of I2), we presume greater solubility of I2 affords a higher initial concentration of the active oxidant, AcOI. Taken together, these data suggest the faster rates shown in Fig. 2 are consistent with a higher initial concentration of reactive AcOI, as proposed in the mechanism shown in Fig. 1. Moreover, less terminal oxidant, and thermal (vs. photolytic) initiation, may be responsible for ensuring AcOI-based, two-electron reactivity is more selective for the desired reaction pathway.
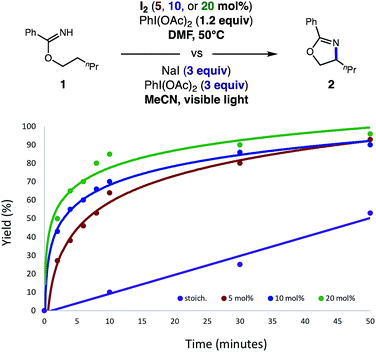 |
| Fig. 2 Comparison of the catalytic C–H amination of imidates with the previous stoichiometric version. | |
Synthetic scope
In order to explore the synthetic utility of our new thermally initiated, I2-catalyzed protocol, we subjected a series of imidates to these β C–H amination conditions (5% I2, 1.2 equiv. PhI(OAc)2, DMF, 50 °C). Upon reaction completion, acidic hydrolysis of the resulting oxazoline with aq. HCl yielded the respective β amino alcohol. As shown in Fig. 3, a wide range of imidates undergo the radical relay mechanism via these catalytic conditions. For trichloroacetimidates (derived from combination of alcohols and Cl3C–CN), a range of electronically diverse 2-phenylethanol derivatives could be selectively aminated at the β position. These benzylic C–H aminations (3–10) are amenable to both electronically rich and deficient substituents (OMe, Me, F, CF3) as well as ortho, meta, and para substitution. Additionally, medicinally relevant heteroarenes (thiophene, pyridine, 11–12) are tolerated, as well as the tertiary C–H of an ibuprofen analog (13). Secondary alcohols are efficiently aminated with excellent diastereoselectivity (up to >20
:
1 d.r.; 14–15). Finally, the allylic C–H of a cholesterol analog is also efficiently and stereo-selectively aminated (16). To promote the β amination of stronger, aliphatic C–H bonds, we employed benzimidates (derived from combination of alcohols with Ph-CN or with Ph(CN)OCH2CF3). This catalytic protocol is also suitable for the regioselective amination of primary, secondary, and tertiary C–H bonds (17–21). Similarly, secondary alcohols are tolerated, although greater diastereoselectivity is observed for cyclic versus acyclic cases (>20
:
1 d.r. vs. 1
:
1 d.r.).
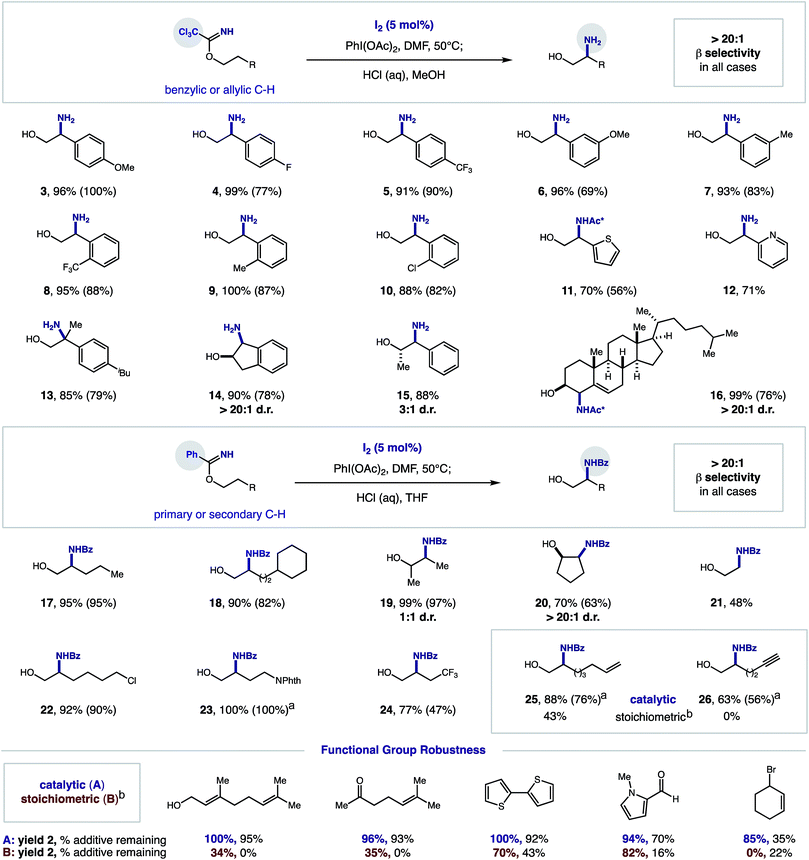 |
| Fig. 3 Synthetic utility of iodine-catalyzed β C–H amination of imidates. Conditions: 0.4 mmol imidate, I2 (5 mol%), PhI(OAc)2 (1.2 equiv.), DMF (0.2 M), 50 °C. 1H NMR yield of oxazoline determined vs. standard. Hydrolysis with HCl (2 M) affords amino alcohol (isolated yield, in parenthesis). aIsolated yield of oxazoline. bStoichiometric NaI (ref. 12). Ac* refers to trichloroacetamide. Functional group robustness: C–H amination yield, % additive remaining. | |
