DOI:
10.1039/C9BM00905A
(Paper)
Biomater. Sci., 2020,
8, 256-265
Programmed delivery of cyclopeptide RA-V and antisense oligonucleotides for combination therapy on hypoxic tumors and for therapeutic self-monitoring†
Received
11th June 2019
, Accepted 11th October 2019
First published on 16th October 2019
Abstract
Chemotherapy is a dominant treatment modality for different types and stages of cancer. However, hypoxia is one of the undesirable limitations of chemotherapy, which reduces the therapeutic efficiency in cancer treatment, ultimately leading to failure of the treatment. Herein, an ideal chemosensitization system capable of attenuating the tumor hypoxia microenvironment and enhancing chemotherapy effects in tumors was designed. This system (designated as the RA/RX Liposome) uses for the first time a pH-sensitive liposome to co-deliver cyclopeptide RA-V as chemotherapeutic drugs and antisense oligonucleotides as HIF-1α inhibitors (RX-0047) for attenuating tumor hypoxia, as well as a caspase-8 activation probe for therapeutic self-monitoring. After modification with death receptor 5-specific antibodies (anti-DR5) on the surface of the liposome, the RA/RX Liposome can successfully deliver components targeting colon tumors in vivo. This work should synergistically enhance the therapeutic effects of the treatment by successfully down-regulating HIF-1α expression against tumor hypoxia during the RA-V-induced apoptotic process. More importantly, the RA/RX Liposome can be precisely applied for therapeutic self-monitoring with the light-up fluorescence of the caspase-8 probe.
Introduction
Cancer has become one of the most serious public health problems, causing unnecessary deaths throughout the world. Among the various cancers, colon cancer is the third leading cause of cancer death in the United States, with over 20 million new cases estimated in 2016.1–3 Chemotherapy plays an essential and significant role in fighting colon cancer. For example, fluorouracil (FU)-based chemotherapy has been confirmed to be effective in reducing tumor recurrence.4 However, hypoxia, as a typical feature of tumor treatment, has been found to hinder treatment effectiveness in most of the therapeutic protocols.5 It has been reported that hypoxia can result in the failure of treatment due to the molecular changes in hypoxic tumors, such as the abnormal activation of oxygen homeostasis associated proteins and changes in metabolic pathways for the prevention of apoptosis.6 For example, hypoxia-inducible factor 1α (HIF-1α), used as an important marker, is involved in related processes. HIF-1α transactivates more than 100 hypoxia-inducible genes, including genes involved in tumor angiogenesis, metastasis, proliferation, and altered energy metabolism.7,8 A number of studies have shown that inhibition of HIF-1α expression can enhance anti-tumor effects.9–15 Recently, combination therapy, which integrates multiple therapeutic modalities, have shown great potential as a highly efficient treatment of cancer.16–20 Therefore, constructing combination therapy systems which successfully combine anti-tumor drugs and the HIF-1α inhibitor will be very useful for improving therapy efficacy in hypoxic tumors.
To date, traditional chemotherapeutic drugs used for combination therapy still have many drawbacks, such as poor targeting properties, low local tumor accumulation and poor pharmacokinetics.21–23 Thus, there is an urgent need for new types of chemotherapy drugs which do not have these properties. Cyclopeptide RA-V (deoxybouvardin), derived from the medicinal plant Rubia yunnanensis,24–26 has attracted much attention from researchers because of its unique chemical structure and excellent anti-tumor efficacy. RA-V has been reported to exhibit anti-tumor activities in vitro and in tumor-bearing BALB/c nude mice, and could suppress inflammation, angiogenesis, and protective tumor cell autophagy and induce apoptosis.27–33 Our previous investigation has established that RA-V exhibits a remarkable antitumor activity in colon cancer cells.34 In our latest study, it was found that RA-V could block the interaction of transforming growth factor-β-activated kinase 1 (TAK1)35 with TAK1 binding protein 2 (TAB2),36 which contributes to tumor cell apoptosis by inhibiting the NF-κB signaling pathway.37 It has been reported that the inhibition of the NF-κB pathway could lead to a decrease in HIF-1α expression.38 So there may be some intrinsic relationship between the anticancer mechanism of RA-V and hypoxia. Thus, RA-V is a promising anticancer agent for constructing a combination therapy system together with the HIF-1α inhibitor for synergistic therapy in hypoxic tumors.
We report herein the development of a combination therapy nanosystem (the RA/RX Liposome) for highly effective therapy against a hypoxic tumor and real-time imaging of caspase-8 activation in the apoptosis process (Scheme 1). In the system, cyclopeptide RA-V in the pH-sensitive shell of an RA/RX Liposome can induce cancer cell apoptosis via mitochondrial pathways and the antisense oligonucleotide in the core of the RA/RX Liposome can inhibit HIF-1α expression simultaneously. To further improve colon cancer selectivity, anti-DR5,39–41 which selectively recognizes the pro-apoptotic cell surface receptor on colorectal tumour cells, was conjugated to the surface of liposomes. The obtained RA/RX Liposome could selectively enter colon cancer cells via receptor-mediated endocytosis into the lysosome, in which the acidic microenvironment stimulated the liposomes to release the payloads. More significantly, the caspase-8 specific substrate peptide (IETD) labeled with Cy5.5 fluorescein and dark quenchers (BHQ-3) (designated as the caspase-8 probe) was also incorporated into the RA/RX Liposome to provide a real-time monitoring of the activation of caspase-8. Since caspase-8 is the chief initiator that participates in the downstream hallmarks of apoptosis,42–45 the assessment of caspase-8 activity can be a useful prognosis modality in therapeutic self-monitoring. To the best of our knowledge, this is the first time that a programmed delivery system has been reported which integrates an anticancer agent and the HIF-1α inhibitor to enhance chemotherapy against colon tumors, as well as to achieve precise therapeutic monitoring with the light-up fluorescence of the caspase-8 probe.
