DOI:
10.1039/C9AN01945F
(Paper)
Analyst, 2020,
145, 249-256
Amphiphilic ligand modified gold nanocarriers to amplify lanthanide loading for ultrasensitive DELFIA detection of Cronobacter†
Received
30th September 2019
, Accepted 28th October 2019
First published on 28th October 2019
Abstract
Conventional dissociation-enhanced lanthanide (Ln3+) fluoroimmunoassays (DELFIAs) using Ln3+ chelate-labeled antibodies as molecular probes exhibit limited sensitivity because of their relatively low Ln3+ labeling ratio per biomolecule. Herein, we applied gold nanoflowers (AuNFs) as amplified nanocarriers to increase the Ln3+ labeling ratio in a single molecular binding event for improving the sensitivity of traditional DELFIA. Two thiolated amphiphilic ligands (thiolated ethylenediaminetetraacetic acid (EDTA) and thiolated acylhydrazine-terminated ligands), consisting of a hydrophobic alkane chain, oligo(ethylene glycol) unit, and functional terminal of the EDTA or acylhydrazine group, were designed for the surface modification of AuNFs. The resultant ligand-coated AuNFs exhibited dual functions of Ln3+ chelation via the EDTA group and oriented attachment of antibodies via the acylhydrazine group. By utilizing 80 nm AuNFs as amplified carriers, we demonstrated that the maximum Eu3+ loading amount reached 1.07 × 104 Eu3+ ions per AuNF, which is approximately two to three orders of magnitude higher than that of traditional molecular probes, thereby amplifying the luminescence signal and enhancing the sensitivity of DELFIA. By combining a magnetic-mediated sandwich-type DELFIA method, the designed amplified AuNF nanoprobes achieved an ultrasensitive luminescence detection of Cronobacter muytjensii with a limit of detection (LOD) of 1.2 × 102 cfu mL−1 in a powdered infant formula. This LOD value was ca. 230-fold lower than that of the traditional colorimetric immunoassay. The designed signal amplification strategy using bifunctional ligand-modified AuNFs as enhanced Ln3+ nanocarriers provided a huge potential for building various ultrasensitive luminescence immunoassays for in vitro biodetection.
1. Introduction
Immunoassays that depend on the highly specific molecular recognition between an antigen and antibody have developed into a primary analytical tool for in vitro biodetection.1 Given their outstanding advantages of high sensitivity and excellent specificity, immunoassays have acquired wide applications in disease diagnosis, food safety inspection, and environmental monitoring.2 Currently, various immunoassay-based technologies have been fabricated through the simple integration of the conventional immunoassay format with a series of signal transducers, such as colorimetry,3 fluorescence,4 chemiluminescence,5 electrochemistry,6 light scattering,7 chirality,8 and magnetic signal.9 Among them, fluorescence immunoassay is an appealing substitute for traditional colorimetric immunoassay because of its intrinsic superior sensitivity.10 The dissociation-enhanced lanthanide (Ln3+) fluoroimmunoassay (DELFIA), as one of the most sensitive fluorescence bioanalysis methods, has attracted increasing interest since it was first reported by Siitari et al. in the 1980s.11 In general, the DELFIA technique commonly uses europium (Eu3+) chelate-labeled antibodies as molecular probes to concatenate the specific immunological recognition of targets with an Eu3+-based luminescent signal transducer. In a typical DELFIA, an acidic enhancer solution is introduced at the end of the immunoreaction to induce the nonluminescent Eu3+ chelate labeled on the antibody for its transformation into a highly luminescent Eu3+ micelle,12 resulting in a significantly enhanced luminescence via the “antenna effect” from the enhancer solution.13 Moreover, by utilizing the time-resolved luminescence (TRL) technique, the background signal noise originating from scattered light and short-lived autofluorescence can be completely eliminated in the DELFIA method, thus providing remarkably increased sensitivity.14 Nevertheless, traditional molecular probes based on Eu3+ chelate-coupled antibodies as signal labels often suffer from several drawbacks, including a low labeling ratio (ca. 10–30 Eu3+ chelate per antibody) for producing limited TRL signals for insufficient sensitivity and poor chemical stability.15 These defects restrain the wide application of the conventional DELFIA approach to some extent.