As a testament to the synthetic utility provided by these mild, catalytic conditions, several functional groups that were not previously tolerated in our stoichiometric protocol can now be aminated (22–26). Most interestingly, the reactive π-systems of alkenes and alkynes, which are prone to deleterious reaction with large amounts of oxidant, are now amenable as substrates. For example, whereas alkene 25 was previously accessible in only 43% yield under the stoichiometric, photochemical protocol, these catalytic, thermal conditions provide amination in 88% yield. Additionally, alkynes, which had previously not been tolerated (0%), are now suitable substrates for this β C–H amination (26, 63% yield).
To further probe this improved functional group tolerance, we conducted an additive robustness screen22 for both the stoichiometric and catalytic protocols – employing synthetically and medicinally relevant functionalities that appeared unlikely to withstand strong oxidative conditions. As shown in Fig. 3, this catalytic method is superior for the β C–H amination of imidate 1 to 2 in the presence of various functional groups, including alkenes, alcohols, ketones, aldehydes, thiophenes, pyrroles, and halides (catalytic: 85–100%; stoichiometric: 0–82% yield). Illustrating the mildness of the new catalytic protocol, these additives are recovered in up to 95% yield, whereas they are frequently decomposed in the highly oxidative environment of the stoichiometric conditions (0–43%) (see ESI† for complete table of functional groups tolerated, including those that are tolerated in both conditions).
Mechanistic investigations
To gain a deeper understanding of this reaction mechanism, we conducted a series of competitive rate studies interrogating various stereoelectronic effects. First, we probed the regioselectivity of the imidate radical-mediated amination in the presence of weaker C–H bonds. Although our reaction design is based on the entropic and enthalpic favorability of 1,5-HAT,8a there are notable examples of 1,6-HAT mediated pathways that are governed by substrate geometry23 or thermodynamics.24 To test the influence of the latter, the β selectivity of this C–H amination was investigated for alcohols bearing a weaker γ C–H bond (Fig. 4). In each case, β selectivity (via 1,5-HAT) was observed in preference to γ selectivity (via 1,6-HAT). When the γ C–H bond is significantly weaker (benzylic: 90 vs. secondary: 98 kcal mol−1),25 the β amine 27 is still preferentially formed (2
:
1 β
:
γ selectivity). However, when γ C–H bond is only marginally weaker (3°: 96 vs. 2°: 98 kcal mol−1),25 the β amine 28 is obtained exclusively (>20
:
1 β
:
γ selectivity).
