DOI:
10.1039/C9SC00205G
(Edge Article)
Chem. Sci., 2019,
10, 3937-3942
Switching transcription with bacterial RNA polymerase through photocaging, photorelease and phosphorylation reactions in the major groove of DNA†
Received
14th January 2019
, Accepted 1st March 2019
First published on 4th March 2019
Abstract
We report proof of principle biomimetic switching of transcription in vitro through non-natural chemical reactions in the major groove of DNA templates. Photocaged DNA templates containing nitrobenzyl-protected 5-hydroxymethyluracil or – cytosine permitted no transcription with E. coli RNA polymerase (OFF state). Their irradiation with 400 nm light resulted in DNA templates containing hydroxymethylpyrimidines, which switched transcription ON with a higher yield (250–350%) compared to non-modified DNA. Phosphorylation of templates containing 5-hydroxymethyluracil (but not 5-hydroxymethylcytosine) then turned transcription OFF again. It is the first step towards artificial bioorthogonal chemical epigenetics.
Epigenetic modifications of DNA by 5-methylcytosine and its oxidized congeners, i.e. 5-hydroxymethyl- or 5-formylcytosine, regulate gene expression1–5 through enhancing or inhibition of binding of transcription factors (TFs) and RNA polymerases (RNAP) to genomic DNA6–8 or through modulation of chromatin properties.9,10 Natural DNA methylation and demethylation occurs during the differentiation of cells to switch on and off certain genes.1–5,11–15 Despite great progress in recent years, the biological roles of the different epigenetic modifications are not yet fully understood.1–17 On the other hand, there is a challenging opportunity to introduce some non-canonical modifications to DNA to explore their possible use in regulation of gene expression.18–23 We have reported a study of transcription of DNA templates bearing different non-natural modifications in the major groove by bacterial RNAPs and found that bulkier modifications inhibited transcription whereas some small modifications were tolerated and the modified DNA templates were still transcribed into RNA.24 We also found that DNA templates containing 5-hydroxymethyluracil (Uhm), a rare natural base whose biological role is yet unknown,25–28 can enhance (up to 3.5 times) transcription depending on the promoter.29 We envisaged that some bioorthogonal chemical reactions in the major groove of DNA could be used to manipulate the bulkiness of the modification and we recently published the first paper on turning OFF transcription through a click reaction of 5-ethynyluracil in the major groove.30 Understanding of how nucleic acids can be modified and subsequently interact with RNAP is still in its infancy. Here we report a proof of principle one-way switch ON and OFF through photocaging, photochemical deprotection, and phosphorylation of 5-hydroxymethyluracil or – cytosine (Chm).
Photocaging of nucleic acids is frequently used for transient blocking of hybridization or other interactions which can be restored by photochemical release.31–35 We had recently reported the use of nitrobenzyl-37 or nitropiperonyl-caged38 5-hydroxymethyluracil or 5-hydroxymethylcytosine39 for transient protection of DNA against the cleavage by restriction endonucleases whereas more bulky nitrophenylethyl-caged nucleotides were previously used as reversible chain terminators.40,41 Therefore, the nitrobenzyl photocaging and release of Uhm and Chm was our first choice to set up a system that would allow to artificially switch transcription ON. In the opposite direction, we envisaged that phosphorylation of 5-hydroxymethyluracil by the natural 5-HMU DNA kinase (5-HMUDK)42–44 might be used to switch transcription OFF due to the increased bulkiness and negative charge of the phosphorylated Uhm.
Results and discussion
The 311-bp templates for transcription containing the Pveg promoter for transcription with E. coli RNAP were designed similarly as previously reported30,45 and were prepared by PCR using modified dUhmTP, dUNBTP,37dChmTP or dCNBTP39 instead of the corresponding natural pyrimidine nucleotide (Scheme 1). In all cases, full length amplicons were obtained efficiently and after isolation were used as templates for in vitro transcription experiments.