Theoretically, increasing the amount of Eu3+ chelate labels in a single molecular binding event can improve the detection sensitivity of the conventional DELFIA method.13,16 Chen et al. reported a new strategy by synthesizing a hybridization Eu3+-activated inorganic nanoparticle as the Eu3+ ion container for amplifying the TRL signal.13 Nonetheless, the synthesis of the inorganic Eu3+ nanoparticle is often in the oil phase,17 and thus, postsynthesis surface modification is needed to render the hydrophobic nanoparticle water soluble and biocompatible, which is relatively complicated and partly limits their practical application. Nanocarriers are nanoparticle-based signal amplification systems in which the large surface areas of nanoparticles can endow their superior capabilities to carry various cargoes, including drugs, enzymes, and signal generating molecules. By virtue of their super-high loading capacity, nanocarriers have been widely utilized in drug delivery, nanomedicine, bioreactors, and bioanalysis.18 Previous studies have indicated that multibranched gold nanoflowers (AuNFs) have a larger surface area than gold nanospheres with a similar size, thereby further improving the cargo loading capacity.19
In this work, we designed thiolated amphiphilic ligand-modified AuNFs as amplified nanocarriers to increase the labeling ratio of Eu3+ chelates for improving the sensitivity of the traditional DELFIA method. To realize the loading of Eu3+ chelates and the antibody functionalization on the AuNF surface, two thiolated amphiphilic ligands, namely, thiolated ethylenediaminetetraacetic acid-terminated ligand (thiol-EDTA) and thiolated acylhydrazine-terminated ligand (thiol-NHNH2), were designed and synthesized. The thiolated amphiphilic ligand consisted of a hydrophobic alkane chain for increased stability, an oligo(ethylene glycol) unit for improved hydrophilicity and biocompatibility, and a functional terminal of EDTA or acylhydrazine group for the Eu3+ chelation or the oriented coupling of the antibody. To validate the feasibility of our design, Cronobacter was selected as the model analyte because its contamination in powdered infant formula (PIF) can cause the occurrence of premature and immunocompromised infants. After covalent linkage with antibodies against Cronobacter muytjensii ATCC 51329, the resultant amplified AuNF nanocarriers were applied as novel luminescent lanthanide nanoprobes in a magnetic-mediated sandwich-type DELFIA method for C. muytjensii detection. We also evaluated the accuracy, specificity, reliability, reproducibility, and practicability of the proposed method in the spiked PIF sample. To the best of our knowledge, this study is the first to use thiolated amphiphilic ligand-modified AuNFs as nanocarriers to increase the loading of Eu3+ chelates for improving the sensitivity of the traditional DELFIA method. We believe that the designed AuNF amplification strategy can be readily adapted for the ultrasensitive luminescence detection of other targets in vitro by simply replacing the recognition elements.
2 Materials and methods
2.1 Materials
Hydrogen tetrachloroaurate(III) hydrate (HAuCl4·3H2O), trisodium citrate, hydroquinone, N-(3-dimethylaminopropyl)-N′-ethylcarbodiimide hydrochloride (EDC·HCl), trichloroethyl phosphate (TCEP), europium(III) chloride hexahydrate, 2-thenoyltrifluoroacetone (TTA), trioctylphosphine oxide (TOPO), Triton X-100, and bovine serum albumin (BSA) were purchased from Sigma-Aldrich Chemical Co. (St Louis, MO, USA). Luria–Bertani (LB) broth and Baird–Parker agar base were provided by Land Bridge Technology Co. Ltd (Beijing, China). Monoclonal antibodies (mAbs) against C. muytjensii ATCC 51329 were prepared in our laboratory and simultaneously applied as the captured (cAbs) and detected (dAbs) antibodies. Moreover, 96-well polystyrene plates (high binding, black) were obtained from Costar Inc. (Cambridge, MA, USA). Carboxylated magnetic beads (MBs) with the size of 400–500 nm were obtained from Tianjin Baseline ChromTech Research Centre (Tianjin, China). All other chemicals applied without further purification were of analytical grade and purchased from Sinopharm Chemical Corp. (Shanghai, China). The bacterial strains used in this work were obtained from the American Type Culture Collection (ATCC), China Center of Industrial Culture Collection (CICC), and China Medical Culture Collection (CMCC). Table S1† summarizes the details of all used strains. All strains were cultured in LB medium (Oxoid, Basingstoke, UK) and inactivated before use on the basis of our previous report.
2.2 Instruments
The morphology and size of AuNFs were determined on a high-resolution transmission electron microscope (TEM, JEOL JEM 2100, Tokyo, Japan). The UV–vis absorption spectra of AuNFs before/after surface modification were recorded on an Amersham Pharmacia Ultrospec 4300 pro UV/visible spectrophotometer (England, UK). The size distribution of AuNFs before/after surface modification was obtained on a Malvern Zetasizer Nano ZS90 dynamic light scattering (DLS) system (Malvern Instruments Ltd, Worcestershire, UK). The TRL signals from the Eu3+ were determined (excitation at 334 nm, emission at 620 nm) on a Multiskan GO multimode reader (Thermo Varioskan Flash, Thermo, USA). Double-distilled water was prepared on a Milli-Q system (Millipore, Milford, MA, USA).
