DOI:
10.1039/C9SC03957K
(Edge Article)
Chem. Sci., 2019,
10, 9949-9953
Chloromethyl-modified Ru(II) complexes enabling large pH jumps at low concentrations through photoinduced hydrolysis†
Received
8th August 2019
, Accepted 15th October 2019
First published on 24th October 2019
Abstract
Photoacid generators (PAGs) are finding increasing applications in spatial and temporal modulation of biological events in vitro and in vivo. In these applications, large pH jumps at low PAG concentrations are of great importance to achieve maximal expected manipulation but minimal unwanted interference. To this end, both high photoacid quantum yield and capacity are essential, where the capacity refers to the proton number that a PAG molecule can release. Up to now, most PAGs only produce one proton for each molecule. In this work, the hydrolysis reaction of benzyl chlorides was successfully leveraged to develop a novel type of PAG. Upon visible light irradiation, Ru(II) polypyridyl complexes modified with chloromethyl groups can undergo full hydrolysis with photoacid quantum yield as high as 0.6. Depending on the number of the chloromethyl groups, the examined Ru(II) complexes can release multiple protons per molecule, leading to large pH jumps at very low PAG concentrations, a feature particularly favorable for bio-related applications.
Introduction
Photoacid generators (PAGs) are molecules which can generate acids under light irradiation,1 and have been widely used as photo-initiators of cationic polymerization in photolithography, photocuring, and three-dimensional (3D) printing.2 The great success that PAGs have achieved in the fields of the microelectronic industry and microfabrication relies on their spatial and temporal control of proton concentrations. Protons also play pivotal roles in a variety of biological events. As a result, PAGs have found ever-increasing applications in biochemical, biological, and biomedical areas in recent years, such as photodynamic therapy,3 drug delivery,4 adenosine triphosphate (ATP) biosynthesis,5 photocontrol of enzyme activity,6 and protein conformations,7 as well as proton transfer in biomolecules.8 For these bio-related applications, PAGs may have some specific characters,9 including proper solubility in aqueous solutions, light response in the visible or near-infrared region for deep tissue penetration and minimal damage to biomolecules,10 and large pH jumps to get remarkable bio-effects. To reach instant large pH jumps, high photoacid quantum yields were vigorously pursued. However, mM levels of PAGs, concentrations not easy to realize under in vitro and in vivo conditions, were usually needed because each PAG molecule can theoretically generate one proton only.4a,5–7 High concentrations of PAGs and their corresponding photoproducts may also interfere with the examined biological processes, hampering their application severely. Though efforts have been made to address this issue by integrating two PAG moieties into a single molecule, the resultant PAGs showed poor photoacid quantum yields.1c,3 New strategies that can endow PAGs with both high photoacid capacity (i.e. the proton number a PAG molecule can release) and high photoacid quantum yield are in urgent demand to boost their bio-related utilization.
Thermal hydrolysis of haloalkanes may release HX (X = Cl, Br, I) and the acid capacity depends strictly on their halogenation levels.11 To the best of our knowledge, such a classic reaction has not been capitalized on in PAGs. What attracts our attention is the hydrolysis of benzyl chloride, the kinetics of which can be effectively modulated by electron donating/withdrawing groups.12 Electron donating groups will accelerate the process while an opposite effect may be observed for electron withdrawing groups. As reported by A. Fry and S. Yamabe, para-methoxy benzyl chloride has a hydrolysis rate of 4 orders of magnitude higher than that of the para-NO2 counterpart.12 Inspired by these facts, we herein synthesized a series of 4,4′-bis(chloromethyl)-2,2′-bipyridine (bcm–bpy) coordinated Ru(II) complexes (1–3, Scheme 1) to explore their PAG capability. The rationale behind our design is as follows. (1) The bcm–bpy ligand is expected to be inactive in thermal hydrolysis due to the electron-deficient feature of the pyridine ring, which may be consolidated further upon coordination to the Ru(II) center.13 A good stability in the dark is a prerequisite for a desired PAG. (2) The highest occupied molecular orbital (HOMO) of Ru(II) polypyridyl complexes is generally Ru(II) centered,14 