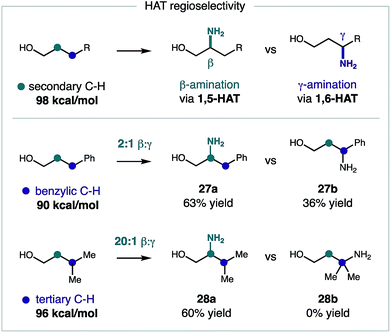 |
| Fig. 4 Regioselectivity probes of β selectivity via 1,5-HAT. | |
Next, we investigated the observed diastereoselectivity of the β C–H amination by employing cis and trans isomers of 2-phenyl-cyclohexanol 29 as stereochemical probes in the formation of β amino alcohol 30 (Fig. 5). Whereas the trichloroacetimidate of cis-29 does not afford C–H amination (likely because the imidate radical is conformationally constrained to the opposite side of the ring), trans-29 efficiently undergoes HAT (since the imidate radical and β C–H are syn to one another). Interestingly, these catalytic conditions afford greater diastereoselectivity (5
:
1 d.r.) than the stoichiometric protocol (2
:
1 d.r.). Moreover, β benzyl iodide intermediate 31 was observed for the first time, only in the catalytic case. Taken together, these results suggest divergent mechanisms are operative in the radical trapping steps of these two protocols. A possible explanation is that the higher oxidant concentration of the (super)stoichiometric method more rapidly oxidizes the benzyl radical to a cation, which is unselectively cyclized to afford the thermodynamically favored cis product in only a 2
:
1 excess. On the other hand, a stepwise iodine trapping and subsequent cyclization mechanism under the low oxidant concentration of the catalytic conditions allow for greater 5
:
1 diastereoselectivity. This likely occurs via slower conversion of the observed alkyl iodide intermediate 31, which enables greater, overall stereocontrol. Oxidation of benzyl iodide 31 to its hypervalent iodane nucleofuge may also afford cyclization – with either retention or inversion.26
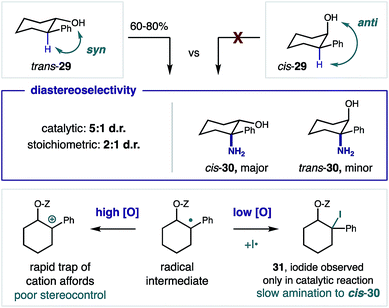 |
| Fig. 5 Diastereoselectivity via divergent trapping mechanisms. | |
Finally, we examined the nature of the hydrogen atom transfer mechanism via a Hammett study (Fig. 6). By varying substituents of 2-arylethanol imidates, we determined a linear free-energy relationship exists between initial reaction rates and the electronics of the para-substituents. As shown in Fig. 6, we observed reaction acceleration with p-OMe and p-Me groups, whereas p-CF3 and p-NO2 substituents decrease product formation relative to the parent 2-Ph-ethanol. The resulting negative slope (ρ) of the Hammett equation is consistent with other HAT-mediated C–H functionalizations.27 In this case, we propose intramolecular HAT (which we have shown to be rate-limiting, with primary KIE values up to 8)12 is enabled by an electrophilic N-centered radical, gaining electron density in the transition state, as an N–H σ bond is formed. At the same time, the carbon atom loses electron density in the transition state as the ensuing C-centered radical is formed. Thus, electron-releasing groups at the para-position stabilize this transition state by electron donation, while electron-withdrawing groups have the opposite effect. The resultant stabilization by donating groups thus reasonably explain the observed reaction rate acceleration.
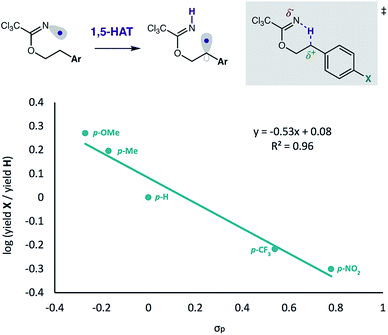 |
| Fig. 6 Hammett plot correlates to a cation-like transition state. | |
Conclusions
In summary, we have developed the first catalytic variant of our radical chaperone strategy for converting alcohols into β amino alcohols via HAT. This conversion of ubiquitous motifs into privileged pharmacophores is a synthetically valuable method enabled by a radical relay cascade. Through a new, I2-catalyzed protocol, this β C–H amination sequence now has significantly broadened synthetic utility. We expect additional mechanistic insights provided herein (on reaction rates, as well as chemo-, regio- and stereo-selectivity) will enable further applications of the imidate-mediated HAT in regioselective C–H functionalizations.
Conflicts of interest
There are no conflicts to declare.
Acknowledgements
We thank the National Institutes of Health (NIH R35 GM119812) and National Science Foundation (NSF CAREER 1654656) for financial support. LMS is supported by the NSF GRFP Fellowship.