2.3 Synthesis of AuNFs
AuNFs with a size of 80 nm were synthesized using the seed growth method as described earlier with a slight modification.20 The gold seed solution (18 nm) was initially prepared via the classical trisodium citrate reduction method. After the seed synthesis, AuNFs were prepared in accordance with a slightly modified seeding growth method.21 In a typical synthesis procedure, 1.2 mL of 1% (w/v) HAuCl4 solution and 2.64 mL of 1% (w/v) trisodium citrate solution were added into 100 mL of double-distilled water containing 1.78 mL of gold seed solution at 50 °C. Subsequently, 24 mL of 30 mM hydroquinone solution was quickly added into the mixture under vigorous stirring. The color of the reaction solution instantaneously changed to deep blue, and then the resulting AuNF solution was cooled to ambient temperature. Finally, the obtained AuNF solution was stored at 4 °C for further use.
2.4 Surface modification of AuNFs with thiol-EDTA and thiol-NHNH2
The thiol-EDTA ligand was initially synthesized via a similar method described by Rotello et al.,22,23 and the detailed synthesis and characterization are presented in the ESI.† The thiol-NHNH2 ligand was provided by our laboratory and used for the oriented coupling of mAbs.24 Then, the AuNFs co-modified by thiol-EDTA and thiol-NHNH2 ligands (thiol-NHNH2@AuNFs@thiol-EDTA) were synthesized via the ligand exchange method, as previously described.19,25 Moreover, 10 mL of AuNFs (16.07 pM) was centrifuged and re-suspended with 1 mL of alkaline double-distilled water (pH 9.0). Then, 5 μL of TCEP solution (4 mg mL−1) was added into a 20 μmol mixed solution of thiol-EDTA (1 μmol) and thiol-NHNH2 (0.01 μmol) for 15 min reaction at room temperature. Afterward, the reacted mixture solution was dropwise added into the concentrated AuNF solution. After stirring at room temperature overnight, the mixture solution was centrifuged at 3000 rpm for 10 min, and the thiol-NHNH2@AuNFs@thiol-EDTA precipitate was re-dissolved with alkaline double-distilled water (pH 9.0).
2.5 Synthesis of dAbs-thiol-NHNH2@AuNFs@thiol-EDTA-Eu3+ probes
The dAbs-thiol-NHNH2@AuNFs@thiol-EDTA-Eu3+ probes, abbreviated as AuNF probes, were prepared by adding 50 μL of Eu3+ solution (100 μM) into 500 μL of thiol-NHNH2@AuNFs@thiol-EDTA solution (160.7 pM). After stirring for 4 h at room temperature, the precipitate was obtained with centrifugation at 3000 rpm for 10 min and then re-suspended in phosphatic buffer (PB, pH 6.0). Then, 12 μL of dAbs (1 mg mL−1) was dropwise added into the abovementioned resuspension solution. After incubation for 2 h under stirring, the resultant probe solution was centrifuged at 3000 rpm for 10 min, and then the precipitate was re-dissolved in PB solution (pH 7.4).
2.6 Preparation of cAbs-modified MBs (MBs@cAbs)
The conjugation of carboxylated MBs with cAbs against C. muytjensii ATCC 51329 was performed on the basis of the formation of amido bonds between the carboxyl group of MBs and the amino group of cAbs in the presence of EDC.26 In a typical synthesis procedure, 6 μL of cAbs solution (1 mg mL−1) was added into 0.4 mL of carboxylated MB solution (2.4 pM). After incubation for 30 min under gentle stirring, 50 μL of EDC solution (1 mg mL−1) was added into the abovementioned mixed solution. After incubation for another 30 min, 100 μL of BSA solution (50 mg mL−1) was added to block the remaining active carboxylic groups for removing the possible nonspecific absorption. The resultant MBs@cAbs were collected using an external magnetic field, washed three times with PB solution, and re-suspended in 2 mL of PB solution. The resuspension solution was stored at 4 °C for further use.
2.7 Procedure for the ultrasensitive luminescence detection of C. muytjensii
As illustrated in Scheme 1, a magnet-mediated double-antibody sandwich-type immunoassay was completed for the ultrasensitive luminescence detection of C. muytjensii. The detection details were as follows: 100 μL of MBs@cAbs (1.2 pM) was added and mixed into 500 μL of the sample solution with a series of desirable concentrations of C. muytjensii over the range of 0–4.1 × 108 cfu mL−1, and the mixture solution was incubated for 30 min at room temperature under gentle stirring. The formed MBs@cAbs-C. muytjensii complexes were washed three times with PB solution (pH 7.4) under an external magnetic field and then re-suspended in 200 μL of the prepared AuNF probes (16 pM). After reaction for 40 min, the formed sandwiched MBs@cAbs-C. muytjensii-AuNF complexes were collected using an external magnetic field. Then, 50 μL of the supernatant solution containing the unbound AuNF probes was pipetted into each well of a 96-well black microplate. Subsequently, 200 μL of the enhancer solution was added into each well. After 20 min, the microplate was subjected to TRL detection on a Multiskan GO multimode reader with excitation and emission wavelengths at 334 and 620 nm, respectively.