while the lowest unoccupied molecular orbital (LUMO) of complexes 1–3 should localize on the bcm–bpy ligand due to the electronegativity of Cl atoms.15 Thus, the bcm–bpy related metal-to-ligand charge transfer (MLCT) state will be accessed preferentially upon light irradiation, from which an efficient hydrolysis of the chloromethyl groups is also anticipated due to the greatly enhanced electron density on the bcm–bpy ligand. This is indeed what we observed in our experiments. Complexes 1–3 can undergo efficient hydrolysis upon visible light irradiation in aqueous solutions, and release 2, 4, and 6 equivalents of HCl, respectively, with photoacid quantum yields as high as 0.6. Both high photoacid capacity and high photoacid quantum yield of complex 3 make large pH jumps feasible at low concentrations. Complex 3 is not only the first PAG which can release six protons per molecule, but also the first type of PAG that makes use of hydrolysis reaction of benzyl chloride groups. As a conceptual demonstration, complex 3 was successfully used to switch on the activity of acid phosphatase upon visible light irradiation at a concentration as low as 10 μM.6
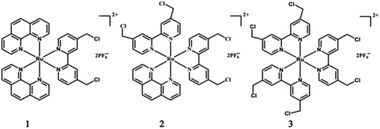 |
| Scheme 1 Chemical structures of complexes 1–3. | |
Results and discussion
Theoretical calculations
Theoretical calculations based on the Gaussian 09 program package16 (see ESI†) were carried out before experiments to examine our ideas. As expected, the calculated HOMO and LUMO of 1–3 are mainly Ru(II) and bcm–bpy based, respectively (Fig. 1 and S1–S2†). Thus MLCTRu→bcm–bpy excitation means pumping one electron from the Ru(II) center to the bcm–bpy ligand, which will greatly enhance the electron density of bcm–bpy. Such an expectation is further convinced by comparison of Mulliken charges of the ground state (GS) and T1 state. Taking complex 1 as an example (Table S1†), the selected Mulliken charges of the atoms on the bcm–bpy ligand in the T1 state are all negatively shifted compared with that in the GS. In addition, the length of C–Cl bonds stretches from 1.83 Å (GS) to 1.85 Å (T1) (Fig. S3–S5†). All these results are favorable for photoinduced hydrolysis of the chloromethyl groups.
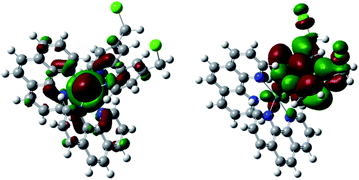 |
| Fig. 1 HOMO (left) and LUMO (right) of complex 1. | |
Synthesis and characterization
The synthesis and characterization of complexes 1–3 were reported in our previous work.13 The aqueous solutions of all the complexes are quite stable in the dark, as evidenced by the negligible changes in absorption spectra, emission spectra, and 1H NMR (nuclear magnetic resonance) spectra, as well as pH values of the solutions (Fig. S7†). Upon irradiation at 520 nm, rapid changes in these spectra were observed. Taking complex 3 as an example, the absorbance in the regions of 315–375 nm and 425–500 nm decreased gradually, along with a slight blueshift of the ligand-based transition peak centered at 291 nm (Fig. 2). The emission intensity also increased quickly upon irradiation (Fig. 2). The absorption and emission spectra did not change any more after irradiation for only 3 min. At that time, the solution exhibited nearly the same absorption and emission as that of [Ru(bhm-bpy)3]2+ (20 μM, bhm-bpy = 4,4′-bis(hydroxymethyl)-2,2′-bipyridine), suggesting full hydrolysis of the six chloromethyl groups. The excited [Ru(bhm-bpy)3]2+ without competing photolysis decay should have a longer excitation lifetime and also a higher luminescence quantum yield compared with complex 3, and thus a turn-on luminescence was observed. The known PAGs usually show absorption changes upon light irradiation. Our complexes represent the first class of PAGs that exhibit remarkable luminescence turn-on along with proton release. By virtue of the higher sensitivity of fluorescence detection as well as the wide application of fluorescence confocal microscopy, this feature may facilitate biological studies. In addition, the transformation of 3 into [Ru(bhm-bpy)3]2+ is also confirmed by high resolution electron spray ionization mass spectra (HR ESI-MS) (Fig. S16†) and 1H NMR spectra (Fig. 3). Only an m/z peak of 375.0861 which can be ascribed to the product with six hydroxymethyl groups was observed after irradiation in H2O. A similar result was obtained in H218O (Fig. S19†), and the 18O labeled Ru product was observed. The 1H NMR signals of 3 displayed a slight shift to higher field upon irradiation, and finally overlapped with that of [Ru(bhm-bpy)3]2+. Similar results were also obtained for complexes 1 and 2 (Fig. S8–S15, S17 and S18†).