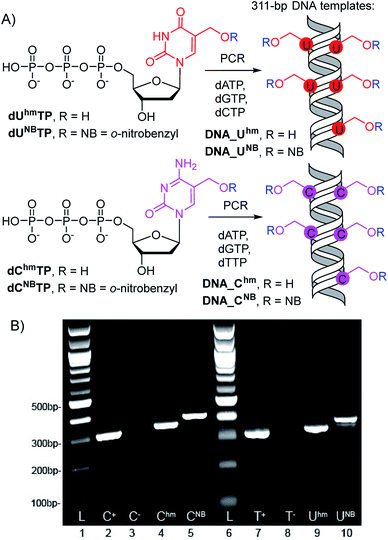 |
| Scheme 1 (A) PCR synthesis of the modified DNA templates, (B) agarose gel electrophoresis of the PCR products. | |
In accord with previous work,29 DNA containing Uhm or Chm displayed increased transcription compared to natural templates (ca. 350 or 220–250%, respectively), whereas templates containing the bulky photocaged bases UNB or CNB gave negligible transcription (<15%). We used 32P-labelled PCR products to accurately quantify the amounts of DNA templates because UV absorption or GelRed staining are not reliable for quantification of base-modified DNA.29 The photochemical deprotection of the photocaged templates (DNA_UNB or DNA_CNB) was performed using a 3 W photodiode with a maximum λ at 400 nm (in analogy to previous works38,39). In order to avoid DNA damage and absorption of light by nitrosobenzaldehyde, which is released as the byproduct,46 we used 1,4-dithiothreitol (DTT) and sodium azide as additives47 (see Fig. S6 in ESI† for the study of the influence of additives). At first, we carried out a simple kinetic study of irradiation of DNA_UNB or DNA_CNB for different reaction times, checked the completion of the deprotection of DNA_UNB by cleavage with REs38,39 (see Fig. S9 in ESI†) and then used them as templates for transcription. In control experiments, we irradiated the non-modified, as well as hydroxymethylated DNA_Uhm or DNA_Chm templates to confirm that the irradiation had no effect on the non-photocaged DNA templates and their transcription. The kinetic study (Fig. 1, S10 and S12 in ESI†) showed that the irradiation of DNA_UNB (for 20 min) or DNA_CNB (for 10 min) released DNA templates and resulted in approximately the same level of transcription as the corresponding DNA_Uhm or DNA_Chm templates (ca. 350 or 230%, respectively) indicating that the deprotection had been completed. This is in accord with our previous studies of the kinetics of photorelease using cleavage with restriction endonucleases as indicator of the photodeprotection.38,39 On the other hand, longer irradiation (>30 min, Fig. S10 and S12 in ESI†) led to a gradual decrease in transcription probably due to DNA damage.
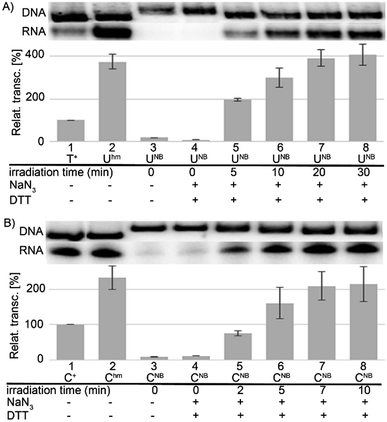 |
| Fig. 1 Kinetics of photochemical deprotection of NB-caged DNA templates [DNA_UNB (A), DNA_CNB (B)] monitored by transcription (lanes 3–8). Lanes 1 and 2 show control transcriptions from natural DNA and DNA_Uhm or DNA_Chm, respectively. Representative primary data (DNA templates and RNA transcripts) are shown. The graphs in this and following Figures are averages of at 2–3 independent experiments ±SD. The time of irradiation and usage of two additives (DTT and NaN3) are indicated below the graphs. | |
Next, we used the optimized conditions for a preparative experiment to turn transcription ON and OFF. Thus, the DNA_UNB template (which gives negligible transcription) was irradiated at 400 nm for 30 min and the resulting DNA_Uhm gave rise to the expected 350% transcription increase. Then, phosphorylation of the deprotected DNA_Uhm was performed in the presence of 5-HMUDK and ATP. Unlike in the photodeprotection of UNB to Uhm,38,39 we could not use cleavage by REs as accurate measure of the yield of phosphorylation (see Scheme S3 and Fig. S13 in ESI†). Therefore, we proceeded directly to the transcription study and found that the resulting phosphorylated template DNA_UhmP supported only a low level of transcription (37% compared to the non-modified DNA template, 10% compared to the starting DNA_Uhm), which indicates a significant (though not complete) switching OFF (Scheme 2, Fig. 2, see also Fig. S15A in ESI† for complete uncut gels).