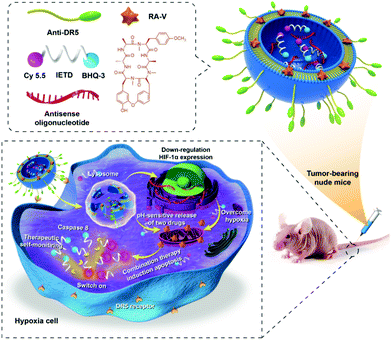 |
| Scheme 1 Schematic illustration of co-delivery cyclopeptide RA-V and antisense oligonucleotide with therapeutic self-monitoring in colon cancer. | |
Results and discussion
Characterization of the RA/RX Liposome and in vitro cyclopeptide RA-V release profiles
The liposome was fabricated using a thin film hydration method.29,46 Antisense oligonucleotide against HIF-1α (RX-0047) and the caspase-8 probe were incorporated into the aqueous core using a self-assembly process and cyclopeptide RA-V was doped in the liposomal shell utilizing hydrophobicity. The RA/RX Liposome was further modified with anti-DR5 for cell selectivity on the surface of the liposome. The encapsulation efficiencies of cyclopeptide RA-V, RX-0047 and caspase-8 probe in the liposome were found to be 62.7%, 24.7% and 25.5%, respectively, using High Performance Liquid Chromatography (HPLC). As shown in Fig. 1a, transmission electron microscopy (TEM) showed that the liposomes with a spherical morphology were averagely dispersed and degraded in an acidic environment (Fig. S1†). The average hydrodynamic diameter of the RA/RX Liposome was determined to be 173.6 ± 1.41 nm (Fig. 1b) with Malvern Instruments, and the diameter decreased when exposed to acidic pH (Fig. S2†). We later measured the apparent zeta potential of the RA/RX Liposome and the RA/RX Liposome (without anti-DR5), which is used to determine the nanomaterial stability in aqueous solution,46 demonstrating that the RA/RX Liposome changed from −44.2 ± 0.1 mV (Fig. S3†) to −29.83 ± 0.75 mV (Fig. S4†) after modification with anti-DR5. The change in the apparent zeta potential qualitatively reflected the successful modification of anti-DR5 on the surface of the RA/RX Liposome because of the positive charge from the peptide.
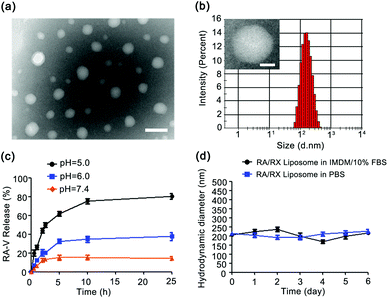 |
| Fig. 1 (a) TEM micrographs of the RA/RX Liposome. Scale bar: 200 nm. (b) Size distribution of nanoparticles characterized using Malvern Instruments at 25 °C. Insets: TEM micrograph of the RA/RX Liposome. Scale bars: 50 nm. (c) In vitro release profiles of RA-V from the RA/RX Liposome at pH 5.0, 6.0 and 7.4. Data are presented as mean ± SD (n = 3). (d) Long-term-stability study of the RA/RX Liposome in IMDM medium with 10% FBS or phosphate buffer saline (PBS). Data are presented as mean ± SD (n = 3). | |
The pH-mediated release of RA-V from the pH-sensitive liposome was investigated using HPLC under different conditions (Fig. 1c). At pH 7.4, no more than 20% of RA-V was released over 25 h incubation, reflecting no significant pH response to a normal physiological environment. However, the release of RA-V was apparently accelerated at pH 6.0, reaching a plateau after about 10 h incubation. 38.28 ± 1.02% of RA-V was released from the RA/RX Liposome after 25 h incubation under these conditions, which is largely dependent on the pH-induced degradation of DOPE. More significantly, the final accumulation of the released RA-V is 80.36 ± 0.75% at pH 5.0. Similar results were observed from the release profiles of RX-0047 and the caspase 8 probe at different pH values (Fig. S5 and S6†). In general, the release of RA-V from the RA/RX Liposome shows an obvious pH-dependent gradient in vitro. The pH-sensitivity of the RA/RX Liposome resulted from the strong tendency of the DOPE phospholipids to accumulate in inverse hexagonal phases instead of lamellar phases like in double layers. At physiological pH the negatively charged functional group of the stabilizing component (CHEMS) creates repulsive forces with the phosphate group of DOPE, which leads to the formation of a lamellar phase. A decrease in the pH induces the formation of the hexagonal phase and a subsequent collapse of the liposomes.47 Moreover, the stability of the RA/RX Liposome in IMDM medium with 10% FBS or phosphate buffer saline (PBS) was measured. As shown in Fig. 1d, the average hydrodynamic diameter of the RA/RX Liposome did not change significantly during 6 days, indicating that the liposomes were stable under conventional conditions. Therefore, the RA/RX Liposome is a smart drug delivery system that achieves a precisely triggered smart release in response to the acid micro-environment of the tumor.