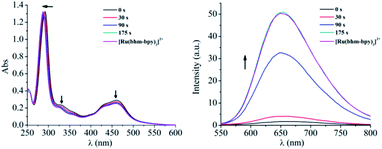 |
| Fig. 2 Absorption (left) and emission (right) spectral changes of 3 (20 μM) in H2O upon irradiation (520 nm), compared with that of [Ru(bhm-bpy)3]2+ (20 μM in H2O). | |
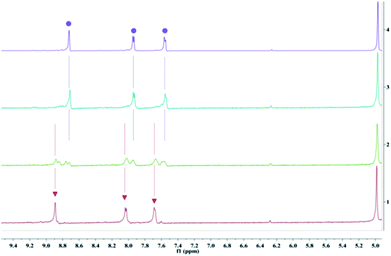 |
| Fig. 3
1H NMR spectra of 3 in D2O before (1, peaks were marked with the red triangles) and after irradiation at 520 nm for 5 min (2) and 20 min (3), and 1H NMR spectrum of [Ru(bhm-bpy)3]2+ (4, peaks were marked with the purple dots). | |
pH changes
The pH changes of the aqueous solutions of complexes 1–3 (10 μM) were monitored with a pH meter (Fig. 4). The initial pH values of the solutions were about 6.6. The weak acidity may be attributable to the dissolved CO2 absorbed from ambient air.17 The pH values of the solutions kept unchanged in the dark for 24 h (Fig. S7†). Upon LED (light-emitting diode) irradiation at 520 nm, the pH values decreased quickly to 4.9 for 1, 4.6 for 2 and 4.3 for 3 in 3 min (the theoretically calculated results are 4.7, 4.4 and 4.2 for complexes 1–3, respectively). A large pH jump of 2.3 units was obtained for complex 3 at a concentration as low as 10 μM, which obviously profits from its ability to generate 6 protons for each molecule. To reach a similar pH jump, much larger concentrations, usually several hundreds of micromolar, were generally needed for the reported systems,6,17,18 unfavorable for biological applications.
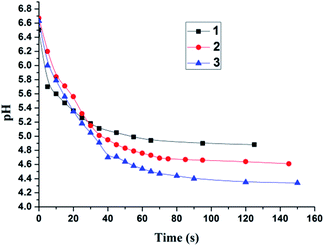 |
| Fig. 4 pH changes of 1–3 (10 μM) in H2O upon 520 nm LED irradiation. | |
In bio-related applications, bioactive molecules, such as glutathione (GSH), may serve as strong nucleophilic agents to impair the dark stability of these chloromethyl-modified Ru complexes.19 Thus, the effect of GSH was also studied. The initial pH value of a GSH (1 mM) aqueous solution was measured to be 3.4. Addition of 1–3 (10 μM) did not cause any pH changes in 24 h without irradiation (Fig. S20†). The negligible pH changes may also be the result of the strong buffering ability of GSH. To rule out this possibility, ethanethiol (1 mM) was used instead of GSH. Similar results were obtained again at either room temperature or 37 °C (Fig. S21 and S24†), confirming the good dark stability of complexes 1–3 even in the presence of strong nucleophilic agents. Upon 520 nm irradiation, the pH values of 1–3 (10 μM) solutions containing ethanethiol decreased quickly (Fig. S22–S24†), showing their desirable anti-interference ability.