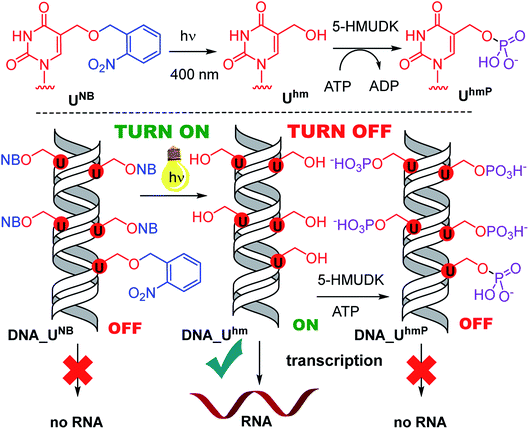 |
| Scheme 2 Switching transcription with photocaged DNA templates containing Uhm. | |
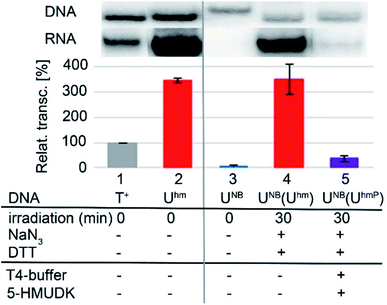 |
| Fig. 2
In vitro transcription from natural DNA (lane 1), DNA_Uhm (2) and DNA_UNB (3) templates. Lane 4 shows transcription from DNA_UNB template after 30 min irradiation with λ = 400 nm. Lane 5 shows transcription from DNA_UNB template after irradiation followed by treatment with 5-HMUDK and ATP. | |
Analogously, the DNA_CNB template (which by itself gives negligible transcription) was irradiated at 400 nm for 10 min to yield the deprotected DNA_Chm template which restored its ca. 250% transcription level compared to natural DNA. However, since the 5-HMUDK specifically phosphorylates only Uhm, the treatment of DNA_Chm with 5-HMUDK and ATP did not lead to a phosphorylated template and the transcription still proceeded at the same high level (Scheme 3, Fig. 3, see also Fig. S15B in ESI† for complete uncut gels).