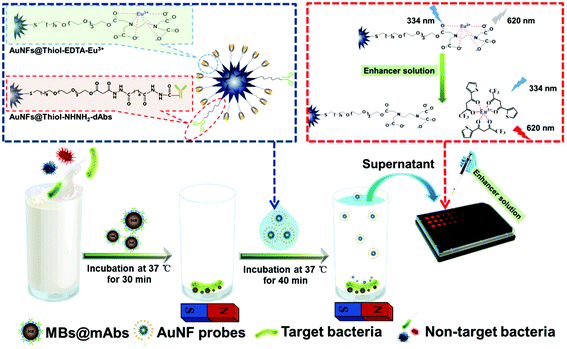 |
| Scheme 1 Schematic illustration of the gold nanocarrier amplified DELFIA method for C. muytjensii detection in powdered infant formula. | |
3 Results and discussion
3.1 Synthesis and characterization of thiol-NHNH2@AuNFs@thiol-EDTA
The TEM image in the inset of Fig. 1A displays that the synthesised AuNFs showed a typical flowerlike multibranched structure with a size of 80 ± 2 nm (n = 100), which was further confirmed by DLS measurement (Fig. 1B). Fig. 1A presents the maximum absorption peak of the prepared AuNFs centered at 651 nm. After ligand modification, the formed thiol-NHNH2@AuNFs@thiol-EDTA under different ligand feeding ratios of thiol-EDTA/thiol-NHNH2 ranging from 30
:
1 to 400
:
1 showed a slight red shift of 3–4 nm compared with the initial AuNFs. Furthermore, DLS analysis indicated that all AuNF conjugates exhibited increased average hydrodynamic diameters (Fig. 1B). Fig. 1C shows that the zeta potential of AuNFs showed a decreasing trend with the change in ligand feeding ratios from 0 (without ligand modification) to 400
:
1, whereas the thiol-EDTA-modified AuNFs displayed the lowest zeta potential. The possible reason for the reduced zeta potential is that each thiol-EDTA ligand contains several electronegative carboxyl groups. These results suggest the successful conjugation of the thiol-EDTA and thiol-NHNH2 ligands on the AuNF surface through the Au–S bond, which is suitable as an enhanced signal carrier for increasing the Eu3+ loading and oriented coupling of mAbs to magnify the TRL signal significantly and thus improve the detection sensitivity.
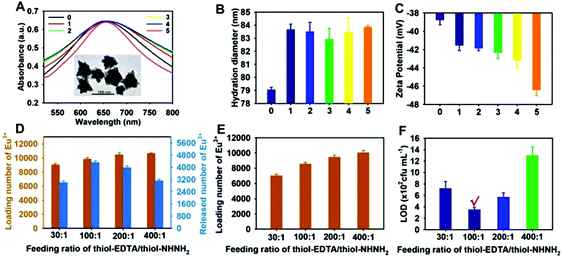 |
| Fig. 1 Characterization of AuNFs before and after ligand modification at different thiol-EDTA/thiol-NHNH2 ratios of 0 (0), 30 : 1 (1), 100 : 1 (2), 200 : 1 (3), 400 : 1 (4), and all thiol-EDTA (5). (A) UV-visible spectra; (B) hydrodynamic diameter; (C) zeta potential. Characterization of the loading and release amounts of Eu3+ per AuNF and the detection sensitivity at different ligand ratios of 30 : 1 to 400 : 1. (D) Luminescence analysis of Eu3+ in the supernatant after AuNF chelation. (E) ICP-MS for the AuNF-Eu3+ chelate. (F) Effect of the detection of limit (LOD) using different AuNF probes. Here, “√” indicates the optimal selection. | |
To investigate the effect of the feeding ratio of thiol-EDTA and thiol-NHNH2 on the loading capacity of Eu3+ ions, 500 μL of thiol-NHNH2@AuNFs@thiol-EDTA conjugates (160.7 pM) under different feeding ratios was incubated with 50 μL of Eu3+ solution (100 μM). After centrifugation, the excess Eu3+ ions were discarded, and the formed thiol-NHNH2@AuNFs@thiol-EDTA-Eu3+ complex was subjected to an optimized enhancer solution (pH 3.0), consisting of Triton X-100 (0.05 μl L−1), TTA (7.5 μg mL−1), and TOPO (20 μg mL−1), as shown in Fig. S1.† Then, the loading and release amounts of Eu3+ ions from AuNFs were determined on the basis of the Eu3+-dependent TRL signal variation (Fig. S2†). The results in Fig. 1D showcase that with the feeding ratios of thiol-EDTA and thiol-NHNH2 varying from 30
:
1 to 400
:
1, the loading Eu3+ amount per AuNF slightly increased from 9028 ± 190 to 10
651 ± 78. Thus, excess thiol-EDTA ligands cannot greatly increase the loading Eu3+ amounts. By contrast, the released Eu3+ amount per AuNF initially increased from 3003 ± 113 to 3329 ± 92 with the change in feeding ratios from 30
:
1 to 100
:
1 and then gradually decreased to 2125 ± 85 with the feeding ratios continuously varying to 400
:
1. The inductively coupled plasma mass spectrometry (ICP-MS) measurement further confirmed the loading amounts of Eu3+ ions per AuNF (Fig. 1E), which were consistent with the results obtained using the TRL analysis. The corresponding release efficiencies of Eu3+ ions from the AuNF surface were calculated as 33.26% for 30
:
1, 36.87% for 100
:
1, 24.73% for 200
:
1, and 19.95% for 400
:
1. Several possible reasons for these phenomena are as follows: (1) when the amount of the thiol-EDTA ligand on the AuNF surface is relatively low (30
:
1), the Eu3+ loading per AuNF will reduce, resulting in a low Eu3+ release. (2) Excessive EDTA amounts can lead to the stronger competitive binding of EDTA with Eu3+ ions than TTA, thereby limiting the Eu3+ release. To obtain the optimal ligand-modified AuNF as an enhanced carrier for amplified luminescence detection, all resultant ligand-modified AuNFs were functionalized with a specific antibody against C. muytjensii ATCC 51329. They were also used as the detector probes to build a double-antibody sandwich-type immunoassay for analyzing the influence of the feeding ratio of thiol-EDTA and thiol-NHNH2 on the detection sensitivity. The results in Fig. 1F show that the highest detection sensitivity with a LOD of 3.5 × 102 cfu mL−1 was obtained when the feeding ratio of thiol-EDTA/thiol-NHNH2 was set at 100
:
1. Therefore, this feeding ratio was selected for the optimized ligand modification on the AuNF surface for further experiments. In addition, considering that the AuNF probe stability determines the reproducibility of the detection results, we further performed the time-dependent dynamic monitoring of the luminescence intensity of the prepared AuNF probes for evaluating their long-term storage stability. Fig. S3† shows no evident changes in the luminescence intensity at 620 nm at the C. muytjensii concentration of 4.1 × 104 cfu mL−1 even after storage for 15 days. Thus, the designed AuNF probes exhibit excellent long-term storage stability in PB with a pH of 7.4.
3.2 Optimization of experimental parameters
The assay development and optimization involved the following several factors that can affect the luminescence intensity at 620 nm (LI620) and the detection sensitivity: the saturated labeling dosage of cAbs on the MB surface, the used amount of MBs@cAbs, the used concentration of AuNF probes, the incubation time for the binding of MBs@cAbs with C. muytjensii, the incubation time for the binding of AuNF probes with the MBs@cAbs-C. muytjensii complex, the magnetic separation time for the MB recovery, and the signal reading time after the addition of the luminescence enhancer solution. Fig. 2A–G show the detailed optimization results for these parameters. As shown in Fig. 2A–F, the largest luminescence intensity changes of ΔLI620 to enable the best detection sensitivity were achieved with the optimized combinations of the amount of labeled cAbs at 6 μg per 400 μL of MBs (2.4 pM, Fig. 2A), the amount of MBs@cAbs at 1.4 pM (Fig. 2B), the concentration of AuNF probes at 16 pM (Fig. 2C), the incubation time for the binding of MBs@cAbs with C. muytjensii at 30 min (Fig. 2D), the incubation time for the binding of AuNF probes at 40 min (Fig. 2E), and the magnetic separation time for the MB separation at 6 min (Fig. 2F). Moreover, Fig. S4† shows the strict time-dependent dissolution-enhanced luminescence phenomenon, in which the LI620 gradually augmented with the increase in the incubation time of the luminescence enhancer solution with AuNF probes from 10 min to 20 min and then reached a balance with the incubation time continuously prolonging to 22 min. Thus, 20 min for conducting the dissolution-enhanced luminescence was necessary to realize a reliable, stable, and reproduce signal output.