Photoacid quantum yields
The photoacid quantum yields of 1–3 (50 μM) in H2O were measured using potassium ferrioxalate as a chemical actinometer (ESI).20 The light of 520 nm was not suitable for potassium ferrioxalate due to its quite small extinction coefficient at 520 nm, and thus a 470 nm LED was selected. The obtained quantum yields at 470 nm are 0.62 ± 0.01 for complex 1, 0.65 ± 0.02 for complex 2 and 0.61 ± 0.01 for complex 3, which are among the highest reported for PAGs.1c,d,2a,b
Photoacid mechanism
The high photoacid quantum yields and photoacid capacities of 1–3, along with their good dark stability, make the hydrolysis mechanism of the chloromethyl groups anchored on these complexes particularly interesting. Generally, a thermal hydrolysis of a haloalkane may undergo through either an SN1 (unimolecular) or an SN2 (bimolecular) pathway.12 For benzyl chlorides, the mechanism may change from SN1 to SN2 depending on the electron donating/withdrawing abilities of substituents. According to the reports of A. Fry and S. Yamabe, the hydrolysis of para-methoxy benzyl chloride proceeds through an SN1 way, while SN2 is found for the para-NO2 compound. For complexes 1–3, MLCT excitation enhances the electron density of the bcm–bpy ligand, which is favorable for SN1 rather than SN2 Based on the theoretical and experimental results, a possible photoacid mechanism is schematically illustrated in Scheme 2, taking complex 1 as an example. Furthermore, we also examined the photolysis of the bcm–bpy ligand in H2O. The absorption of the free bcm–bpy ligand appears below 310 nm. Under direct UV light (254 nm) irradiation, HCl was also generated, most probably through a different mechanism involving homolysis of a carbon–chorine bond as reported by R. Sinta et al. in the studying of photoacid properties of 4,6-bis(trichloromethyl)-1,3,5-triazines.21 Compared with the clean transformation of 1–3 into their corresponding hydroxymethyl products, the photolysis of bcm–bpy is very complicated. The absorption spectra changed in an irregular way (Fig. S25†), and complex photoproducts were obtained as evidenced by the mass spectrum (Fig. S26†), in line with a homolysis-based radical mechanism. As a result, only 0.8 unit of pH change was observed at 10 μM of bcm–bpy (Fig. S25†), in sharp contrast to complex 1, which led to 1.6 units of pH jump at the concentration of 10 μM upon visible light irradiation.
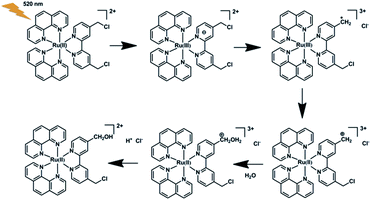 |
| Scheme 2 A proposed photoacid mechanism for 1. | |
To the best of our knowledge, this is the first time that the hydrolysis reaction was applied to develop a novel type of PAG. Since their discovery four decades ago, PAGs have been dominated by iodonium and sulfonium salts. Only over the past decade, new structures along with their unique properties have emerged in the PAG arsenal, such as photochromic triangle terarylenes,1e,2a the open form of spiropyrans,17,18 and N-oxyimidesulfonates.22 Bearing in mind that the benzyl chloride groups are easy to integrate into a variety of photosensitizers, such as porphyrins, phthalocyanines, and boron dipyrromethene (BODIPY) dyes, more diverse PAG structures and their broader applications are expected.
Biological applications
As a conceptual demonstration of its possible biological applications, complex 3 at 10 μM was used to modulate the activity of acid phosphatase (ACP).6 ACPs are widely distributed in the human body. An abnormal activity of ACPs is related to many diseases, including prostate cancer, kidney disease, multiple myeloma, Gaucher disease, etc.23 Therefore, controlling the ACP activity may find potential applications in disease treatment and drug development. S. Kohse and co-workers successfully utilized a photoacid (2-nitrobenzaldehyde) to realize efficient tuning of the ACP activity.6 Similarly, 4-methylumbelliferyl phosphate (MUP) was selected as the substrate of ACP. The transformation of MUP into 4-methylumbelliferone (MU) was monitored by absorption spectra. The initial pH of the solution of MUP and acid phosphatase was kept at 8.0, where the activity of the enzyme was faint as negligible spectral changes were observed. Upon addition of complex 3 (10 μM) and irradiation for 90 s with an LED at 520 nm, the activity of the enzyme was successfully switched on, and elevated to a level of 0.05 μmol (min mgenzyme)−1 (Fig. 5 and S27†), which is consistent with the reported results.6 However, a PAG concentration as high as 500 μM was implemented in their experiments. Light irradiation in the control experiment without the enzyme caused no effects on MUP (Fig. S28†).