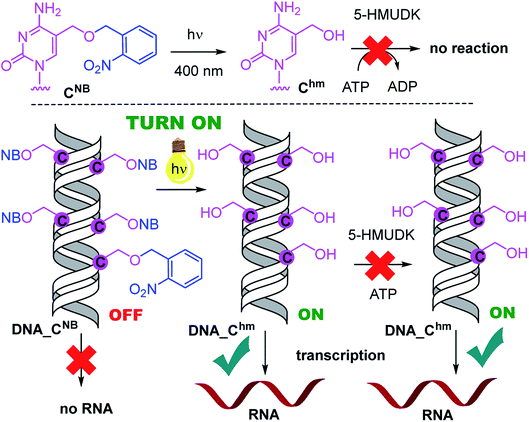 |
| Scheme 3 Switching transcription with photocaged DNA templates containing Chm. | |
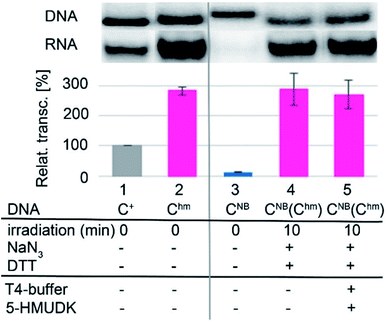 |
| Fig. 3
In vitro transcription from natural DNA (lane 1), DNA_Chm (2) and DNA_CNB (3) templates. Lane 4 shows transcription from DNA_CNB template after 10 min irradiation with λ = 400 nm. Lane 5 shows transcription from DNA_CNB template after irradiation followed by treatment with 5-HMUDK and ATP. | |
In conclusion, we have demonstrated for the first time that bioorthogonal chemical reactions in the major groove of DNA can turn ON or OFF transcription with bacterial RNAP in vitro, similarly to the naturally-occurring DNA methylation and demethylation involved in epigenetic regulations of gene expression.1–15 Previously, we showed that DNA templates containing rare natural Uhm or Chm supported transcription more efficiently than natural DNA, probably by facilitating the recruitment of RNAP to the promoter.29 Now we used nitrobenzyl-photocaging of the hydroxymethylated templates prevent transcription (OFF state), which can be then switched ON through photochemical deprotection using the relatively harmless 400 nm light (at least in low doses).48 In the case of Uhm, the transcription can be switched OFF again by enzymatic phosphorylation. The decreased transcription from DNA_UhmP may indicate that the 5-HMUDK42–44 enzyme can serve as an epigenetic writer to inactivate genes which were accidentally activated due to oxidative formation Uhm or as a defense against bacteriophages that contain DNA bearing this modification,43,44 however a further more detailed study will be needed to confirm this hypothesis. For photocaged Chm, the switch ON through photodeprotection proceeds in the same way as for photocaged Uhm, however, the second switch OFF with 5-HMUDK does not work. Therefore, photocaged Uhm in DNA templates function as a logic gate49–51 with binary transcriptional outputs of 0-1-0, whereas for Chm the outputs are 0-1-1. In principle, further switching could be envisaged by dephosphorylation of DNA_UhmP with a phosphatase or though enzymatic glycosylation of DNA_Chm.52 We are currently working on both of these reactions and, despite some initial unsuccessful experiments, we hope to be able to develop one or both of them to further extend the portfolio of transformations useful for regulation of transcription from modified DNA.
The presented new strategy of photocaging and release control of transcription in the major groove of DNA is conceptually different from previously known photocaging approaches33–36 where the photocaging groups interfere with Watson–Crick pairing of DNA bases preventing duplex formation and therefore the photocaged oligonucleotides (ONs) can only be prepared by chemical synthesis on solid support.33–36 In our approach, the photocaged oligonucleotides (ONs) form stable duplexes and can even be prepared enzymatically by direct polymerase incorporation of the modified nucleotides. We emphasize that the switching has so far only been demonstrated in vitro and any application in cellulo or even in vivo will be still very challenging (although both reactions are in principle biocompatible and bioorthogonal and we have recently reported transport of modified dNTPs and in cellulo incorporation of modified nucleotides into the genomic DNA53). However, this is the proof of principle, the first and essential step towards exciting artificial chemical regulations of gene expression. Follow up studies along these lines using these or other reactions54,55 are under way in our groups.