Notes and references
- H. Yi, G. Zhang, H. Wang, Z. Huang, J. Wang, A. K. Singh and A. Lei, Chem. Rev., 2017, 117, 9016–9085 CrossRef CAS PubMed.
- C–H amination reviews:
(a) H. M. L. Davies and J. R. Manning, Nature, 2008, 451, 417–424 CrossRef CAS PubMed;
(b) J. L. Roizen, M. E. Harvey and J. Du Bois, Acc. Chem. Res., 2012, 45, 911–922 CrossRef CAS PubMed;
(c) J. L. Jeffrey and R. Sarpong, Chem. Sci., 2013, 4, 4092–4106 RSC;
(d) Y. Park, Y. Kim and S. Chang, Chem. Rev., 2017, 117, 9247–9301 CrossRef CAS PubMed.
-
(a) T. Cernak, K. D. Dykstra, S. Tyagarajan, P. Vachal and S. W. Krska, Chem. Soc. Rev., 2016, 45, 546–576 RSC;
(b) D. C. Blakemore, L. Castro, I. Churcher, D. C. Rees, A. W. Thomas, D. M. Wilson and A. Wood, Nat. Chem., 2018, 10, 383–394 CrossRef CAS PubMed.
-
(a) R. Breslow and M. A. Winnik, J. Am. Chem. Soc., 1969, 91, 3083–3084 CrossRef CAS;
(b) D. P. Curran, D. Kim, H. T. Liu and W. Shen, J. Am. Chem. Soc., 1988, 110, 5900–5902 CrossRef CAS;
(c) K. Chen, J. M. Richter and P. S. Baran, J. Am. Chem. Soc., 2008, 130, 7247–7249 CrossRef CAS PubMed;
(d) E. M. Simmons and J. F. Hartwig, Nature, 2012, 483, 70–73 CrossRef CAS PubMed;
(e) Z. Ren, F. Mo and G. Dong, J. Am. Chem. Soc., 2012, 134, 16991–16994 CrossRef CAS PubMed;
(f) M. Parasram, P. Chuentragool, D. Sarkar and V. Gevorgyan, J. Am. Chem. Soc., 2016, 138, 6340–6343 CrossRef CAS PubMed;
(g) J. Ozawa, M. Tashiro, J. Ni, K. Oisaki and M. Kanai, Chem. Sci., 2016, 7, 1904–1909 RSC;
(h) M. Parasram, P. Chuentragool, Y. Wang, Y. Shi and V. Gevorgyan, J. Am. Chem. Soc., 2017, 139, 14857–14860 CrossRef CAS PubMed;
(i) Y. Xu and G. Dong, Chem. Sci., 2018, 9, 1424–1432 RSC;
(j) S. Sathyamoorthi, S. Banerjee, J. Du Bois, N. Z. Burns and R. N. Zare, Chem. Sci., 2018, 9, 100–104 RSC;
(k) E. Azek, M. Khalifa, J. Bartholoméüs, M. Ernzerhof and H. Lebel, Chem. Sci., 2019 10.1039/C8SC03153C.
-
(a) E. Vitaku, D. T. Smith and J. T. Njardarson, J. Med. Chem., 2014, 57, 10257–10274 CrossRef CAS PubMed;
(b) D. J. Ager, I. Prakash and D. R. Schaad, Chem. Rev., 1996, 96, 835–876 CrossRef CAS PubMed;
(c) S. C. Bergmeier, Tetrahedron, 2000, 56, 2561–2576 CrossRef CAS;
(d) O. K. Karjalainen and A. M. P. Koskinen, Org. Biomol. Chem., 2012, 10, 4311 RSC.
- C–H amination of carbamates:
(a) C. G. Espino, P. M. Wehn, J. Chow and J. Du Bois, J. Am. Chem. Soc., 2001, 123, 6935–6936 CrossRef CAS;
(b) Y. Cui and C. He, Angew. Chem., Int. Ed., 2004, 43, 4210–4212 CrossRef CAS PubMed;
(c) H. Lebel, K. Huard and S. Lectard, J. Am. Chem. Soc., 2005, 127, 14198–14199 CrossRef CAS PubMed;
(d) K. J. Fraunhoffer and M. C. White, J. Am. Chem. Soc., 2007, 129, 7274–7276 CrossRef CAS PubMed;
(e) S. M. Paradine, J. R. Griffin, J. Zhao, A. L. Petronico, S. M. Miller and M. Christina White, Nat. Chem., 2015, 7, 987–994 CrossRef CAS PubMed.