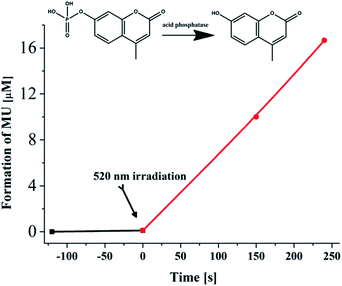 |
| Fig. 5 Hydrolytic conversion of MUP to MU by acid phosphatase (0.08 mg mL−1) in the presence of 3 (10 μM) before (dark line) and after 520 nm irradiation (4 mW cm−2, red line). | |
Conclusions
In conclusion, we designed and synthesized three bcm–bpy based Ru(II) complexes as novel PAGs, which can work in aqueous solution and be excited by green light with photoacid quantum yields of about 0.6. Complex 3 can release six protons per molecule, leading to large pH jumps at low concentrations. Theoretically, more protons may be released provided more chloromethyl groups are anchored on the ligand. Our results may open new avenues for developing novel PAGs with both high photoacid quantum yield and capacity to meet more challenging demands particularly in bio-related areas.
Conflicts of interest
There are no conflicts to declare.
Acknowledgements
This work was financially supported by the National Key R&D Program of China (2018YFC1602204), the NSFC (21390400, 21571181 and 21773277) and the Strategic Priority Research Program of Chinese Academy of Sciences (XDB17000000). We also appreciate the funding and technical support from the Shanghai Supercomputer Center in theoretical calculations.
Notes and references
-
(a) J. V. Crivello, J. Photopolym. Sci. Technol., 2009, 22, 575–582 CrossRef CAS;
(b) M. Shirai and M. Tsunooka, Prog. Polym. Sci., 1996, 21, 1–45 CrossRef CAS;
(c) C. O. Yanez, C. D. Andrade and K. D. Belfield, Chem. Commun., 2009, 827–829 RSC;
(d) M. Jin, H. Xu, H. Hong, J. P. Malval, Y. Zhang, A. Ren, D. Wan and H. Pu, Chem. Commun., 2013, 49, 8480–8482 RSC;
(e) T. Nakashima, K. Tsuchie, R. Kanazawa, R. Li, S. Lijima, O. Galangau, H. Nakagawa, K. Mutoh, Y. Kobayashi, J. Abe and T. Kawai, J. Am. Chem. Soc., 2015, 137, 7023–7026 CrossRef CAS PubMed.
-
(a) R. Li, T. Nakashima, R. Kanazawa, O. Galangau and T. Kawai, Chem.–Eur. J., 2016, 22, 16250–17257 CrossRef CAS PubMed;
(b) W. Zhou, S. M. Kuebler, D. Carrig, J. W. Perry and S. R. Marder, J. Am. Chem. Soc., 2002, 124, 1897–1901 CrossRef CAS PubMed;
(c) C. K. Ober, J. W. Perry and S. R. Marder, Science, 2002, 296, 1106–1109 CrossRef PubMed;
(d) H. Ito, J. Polym. Sci., Part A: Polym. Chem., 2003, 41, 3863–3870 CrossRef CAS.
- X. Yue, C. O. Yanez, S. Yao and K. D. Belfield, J. Am. Chem. Soc., 2013, 135, 2112–2115 CrossRef CAS PubMed.