Experimental
Preparation of fully modified DNA and deprotection of DNA_NNB by light irradiation
Nitrobenzyl- and hydroxymethyl- modified DNA templates (DNA_UNB; DNA_CNB, DNA_Uhm; DNA_Chm) containing specific Pveg promoter region were synthesized in the presence of either NON-labelled or 32P-labelled primers (PrimFOR –32P and PrimREV –32P) by PCR reaction under the conditions reported in ESI (ESI Section 2.3.1–2.3.3†). For the study of deprotection of photolabile nitrobenzyl protecting groups, the purified NB-modified DNAs (DNA_UNB; DNA_CNB) were diluted to the final concentration of approx. 20 ng μL−1 and used as a stock for irradiation experiments. Approx. 240 ng of stock nitrobenzyl-modified DNA (DNA_UNB or DNA_CNB) was irradiated by light from different photodiodes (355 nm, 365 nm or 400 nm) in the particular time intervals (ESI Section 4.2.†). The samples were irradiated either without additives or in the presence of NaN3 and DTT. The irradiated DNA, as well as natural or hm-modified or NB-modified DNA right after PCR, were used as templates for an in vitro transcription assay (ESI Section 4.†).
General procedure for transcription studies of prepared DNA
Transcription studies of prepared DNA templates were performed with RNA polymerase (RNAP) from Escherichia coli (EcoRNAP) – a holoenzyme complexed RNAP with σ70. The resulted transcripts (RNA) were about 145 nucleobases long. Multiple round in vitro transcription assays were performed essentially as described.29,30 The experiments were carried out in total volume 10 μL with 5 ng of DNA template. The reactions proceeded for 10 min at 37 °C after previous preheating of reaction mixture without NTPs. For visualization of prepared RNA product, the transcription was performed in the presence of [α-32P] UTP. The reactions were stopped by the addition of 10 μL of formamide stop solution. The products of transcription were checked by running of 7% polyacrylamide gels. After scanning of exposed gels, the scanned gels were analysed with Quantity One program (BIORAD). For a quantification of relative transcriptions, the transcript signals were normalized to DNA template signals. Signals of transcriptions of modified DNA templates were normalized to the signal of natural DNA (T+ or C+), which was set as 100%. Two–three independent experiments were performed (ESI Section 4.†).
Phosphorylation of hm-modified DNA with 5-HMUDK
Conditions for phosphorylation of hydroxymethyl-moieties on DNA were optimized on DNA_Uhm synthesized in the presence of dUhmTP by PCR. Hydroxymethyl-modified DNA was incubated with different amount of 5-HMUDK (20U; 1.2 μL or 18U; 0.9 μL or 12U; 0.6 μL) at 37 °C for 30 min. The purified phosphorylated DNA (DNA_UhmP) along with natural DNA, which was exposed to the same conditions of phosphorylation were used as templates for transcription studies (ESI Section 5.†).
DNA sample preparation for a study of switching ON and OFF transcription
For a study of switching ON and OFF transcription, the modified DNA templates were synthesized in the presence of 32P-labelled primers by PCR reaction under the reported conditions (ESI Section 2.3.1–2.3.3†). Purified DNA_UNB (cca 240 ng) was irradiated in the presence of additives [1 μL of (1 mM) NaN3 and 1 μL of (50 mM) DTT] with UV lamp (3 W, 400 nm) during 30 min in 1.5 mL Eppendorf tube at room temperature (Scheme S5A, Fig. S15A lane 10 in ESI†). The irradiation experiments were repeated in six portions. After irradiation, all six portions were mixed together and non-purified previously irradiated DNA (400 ng) was incubated under optimized conditions with 5-HMUDK (0.3 μL) in 1× T4 DNA Ligase Reaction Buffer at 37 °C for 30 min (Scheme S5A, Fig. S15A lane 12 in ESI†). As a control of phosphorylation, non-irradiated hydroxymethyl-modified DNA (synthesized by PCR in the presence of dUhmTP) incubated with 5-HMUDK under the same conditions was considered. As a control of selective phosphorylation, natural DNA, DNA_Chm and irradiated DNA_CNB (irradiated under the same conditions as DNA_UNB in time interval 10 min) were also incubated with 0.3 μL of 5-HMUDK at 37 °C during 30 min. In all cases, the DNA right after the reactions were used as templates for the multiple-round in vitro transcription assays (ESI Section 6.†).
Conflicts of interest
There are no conflicts to declare.