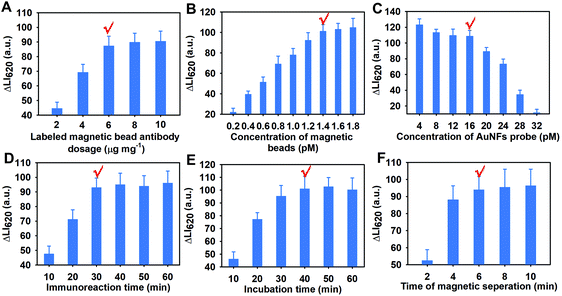 |
| Fig. 2 Optimization of various experimental parameters. (A) The saturated labeling dosage of cAbs on the MB surface; (B) the used amount of MBs@cAbs; (C) the used concentration of AuNF probes; (D) the incubation time for the binding of MBs@cAbs with C. muytjensii; (E) the incubation time for the binding of AuNF probes; (F) the magnetic separation time; each value represents the mean of five independent experiments (n = 5). Here, “√” indicates the optimal selection. | |
3.3 Analytical performances of the AuNF-amplified DELFIA method
Under the developed conditions, we utilized the AuNF-amplified luminescent nanoprobes in a sandwich-type DELFIA for the ultrasensitive detection of C. muytjensii. Scheme 1 presents the detection principle. The detection response and dynamic linear range of our method were systematically evaluated by analyzing a range of standard solutions with the target concentration of 0–4.1 × 107 cfu mL−1. Fig. 3A shows that the red luminescence in the supernatant gradually weakened as the concentration of C. muytjensii increased. By recording the corresponding LI620, Fig. 3B presents target concentration dependent TRL signal changes (ΔLI620), where the ΔLI620 value for the C. muytjensii detection gradually increased with the increase in C. muytjensii concentration. Moreover, an excellent linear correlation of ΔLI620versus C. muytjensii concentration ranged from 4.1 × 102 cfu mL−1 to 4.1 × 106 cfu mL−1 with a correlation coefficient of 0.9962 (inset of Fig. 3B). The LOD, defined as the concentration that corresponds to the mean plus the threefold standard deviation from the blank sample, was calculated to be 1.2 × 102 cfu mL−1, which is approximately 230-fold lower than that of conventional HRP-based ELISA (2.6 × 104 cfu mL−1, Fig. S5†). Table S2† indicates that the LOD value of our AuNF-amplified DELFIA method was comparable with or better than those of other reported immunoassay-based methods for Cronobacter detection.
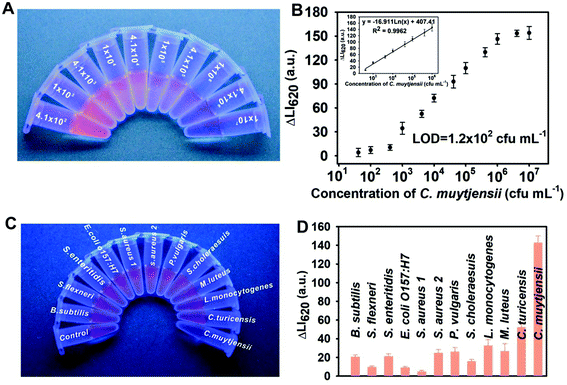 |
| Fig. 3 Quantitative detection of C. muytjensii using the proposed method. (A) Photograph of fluorescence signal changes under different concentrations of C. muytjensii. (B) The relationship between the ΔLI620 and C. muytjensii concentrations. The inset indicated a standard curve for C. muytjensii detection with the concentration ranging from 4.1 × 102 to 4.1 × 106 cfu mL−1. (C) Photograph of fluorescence signal changes for specificity evaluation with C. muytjensii (4.1 × 106) over other nontarget bacteria. (D) The corresponding ΔLI620 value from C. Each value represents the mean of three independent experiments (n = 3). | |
To evaluate the specificity of the proposed method, we applied it to detect eleven other common pathogenic bacteria, namely, one Cronobacter turicensis strain, two Salmonella strains, one Escherichia coli O157:H7 strain, one Proteus vulgaris strain, one Shigella flexneri strain, one Listeria monocytogenes strain, one Micrococcus luteus strain, one Bacillus subtilis strain, and two Staphylococcus strains. Meanwhile, one negative control was performed only using sterile PBS solution, whereas one positive control was carried out using sterile PBS solution containing C. muytjensii (4.1 × 106 cfu mL−1). The results in Fig. 3C showed a significant reduction in the red luminescence when C. muytjensii is present in the sample solution, whereas distinct red luminescence was observed in testing other nontarget bacteria. These results were further confirmed via TRL spectrum analysis based on the luminescence intensity change at 620 nm (ΔLI620). As shown in Fig. 3D, compared with the negative control, a markedly increased ΔLI620 value appeared in the presence of C. muytjensii, whereas negligible changes in ΔLI620 for other nontarget bacteria were observed even at a high bacterial concentration of 107 cfu mL−1. These phenomena suggest the high selectivity of our proposed method against C. muytjensii.
To estimate the precision and accuracy of this amplified DELFIA method, the addition and recovery experiments for the intra- and inter-assays were implemented by analyzing four C. muytjensii-spiked PIF samples with different concentrations at 1 × 103, 1 × 104, 1 × 105, and 1 × 106 cfu mL−1. Table 1 shows that the average recovery rates for the intra-assay ranged from 92.3% to 116.8% with the coefficient of variation (CV) of 4.4%–7.3%, and those for the inter-assay varied from 93.1% to 115.2% with the CV of 4.8%–6.0%. These results indicate that the amplified DELFIA method provides an acceptable accuracy for the quantitative detection of C. muytjensii in real PIF samples. To investigate the practicability of the current method further, 18 randomly spiked PIF samples with a C. muytjensii concentration of 0–5 × 106 cfu mL−1 were determined using the proposed method. The results in Table 2 show that although the concentrations of C. muytjensii in PIF samples varied randomly, the recovery rates were maintained above 80% with the CV below 14%. The C. muytjensii-negative samples were found to be negative. These findings indicate the feasibility of our designed amplified DELFIA approach for the rapid, sensitive, and accurate screening of C. muytjensii contaminated in PIF samples.