-
(a) D. He, X. He, K. Wang, Z. Zou, X. Yang and X. Li, Langmuir, 2014, 30, 7182–7189 CrossRef CAS PubMed;
(b) P. Shum, J. M. Kim and D. H. Thompson, Adv. Drug Delivery Rev., 2001, 53, 273–284 CrossRef CAS;
(c) Z. Song, Y. Wu, Q. Cao, H. Wang, X. Wang and H. Han, Adv. Funct. Mater., 2018, 28, 1800011 CrossRef;
(d) H. W. Choi, J. Kim, J. Kim, Y. Kim, H. B. Song, J. H. Kim, K. Kim and W. J. Kim, ACS Nano, 2016, 10, 4199–4208 CrossRef CAS PubMed.
-
(a) Y. Xu, J. Fei, G. Li, T. Yuan, Y. Li, C. Wang, X. Li and J. Li, Angew. Chem., Int. Ed., 2017, 56, 12903–12907 CrossRef CAS PubMed;
(b) Y. Li, X. Feng, A. Wang, Y. Yang, J. Fei, B. Sun, Y. Jia and J. Li, Angew. Chem., Int. Ed., 2019, 58, 796–800 CrossRef CAS PubMed.
- S. Kohse, A. Neubauer, A. Pazidis, S. Lochbrunner and U. Kragl, J. Am. Chem. Soc., 2013, 135, 9407–9411 CrossRef CAS PubMed.
-
(a) S. Abbruzzetti, E. Crema, L. Masino, A. Vecli, C. Viappiani, J. R. Small, L. J. Libertini and E. W. Small, Biophys. J., 2000, 78, 405–415 CrossRef CAS PubMed;
(b) A. Barth and J. E. T. Corrie, Biophys. J., 2002, 83, 2864–2871 CrossRef CAS PubMed;
(c) S. Abbruzzetti, S. Sottini, C. Viappiani and J. E. T. Corrie, Photochem. Photobiol. Sci., 2006, 5, 621–628 RSC;
(d) F. I. Rosell and A. G. Mauk, Coord. Chem. Rev., 2011, 255, 737–756 CrossRef CAS.
-
(a) J. J. V. Thor, Photoreactions and dynamics of the green fluorescent protein, Chem. Soc. Rev., 2009, 38, 2935–2950 RSC;
(b) P. Chou and K. M. Solntsev, J. Phys. Chem. B, 2015, 119, 2089 CrossRef CAS PubMed.
-
(a) P. Klán, T. Šolomek, C. G. Bochet, A. Blanc, R. Givens, M. Rubina, V. Popik, A. Kostikov and J. Wirz, Chem. Rev., 2013, 113, 119–191 CrossRef PubMed;
(b) A. P. Pelliccioli and J. Wirz, Photochem. Photobiol. Sci., 2002, 1, 441–458 RSC.
-
(a) D. K. Chatterjee, L. S. Fong and Y. Zhang, Adv. Drug Delivery Rev., 2008, 60, 1627–1637 CrossRef CAS PubMed;
(b) M. R. Detty, S. L. Gibson and S. J. Wagner, J. Med. Chem., 2004, 47, 3897–3915 CrossRef CAS PubMed.
-
(a) R. E. Robertson and J. M. W. Scott, J. Chem. Soc., 1961, 1596–1604 RSC;
(b) S. Koshy and R. Anantaraman, J. Am. Chem. Soc., 1960, 82, 1574–1576 CrossRef CAS.
-
(a) J. W. Hill and A. Fry, J. Am. Chem. Soc., 1962, 84, 2763–2769 CrossRef CAS;
(b) S. Yamabe, G. Zeng, W. Guan and S. Sakaki, J. Comput. Chem., 2014, 35, 1140–1148 CrossRef CAS PubMed.
- N. Tian, W. Sun, X. Guo, J. Lu, C. Li, Y. Hou, X. Wang and Q. Zhou, Chem. Commun., 2019, 55, 2676–2679 RSC.
-
(a) A. Juris, V. Balzani, F. Barigelletti, S. Campagna, P. Belser and A. V. Zelewsky, Coord. Chem. Rev., 1988, 84, 85 CrossRef CAS;
(b) Q. X. Zhou, F. Yang, W. H. Lei, J. R. Chen, C. Li, Y. J. Hou, X. C. Ai, J. P. Zhang, X. S. Wang and B. W. Zhang, J. Phys. Chem. B, 2009, 113, 11521–11526 CrossRef CAS PubMed;
(c) D. A. Lutterman, A. A. Rachford, J. J. Rack and C. Turro, J. Phys. Chem. A, 2009, 113, 11002–11006 CrossRef CAS PubMed.