Acknowledgements
This work was supported by the Czech Academy of Sciences (RVO: 61388963 and the Praemium Academiae award to M. H.) and by the Czech Science Foundation (17-03419S to Z. V., M. J., L. K. and M. H.). The work was also supported by the Czech research infrastructure for systems biology C4SYS (project LM2015055).
References
- K. Chen, B. S. Zhao and C. He, Cell Chem. Biol., 2016, 23, 74–85 CrossRef CAS PubMed.
- J. X. Lu, B. S. Zhao and C. He, Chem. Rev., 2015, 115, 2225–2239 CrossRef PubMed.
- T. Carell, M. Q. Kurz, M. Müller, M. Rossa and F. Spada, Angew. Chem., Int. Ed., 2018, 57, 4296–4312 CrossRef CAS PubMed.
- C. Luo, P. Hajkova and J. R. Ecker, Science, 2018, 361, 1336–1340 CrossRef CAS PubMed.
- E.-A. Raiber, R. Hardisty, P. van Delft and S. Balasubramanian, Nat. Rev. Chem., 2017, 1, 0069 CrossRef CAS.
- L. Wang, Y. Zhou, L. Xu, R. Xiao, X. Lu, L. Chen, J. Chong, H. Li, C. He, X.-D. Fu and D. Wang, Nature, 2015, 523, 621–625 CrossRef CAS PubMed.
- A. Perera, D. Eisen, M. Wagner, S. K. Laube, A. F. Künzel, S. Koch, J. Steinbacher, E. Schulze, V. Splith, N. Mittermeier, M. Muller, M. Biel, T. Carell and S. Michalakis, Cell Rep., 2015, 11, 283–294 CrossRef CAS PubMed.
- N. Kitsera, J. Allgayer, E. Parsa, N. Geier, M. Rossa, T. Carell and A. Khobta, Nucleic Acids Res., 2017, 45, 11033–11042 CrossRef CAS PubMed.
- X. Cheng and R. M. Blumenthal, Biochemistry, 2010, 49, 2999–3008 CrossRef CAS PubMed.
- T. T. M. Ngo, J. Yoo, Q. Dai, Q. Zhang, C. He, A. Aksimentiev and T. Ha, Nat. Commun., 2016, 7, 10813 CrossRef CAS PubMed.
- A. Jeltsch, ChemBioChem, 2002, 3, 274–293 CrossRef CAS PubMed.
- S. Schiesser, T. Pfaffeneder, K. Sadeghian, B. Hackner, B. Steigenberger, A. S. Schröder, J. Steinbacher, G. Kashiwazaki, G. Höfner, K. T. Wanner, C. Ochsenfeld and T. Carell, J. Am. Chem. Soc., 2013, 135, 14593–14599 CrossRef CAS PubMed.
- K. Iwan, R. Rahimoff, A. Kirchner, F. Spada, A. S. Schröder, O. Kosmatchev, S. Ferizaj, J. Steinbacher, E. Parsa, M. Müller and T. Carell, Nat. Chem. Biol., 2018, 14, 72–78 CrossRef CAS PubMed.
- H. Wu and Y. Zhang, Cell, 2014, 156, 45–68 CrossRef CAS PubMed.
- X. Wu and Y. Zhang, Nat. Rev. Genet., 2017, 18, 517–534 CrossRef CAS PubMed.
- M. Mellén, P. Ayata and N. Heintz, Proc. Natl. Acad. Sci. U. S. A., 2017, 114, E7812–E7821 CrossRef PubMed.
- M. Bachman, S. Uribe-Lewis, X. Yang, M. Williams, A. Murrell and S. Balasubramanian, Nat. Chem., 2014, 6, 1049–1055 CrossRef CAS PubMed.
- A. Viswanathan and P. W. Doetsch, J. Biol. Chem., 1998, 273, 21276–21281 CrossRef CAS PubMed.