- Metal-catalyzed sp3 C–H aminations:
(a) R. Breslow and S. H. Gellman, J. Am. Chem. Soc., 1983, 105, 6728–6729 CrossRef CAS;
(b) B. Haffemayer, M. Gulias and M. J. Gaunt, Chem. Sci., 2011, 2, 312–315 RSC;
(c) E. T. Hennessy and T. A. Betley, Science, 2013, 340, 591–595 CrossRef CAS PubMed;
(d) G. He, S. Y. Zhang, W. A. Nack, Q. Li and G. Chen, Angew. Chem., Int. Ed., 2013, 52, 11124–11128 CrossRef CAS PubMed;
(e) H. Lu, H. Jiang, Y. Hu, L. Wojtas and X. P. Zhang, Chem. Sci., 2011, 2, 2361–2366 RSC;
(f) A. McNally, B. Haffemayer, B. S. L. Collins and M. J. Gaunt, Nature, 2014, 510, 129–133 CrossRef CAS PubMed;
(g) M. Yang, B. Su, Y. Wang, K. Chen, X. Jiang, Y.-F. Zhang, X.-S. Zhang, G. Chen, Y. Cheng, Z. Cao, Q.-Y. Guo, L. Wang and Z.-J. Shi, Nat. Commun., 2014, 5, 4707 CrossRef CAS PubMed;
(h) E. T. Hennessy, R. Y. Liu, D. A. Iovan, R. A. Duncan and T. A. Betley, Chem. Sci., 2014, 5, 1526–1532 RSC;
(i) Z. Wang, J. Ni, Y. Kuninobu and M. Kanai, Angew. Chem., Int. Ed., 2014, 53, 3496–3499 CrossRef CAS PubMed;
(j) O. Villanueva, N. M. Weldy, S. B. Blakey and C. E. MacBeth, Chem. Sci., 2015, 6, 6672–6675 RSC;
(k) X. Wu, K. Yang, Y. Zhao, H. Sun, G. Li and H. Ge, Nat. Commun., 2015, 6, 6462 CrossRef CAS PubMed;
(l) H. Lu, K. Lang, H. Jiang, L. Wojtas and X. P. Zhang, Chem. Sci., 2016, 7, 6934–6939 RSC;
(m) M. Shang, Q. Shao, S. Z. Sun, Y. Q. Chen, H. Xu, H. X. Dai and J. Q. Yu, Chem. Sci., 2017, 8, 1469–1473 RSC;
(n) S. A. Cook, J. A. Bogart, N. Levi, A. C. Weitz, C. Moore, A. L. Rheingold, J. W. Ziller, M. P. Hendrich and A. S. Borovik, Chem. Sci., 2018, 9, 6540–6547 RSC.
- HAT reviews:
(a) L. M. Stateman, K. M. Nakafuku and D. A. Nagib, Synthesis, 2018, 50, 1569–1586 CrossRef CAS PubMed;
(b) W. Li, W. Xu, J. Xie, S. Yu and C. Zhu, Chem. Soc. Rev., 2018, 47, 654–667 RSC;
(c) S. Chiba and H. Chen, Org. Biomol. Chem., 2014, 12, 4051–4060 RSC.
- Pioneering examples:
(a) C. Betancor, J. I. Concepción, R. Hernández, J. A. Salazar and E. Suárez, J. Org. Chem., 1983, 48, 4430–4432 CrossRef CAS;
(b) C. G. Francisco, A. J. Herrera and E. Suárez, J. Org. Chem., 2003, 68, 1012–1017 CrossRef CAS PubMed.