Table 1 Recovery and precision of the proposed DELFIA in C. muytjensii ATCC 51329-spiked milk samples (n = 3)
C. muytjensii added (cfu mL−1) |
Intra-assay precisiona |
Inter-assay precisionb |
Mean of C. muytjensii detected (cfu mL−1) |
Recovery (%) |
CV (%) |
Mean of C. muytjensii detected (cfu mL−1) |
Recovery (%) |
CV (%) |
The assay was carried out in quadruplicate on the same day.
The assay was performed on three consecutive days.
|
1000 |
929 |
92.90 |
7.32 |
931 |
93.10 |
5.39 |
10 000 |
10 821 |
108.21 |
6.51 |
98 977 |
98.98 |
6.00 |
100 000 |
116 791 |
116.79 |
6.74 |
115 185 |
115.19 |
8.83 |
1 000 000 |
922 686 |
92.27 |
4.37 |
1 029 756 |
102.98 |
4.76 |
Table 2 Detection of C. muytjensii ATCC 51329 in PIF samples using the proposed method (n = 3)
Sample no. |
Spiked (cfu mL−1) |
Detected (cfu mL−1) |
Recovery (%) |
CV (%) |
ND is representative for not detected. |
1 |
0 |
ND |
— |
— |
2 |
0 |
ND |
— |
— |
3 |
0 |
ND |
— |
— |
4 |
200 |
169 |
84.53 |
10.61 |
5 |
200 |
194 |
97.02 |
8.44 |
6 |
200 |
187 |
93.51 |
9.24 |
7 |
1000 |
1132 |
113.17 |
6.87 |
8 |
1000 |
953 |
95.33 |
8.70 |
9 |
1000 |
825 |
82.54 |
11.47 |
10 |
30 000 |
26 778 |
89.29 |
9.84 |
11 |
30 000 |
30 516 |
101.65 |
6.62 |
12 |
30 000 |
25 753 |
85.81 |
10.37 |
13 |
400 000 |
426 071 |
106.50 |
7.41 |
14 |
400 000 |
438 923 |
109.67 |
11.30 |
15 |
400 000 |
395 836 |
99.04 |
7.72 |
16 |
5 000 000 |
4 168 293 |
83.43 |
9.43 |
17 |
5 000 000 |
4 973 590 |
99.52 |
10.15 |
18 |
5 000 000 |
5 644 871 |
112.87 |
13.80 |
4 Conclusion
We successfully developed a novel ultrasensitive luminescence immunoassay by using amphiphilic ligand-modified AuNFs as enhanced lanthanide nanocarriers for increasing the Eu3+ labeling ratio. Given the ultrahigh loading amount of Eu3+ ions in a single AuNF, numerous Eu3+ ions from the AuNF surface were released and transformed into highly luminescent Eu3+ micelles after the addition of the acid enhancer solution. This approach significantly amplified the TRL signal and thus enhanced the detection sensitivity of the conventional DELFIA method. Our amplified AuNF nanoprobes achieved an accurately quantitative assay of C. muytjensii in a highly sensitive manner by integrating a magnetic-mediated sandwich-type DELFIA method. The LOD of this method was as low as 1.2 × 102 cfu mL−1, which is approximately 230-fold lower than that of the conventional colorimetric immunoassay. Moreover, we demonstrated that our proposed method displayed excellent selectivity, high reproducibility, and strong practicality in the spiked PIF sample. These findings indicated that our developed AuNF-mediated nanocarrier signal amplification strategy can serve as a powerful tool to improve the labeling ratio of the Ln3+ chelate for markedly enhancing the TRL signal and can open a new direction for fabricating various ultrasensitive luminescence immunoassays.
Conflicts of interest
There are no conflicts of interest.
Acknowledgements
This work was supported by a grant from the National Key Research and Development Program of China (2018YFC1602202 and 2018YFC1602505), the National Natural Science Foundation of China (31760485), the Interdisciplinary Innovation Fund of Natural Science, Nanchang University (9166-27060003-ZD01), and the Opening Fund of Jiangsu Key Laboratory for Food Quality and Safety-State Key Laboratory Cultivation Base, Ministry of Science and Technology (028074911709).