- N. Kitsera, D. Stathis, B. Lühnsdorf, H. Müller, T. Carell, B. Epe and A. Khobta, Nucleic Acids Res., 2011, 39, 5926–5934 CrossRef CAS PubMed.
- C. You and Y. Wang, Nat. Protoc., 2015, 10, 1389–1406 CrossRef CAS PubMed.
- C. You, J. Wang, X. Dai and Y. Wang, Nucleic Acids Res., 2015, 43, 1012–1018 CrossRef CAS PubMed.
- A. M. Fleming, Y. Ding and C. J. Burrows, Proc. Natl. Acad. Sci. U. S. A., 2017, 114, 2604–2609 CrossRef CAS PubMed.
- S. Ogasawara, ACS Synth. Biol., 2018, 7, 2507–2513 CrossRef CAS PubMed.
- V. Raindlová, M. Janoušková, M. Slavíčková, P. Perlíková, S. Boháčová, N. Milisavljevič, H. Šanderová, M. Benda, I. Barvík, L. Krásný and M. Hocek, Nucleic Acids Res., 2016, 44, 3000–3012 CrossRef PubMed.
- T. Pfaffeneder, F. Spada, M. Wagner, C. Brandmayr, S. K. Laube, D. Eisen, M. Truss, J. Steinbacher, B. Hackner, O. Kotljarova, D. Schuermann, S. Michalakis, O. Kosmatchev, S. Schiesser, B. Steigenberger, N. Raddaoui, G. Kashiwazaki, U. Müller, C. G. Spruijt, M. Vermeulen, H. Leonhardt, P. Schär, M. Müller and T. Carell, Nat. Chem. Biol., 2014, 10, 574–581 CrossRef CAS PubMed.
- J. Guz, D. Gackowski, M. Foksinski, R. Rozalski and R. Olinski, Biol. Reprod., 2014, 91, 55 Search PubMed.
- R. Olinski, M. Starczak and D. Gackowski, Mutat. Res., 2016, 767, 59–66 CAS.
- F. Kawasaki, D. Beraldi, R. E. Hardisty, G. R. McInroy, P. van Delft and S. Balasubramanian, Genome Biol., 2017, 18, 23 CrossRef PubMed.
- M. Janoušková, Z. Vaníková, F. Nici, S. Boháčová, D. Vítovská, H. Šanderová, M. Hocek and L. Krásný, Chem. Commun., 2017, 53, 13253–13255 RSC.
- M. Slavíčková, M. Janoušková, A. Šimonová, H. Cahová, M. Kambová, H. Šanderová, L. Krásný and M. Hocek, Chem.–Eur. J., 2018, 24, 8311–8314 CrossRef PubMed.
- P. Klan, T. Solomek, C. G. Bochet, A. Blanc, R. Givens, M. Rubina, V. Popik, A. Kostikov and J. Wirz, Chem. Rev., 2013, 113, 119–191 CrossRef CAS PubMed.
- M. Ikeda and M. Kabumoto, Chem. Lett., 2017, 46, 634–640 CrossRef CAS.
- Q. Liu and A. Deiters, Acc. Chem. Res., 2014, 47, 45–55 CrossRef CAS PubMed.
- J. Hemphill, J. Govan, R. Uprety, M. Tsan and A. Deiters, J. Am. Chem. Soc., 2014, 136, 7152–7158 CrossRef CAS PubMed.
- M. A. H. Fichte, X. M. M. Weyel, S. Junek, F. Schäfer, C. Herbivo, M. Goeldner, A. Specht, J. Wachtveitl and A. Heckel, Angew. Chem., Int. Ed., 2016, 55, 8948–8952 CrossRef CAS PubMed.
- L. Kröck and A. Heckel, Angew. Chem., Int. Ed., 2005, 44, 471–473 CrossRef PubMed.
- Z. Vaníková and M. Hocek, Angew. Chem., Int. Ed., 2014, 53, 6734–6737 CrossRef PubMed.