- Recent stoichiometric remote C–H aminations via HAT:
(a) T. Liu, T.-S. Mei and J.-Q. Yu, J. Am. Chem. Soc., 2015, 137, 5871–5874 CrossRef CAS PubMed;
(b) E. A. Wappes, S. C. Fosu, T. C. Chopko and D. A. Nagib, Angew. Chem., Int. Ed., 2016, 55, 9974–9978 CrossRef CAS PubMed;
(c) N. R. Paz, D. Rodríguez-Sosa, H. Valdés, R. Marticorena, D. Melián, M. B. Copano, C. C. González and A. J. Herrera, Org. Lett., 2015, 17, 2370–2373 CrossRef CAS PubMed;
(d) C. Q. O'Broin, P. Fernández, C. Martínez and K. Muñiz, Org. Lett., 2016, 18, 436–439 CrossRef PubMed;
(e) D. Zhang, H. Wang, H. Cheng, J. G. Hernández and C. Bolm, Adv. Synth. Catal., 2017, 359, 4274–4277 CrossRef CAS;
(f) J. Long, X. Cao, L. Zhu, R. Qiu, C.-T. Au, S.-F. Yin, T. Iwasaki and N. Kambe, Org. Lett., 2017, 19, 2793–2796 CrossRef CAS PubMed;
(g) S. C. Cosgrove, J. M. C. Plane and S. P. Marsden, Chem. Sci., 2018, 9, 6647–6652 RSC;
(h) X. Hu, G. Zhang, F. Bu, L. Nie and A. Lei, ACS Catal., 2018, 8, 9370–9375 CrossRef CAS;
(i) D. Chang, R. Zhao, C. Wei, Y. Yao, Y. Liu and L. Shi, J. Org. Chem., 2018, 83, 3305–3315 CrossRef CAS PubMed.
- Recent catalytic remote C–H aminations via HAT:
(a) Q. Qin and S. Yu, Org. Lett., 2015, 17, 1894–1897 CrossRef CAS PubMed;
(b) C. Zhu, Y. Liang, X. Hong, H. Sun, W.-Y. Sun, K. N. Houk and Z. Shi, J. Am. Chem. Soc., 2015, 137, 7564–7567 CrossRef CAS PubMed;
(c) C. Martínez and K. Muñiz, Angew. Chem., Int. Ed., 2015, 54, 8287–8291 CrossRef PubMed;
(d) P. Becker, T. Duhamel, C. J. Stein, M. Reiher and K. Muñiz, Angew. Chem., Int. Ed., 2017, 56, 8004–8008 CrossRef CAS PubMed;
(e) D. Meng, Y. Tang, J. Wei, X. Shi and M. Yang, Chem. Commun., 2017, 53, 5744–5747 RSC;
(f) A. Hu, J.-J. Guo, H. Pan, H. Tang, Z. Gao and Z. Zuo, J. Am. Chem. Soc., 2018, 140, 1612–1616 CrossRef CAS PubMed;
(g) T. Duhamel, C. J. Stein, C. Martínez, M. Reiher and K. Muñiz, ACS Catal., 2018, 8, 3918–3925 CrossRef CAS.
- E. A. Wappes, K. M. Nakafuku and D. A. Nagib, J. Am. Chem. Soc., 2017, 139, 10204–10207 CrossRef CAS PubMed.
- Recent imidate radical relays:
(a) X. Q. Mou, X. Y. Chen, G. Chen and G. He, Chem. Commun., 2018, 54, 515–518 RSC;
(b) E. A. Wappes, A. Vanitcha and D. A. Nagib, Chem. Sci., 2018, 9, 4500–4504 RSC;
(c) Y. Kumar, Y. Jaiswal and A. Kumar, Org. Lett., 2018, 20, 4964–4969 CrossRef CAS PubMed;
(d) K. M. Nakafuku, S. C. Fosu and D. A. Nagib, J. Am. Chem. Soc., 2018, 140, 11202–11205 CrossRef CAS PubMed.
- N-centered radical reviews:
(a) S. Z. Zard, Chem. Soc. Rev., 2008, 37, 1603–1618 RSC;
(b) T. Xiong and Q. Zhang, Chem. Soc. Rev., 2016, 45, 3069–3087 RSC;
(c) Y. Zhao and W. Xia, Chem. Soc. Rev., 2018, 47, 2591–2608 RSC.