- P. S. Wagenknecht and P. C. Ford, Coord. Chem. Rev., 2011, 255, 591–616 CrossRef CAS.
-
M. J. Frisch, G. W. Trucks, H. B. Schlegel, G. E. Scuseria, M. A. Robb, J. R. Cheeseman, G. Scalmani, V. Barone, B. Mennucci, G. A. Petersson, H. Nakatsuji, M. Caricato, X. Li, H. P. Hratchian, A. F. Izmaylov, J. Bloino, G. Zheng, J. L. Sonnenberg, M. Hada, M. Ehara, K. Toyota, R. Fukuda, J. Hasegawa, M. Ishida, T. Nakajima, Y. Honda, O. Kitao, H. Nakai, T. Vreven, J. A. Montgomery Jr, J. E. Peralta, F. Ogliaro, M. Bearpark, J. J. Heyd, E. Brothers, K. N. Kudin, V. N. Staroverov, R. Kobayashi, J. Normand, K. Raghavachari, A. Rendell, J. C. Burant, S. S. Iyengar, J. Tomasi, M. Cossi, N. Rega, J. M. Millam, M. Klene, J. E. Knox, J. B. Cross, V. Bakken, C. Adamo, J. Jaramillo, R. Gomperts, R. E. Stratmann, O. Yazyev, A. J. Austin, R. Cammi, C. Pomelli, J. W. Ochterski, R. L. Martin, K. Morokuma, V. G. Zakrzewski, G. A. Voth, P. Salvador, J. J. Dannenberg, S. Dapprich, A. D. Daniels, Ö. Farkas, J. B. Foresman, J. V. Ortiz, J. Cioslowski, and D. J. Fox, Gaussian 09, revision A.1, Gaussian, Inc., Wallingford, CT, 2009 Search PubMed.
- N. Abeyrathna and Y. Liao, J. Am. Chem. Soc., 2015, 137, 11282–11284 CrossRef CAS PubMed.
- Z. Shi, P. Peng, D. Strohecker and Y. Liao, J. Am. Chem. Soc., 2011, 133, 14699–14703 CrossRef CAS PubMed.
-
(a) M. H. Lee, N. Park, C. Yi, J. H. Han, J. H. Hong, K. P. Kim, D. H. Kang, J. L. Sessler, C. Kang and J. S. Kim, J. Am. Chem. Soc., 2014, 136, 14136–14142 CrossRef CAS PubMed;
(b) L. Long, M. Huang, N. Wang, Y. Wu, K. Wang, A. Gong, Z. Zhang and J. L. Sessler, J. Am. Chem. Soc., 2018, 140, 1870–1875 CrossRef CAS PubMed.
-
M. Montalti, A. Credi, L. Prodi, and M. T. Gandolfi, Handbook of photochemistry, CRC Press, Boca Raton, 3rd edn, 2006, ch. 12 Search PubMed.
- G. Pohlers and J. C. Scaiano, Chem. Mater., 1997, 9, 1353–1361 CrossRef CAS.
-
(a) F. Ortica, C. Coenjarts, J. C. Scaiano, H. Liu, G. Pohlers and J. F. Cameron, Chem. Mater., 2001, 13, 2297–2304 CrossRef CAS;
(b) M. Ikbal, R. Banerjee, S. Atta, D. Dhara, A. Anoop and N. D. P. Singh, J. Org. Chem., 2012, 77, 10557–10567 CrossRef CAS PubMed.
- H. Bull, P. G. Murray, D. Thomas, A. M. Fraser and P. N. Nelson, Mol. Pathol., 2002, 55, 65–72 CrossRef CAS PubMed.
Footnote |
† Electronic supplementary information (ESI) available. See DOI: 10.1039/c9sc03957k |
|
This journal is © The Royal Society of Chemistry 2019 |
Click here to see how this site uses Cookies. View our privacy policy here.