- S. Boháčová, L. Ludvíková, L. Poštová Slavětínská, Z. Vaníková, P. Klán and M. Hocek, Org. Biomol. Chem., 2018, 16, 1527–1535 RSC.
- S. Boháčová, Z. Vaníková, L. Poštová Slavětínská and M. Hocek, Org. Biomol. Chem., 2018, 16, 5427–5432 RSC.
- V. A. Litosh, W. D. Wu, B. P. Stupi, J. C. Wang, S. E. Morris, M. N. Hersh and M. L. Metzker, Nucleic Acids Res., 2011, 39, e39 CrossRef CAS PubMed.
- B. P. Stupi, H. Li, J. C. Wang, W. D. Wu, S. E. Morris, V. A. Litosh, J. Muniz, M. N. Hersh and M. L. Metzker, Angew. Chem., Int. Ed., 2012, 51, 1724–1727 CrossRef CAS PubMed.
- L. M. Iyer, D. Zhang, A. M. Burroughs and L. Aravind, Nucleic Acids Res., 2013, 41, 7635–7655 CrossRef CAS PubMed.
- Y.-J. Lee, N. Dai, S. E. Walsh, S. Müller, M. E. Fraser, K. M. Kauffman, C. Guan, I. R. Corrêa and P. R. Weigele, Proc. Natl. Acad. Sci. U. S. A., 2018, 115, E3116–E3125 CrossRef CAS PubMed.
- P. Weigele and E. A. Raleigh, Chem. Rev., 2016, 116, 12655–12687 CrossRef CAS PubMed.
- L. Sojka, T. Kouba, I. Barvík, H. Šanderová, Z. Maderová, J. Jonák and L. Krásný, Nucleic Acids Res., 2011, 39, 4598–4611 CrossRef CAS PubMed.
- I. Aujard, C. Benbrahim, M. Gouget, O. Ruel, J.-B. Baudin, P. Neveu and L. Jullien, Chem.–Eur. J., 2006, 12, 6865–6879 CrossRef CAS PubMed.
- A. Barth, J. E. T. Corrie, M. J. Gradwell, Y. Maeda, W. Mäntele, T. Meier and D. R. Trentham, J. Am. Chem. Soc., 1997, 119, 4149–4159 CrossRef CAS.
- P. Ramakrishnan, M. Maclean, S. J. MacGregor, J. G. Anderson and M. H. Grant, Toxicol. In Vitro, 2016, 33, 54–62 CrossRef CAS PubMed.
- T. Carell, Nature, 2011, 469, 45–46 CrossRef CAS PubMed.
- R. Ornach, R. Willner and I. Willner, Chem. Commun., 2015, 51, 4144–4160 RSC.
- S. Wang, L. Yue, Z.-Y. Li, J. Zhang, H. Tian and I. Willner, Angew. Chem., Int. Ed., 2018, 57, 8105–8109 CrossRef CAS PubMed.
- P. Borst and R. Sabatini, Annu. Rev. Microbiol., 2008, 62, 235–251 CrossRef CAS PubMed.
- P. Güixens-Gallardo, Z. Zawada, J. Matyasovsky, D. Dziuba, R. Pohl, T. Kraus and M. Hocek, Bioconjugate Chem., 2018, 29, 3906–3912 CrossRef PubMed.
- P. Kielkowski, H. Macíčková-Cahová, R. Pohl and M. Hocek, Angew. Chem., Int. Ed., 2011, 50, 8727–8730 CrossRef CAS PubMed.
- M. Krömer, K. Bártová, V. Raindlová and M. Hocek, Chem.–Eur. J., 2018, 24, 11890–11894 CrossRef PubMed.
Footnotes |
† Electronic supplementary information (ESI) available: Contains detailed experimental procedures, characterization data and additional figures and gels. See DOI: 10.1039/c9sc00205g |
‡ These authors contributed equally. |
|
This journal is © The Royal Society of Chemistry 2019 |
Click here to see how this site uses Cookies. View our privacy policy here.