Notes and references
- G. D. Grothaus, M. Bandla, T. Currier, R. Giroux, G. R. Jenkins, M. Lipp, G. Shan, J. W. Stave and V. Pantella, J. AOAC Int., 2006, 89, 913–928 CAS.
- T. R. Holford, F. Davis and S. P. Higson, Biosens. Bioelectron., 2012, 34, 12–24 CrossRef CAS.
- X. Huang, R. Chen, H. Xu, W. Lai and Y. Xiong, Anal. Chem., 2016, 88, 1951–1958 CrossRef CAS.
- D. A. Tomalia, B. Klajnert-Maculewicz, K. A. M. Johnson, H. F. Brinkman, A. Janaszewska and D. M. Hedstrand, Prog. Polym. Sci., 2019, 90, 35–117 CrossRef CAS.
- H. Chen, L. Lin, H. Li, J. Li and J.-M. Lin, ACS Nano, 2015, 9, 2173–2183 CrossRef CAS.
- W. Xu, G. Zou, H. Hou and X. Ji, Small, 2019, 15, e1804908 CrossRef.
- X. Huang, Z. Xu, Y. Mao, Y. Ji, H. Xu, Y. Xiong and Y. Li, Biosens. Bioelectron., 2015, 66, 184–190 CrossRef CAS PubMed.
- X. Huang, Y. Liu, B. Yung, Y. Xiong and X. Chen, ACS Nano, 2017, 11, 5238–5292 CrossRef CAS.
- D. Kim, J. Kim and T. S. Lee, Sens. Actuators, B, 2018, 264, 45–51 CrossRef CAS.
- D. Liu, Z. Wang and X. Jiang, Nanoscale, 2011, 3, 1421–1433 RSC.
- H. Siitari, I. Hemmilä, E. Soini, T. Lövgren and V. Koistinen, Nature, 1983, 301, 258 CrossRef CAS.
- I. Hemmilä, S. Dakubu, V.-M. Mukkala, H. Siitari and T. Lövgren, Anal. Biochem., 1984, 137, 335–343 CrossRef.
- S. Zhou, W. Zheng, Z. Chen, D. Tu, Y. Liu, E. Ma, R. Li, H. Zhu, M. Huang and X. Chen, Angew. Chem., 2014, 126, 12706–12710 CrossRef.
- E. P. Diamandis, Clin. Biochem., 1988, 21, 139–150 CrossRef CAS.
- Z. Chen, W. Zheng, P. Huang, D. Tu, S. Zhou, M. Huang and X. Chen, Nanoscale, 2015, 7, 4274–4290 RSC.
- J. Xu, S. Zhou, D. Tu, W. Zheng, P. Huang, R. Li, Z. Chen, M. Huang and X. Chen, Chem. Sci., 2016, 7, 2572–2578 RSC.
- Z. Li and Y. Zhang, Nanotechnology, 2008, 19, 345606 CrossRef.
- A. Bordat, T. Boissenot, J. Nicolas and N. Tsapis, Adv. Drug Delivery Rev., 2019, 138, 167–192 CrossRef CAS.
- Y. Zhou, X. Huang, W. Zhang, Y. Ji, R. Chen and Y. Xiong, Biosens. Bioelectron., 2018, 102, 9–16 CrossRef CAS.
- P. Xu, J. Li, X. Huang, H. Duan, Y. Ji and Y. Xiong, Anal. Methods, 2016, 8, 3316–3324 RSC.
- J. Li, J. Wu, X. Zhang, Y. Liu, D. Zhou, H. Sun, H. Zhang and B. Yang, J. Phys. Chem. C, 2011, 115, 3630–3637 CrossRef CAS.
- S. C. Hayden, G. Zhao, K. Saha, R. L. Phillips, X. Li, O. R. Miranda, V. M. Rotello, M. A. El-Sayed, I. Schmidt-Krey and U. H. Bunz, J. Am. Chem. Soc., 2012, 134, 6920–6923 CrossRef CAS.
- Z. Jiang, N. D. B. Le, A. Gupta and V. M. Rotello, Chem. Soc. Rev., 2015, 44, 4264–4274 RSC.
- S. Kumar, J. Aaron and K. Sokolov, Nat. Protoc., 2008, 3, 314–320 CrossRef CAS.
- A. M. Smith, L. E. Marbella, K. A. Johnston, M. J. Hartmann, S. E. Crawford, L. M. Kozycz, D. S. Seferos and J. E. Millstone, Anal. Chem., 2015, 87, 2771–2778 CrossRef CAS.
- D. Lou, L. Fan, Y. Cui, Y. Zhu, N. Gu and Y. Zhang, Anal. Chem., 2018, 90, 6502–6508 CrossRef CAS.
Footnote |
† Electronic supplementary information (ESI) available. See DOI: 10.1039/c9an01945f |
|
This journal is © The Royal Society of Chemistry 2020 |
Click here to see how this site uses Cookies. View our privacy policy here.