- Iminyl radical reactivity:
(a) A. R. Forrester, M. Gill and R. H. Thomson, J. Chem. Soc., Perkin Trans. 1, 1979, 621–631 RSC;
(b) J. Boivin, E. Fouquet and S. Z. Zard, J. Am. Chem. Soc., 1991, 113, 1055–1057 CrossRef CAS;
(c) J. Davies, S. G. Booth, S. Essafi, R. A. W. Dryfe and D. Leonori, Angew. Chem., Int. Ed., 2015, 54, 14017–14021 CrossRef CAS PubMed;
(d) W. Shu and C. Nevado, Angew. Chem., Int. Ed., 2017, 56, 1881–1884 CrossRef CAS PubMed;
(e) J. Davies, N. S. Sheikh and D. Leonori, Angew. Chem., Int. Ed., 2017, 56, 13361–13365 CrossRef CAS PubMed;
(f) H. Jiang and A. Studer, Angew. Chem., Int. Ed., 2017, 56, 12273–12276 CrossRef CAS PubMed;
(g) E. M. Dauncey, S. P. Morcillo, J. J. Douglas, N. S. Sheikh and D. Leonori, Angew. Chem., Int. Ed., 2018, 57, 744–748 CrossRef CAS PubMed;
(h) H. Jiang and A. Studer, Angew. Chem., Int. Ed., 2018, 57, 1692–1696 CrossRef CAS PubMed.
- Since AcOI is electrophilic at I, combination with an imidate likely forms N–I vs. N–OAc; E. M. Chen, R. M. Keefer and L. J. Andrews, J. Am. Chem. Soc., 1967, 89, 428–430 CrossRef CAS.
- An N–OAc oxime imidate is also an unlikely intermediate since it is stable to thermal decomposition (up to 110 °C), and it does not afford product under these reaction conditions. See ESI† for details.
- S. A. Glover, G. P. Hammond, D. G. Harman, J. G. Mills and C. A. Rowbottom, Aust. J. Chem., 1993, 46, 1213–1228 CrossRef CAS . See also ref. 12 and 13b–d.
-
(a) M. Uyanik, H. Okamoto, T. Yasui and K. Ishihara, Science, 2010, 328, 1376–1379 CrossRef CAS PubMed;
(b) M. Uyanik, H. Hayashi and K. Ishihara, Science, 2014, 345, 291–294 CrossRef CAS PubMed . See also ref. 11d.
- A. Yoshimura and V. V. Zhdankin, Chem. Rev., 2016, 116, 3328–3435 CrossRef CAS PubMed . See also ref. 11c.
- J. L. Courtneidge, J. Lusztyk and D. Pagé, Tetrahedron Lett., 1994, 35, 1003–1006 CrossRef CAS.
-
(a) K. D. Collins and F. Glorius, Nat. Chem., 2013, 5, 597–601 CrossRef CAS PubMed;
(b) K. D. Collins and F. Glorius, Acc. Chem. Res., 2015, 48, 619–627 CrossRef CAS PubMed;
(c) T. Gensch, M. Teders and F. Glorius, J. Org. Chem., 2017, 82, 9154–9159 CrossRef CAS PubMed.
- M. A. Short, J. M. Blackburn and J. L. Roizen, Angew. Chem., Int. Ed., 2018, 57, 296–299 CrossRef CAS PubMed.
-
(a) M. Koag and S. Lee, Org. Lett., 2011, 13, 4766–4769 CrossRef CAS PubMed;
(b) H. Zhang and K. Muñiz, ACS Catal., 2017, 7, 4122–4125 CrossRef CAS.
-
Y. R. Luo, Comprehensive Handbook of Chemical Bond Energies, Taylor & Francis, Boca Raton, FL, 2010 Search PubMed.
- R. C. Cambie, D. Chambers, B. G. Lindsay, P. S. Rutledge and P. D. Woodgate, J. Chem. Soc., Perkin Trans. 1, 1980, 822–827 RSC.
-
(a) A. Coniglio, C. Galli, P. Gentili and R. Vadalà, Org. Biomol. Chem., 2009, 7, 155–160 RSC;
(b) P. C. Nam, M. T. Nguyen and A. K. Chandra, J. Phys. Chem. A, 2005, 109, 10342–10347 CrossRef CAS PubMed.
Footnote |
† Electronic supplementary information (ESI) available. See DOI: 10.1039/c8sc05685d |
|
This journal is © The Royal Society of Chemistry 2019 |
Click here to see how this site uses Cookies. View our privacy policy here.