Fluorescence response of the caspase-8 probe
The specific response of the caspase-8 probe was investigated by fluorescence spectral analysis (Fig. 2). As shown in Fig. 2a, on incubation of active caspase-8 with varying concentrations (0, 0.25 nM, 0.50 nM, 1.00 nM, 1.50 nM, 3.00 nM, and 6.00 nM), the fluorescence signals displayed a gradual increase, which shows a linear relationship between the maxima of fluorescence emission spectra and the concentrations (R2 = 0.9982). The results indicate that the caspase-8 probe has the ability to determine caspase-8 quantitatively. To further explore the specificity of the caspase-8 probe, the interference effect of other caspases and proteins was also investigated. As shown in Fig. 2b, the caspase-8 probe demonstrated high selectivity to caspase-8 compared with bovine serum albumin (BSA) or other incubators. In addition, the fluorescence intensity showed a negligible increase in the presence of the caspase-8 inhibitor (10 μM, Z-IETD-FMK42), confirming its specificity for the caspase-8 probe in response to caspase-8.
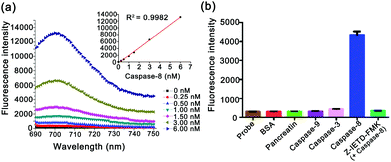 |
| Fig. 2 (a) Fluorescence emission spectra of the probe in the presence of various concentrations of caspase-8. Inset: Caspase-8 standard curve. (b) Fluorescence emission spectra of the probe incubated with bovine serum albumin (BSA) and different caspases (2 nM) for 1 h. | |
Cellular selectivity
For tracking the RA/RX Liposome in living colon cancer cells, 3,3′-dioctadecyloxacarbocyanineperchlorate (DiO), a lipophilic dye, was introduced into the liposome shells as a fluorescence tracker. When the RA/RX Liposome incubated with DR5 receptor overexpressed HCT116 cells, the fluorescence intensity in the cells increased gradually along with the incubation time and reached a maximum at 8 h (Fig. 3a). Quantitative analysis of the real-time confocal fluorescence images showed that the RA/RX Liposome was efficiently taken up or incorporated by the HCT116 cells (Fig. S7†). Moreover, the RA/RX Liposome was also incubated with NCM460 cells, a normal colon epithelial cell with low expression levels of DR5 receptor, demonstrating that only very weak fluorescence was observed in the NCM460 cells compared with the HCT116 cells. The distinct difference in the fluorescence between these two cell lines suggests that the targeting specificity of the RA/RX Liposome toward colon cancer significantly avoids the uptake by normal cells thus effecting the attenuation of side effects.
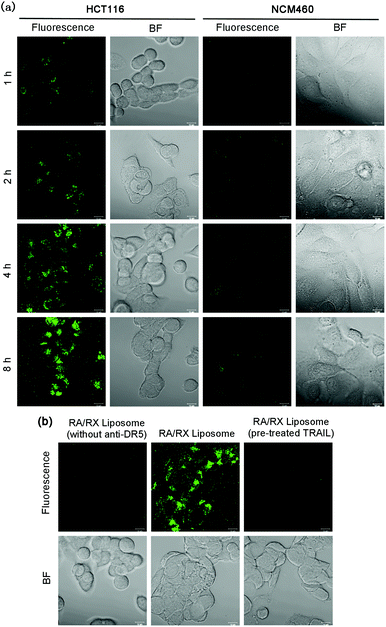 |
| Fig. 3 (a) Real-time confocal fluorescence images display the uptake of the RA/RX Liposome by HCT116 and NCM460 cells. Cells were incubated with the RA/RX Liposome for 1–8 h. Scale bars: 10 μm. (b) Confocal fluorescence imaging of HCT116 cells incubated with the RA/RX Liposome (without anti-DR5) or the RA/RX Liposome alone for 6 h; HCT116 cells pretreated with excessive free TRAIL, followed by incubation with the RA/RX Liposome for 6 h. Scale bars: 10 μm. | |
To further confirm that the selective recognition of anti-DR5 as a ligand to the DR5 receptor towards cancer cells played an indispensable role in cellular uptake, the RA/RX Liposome (without anti-DR5) was studied and compared with the RA/RX Liposome. As shown in Fig. 3b, the HCT116 cells displayed strong fluorescence within a 6 h incubation of the RA/RX Liposome, while the cells incubated with the RA/RX Liposome (without anti-DR5) showed a very weak fluorescence signal under similar experimental conditions. In addition, when the cells were treated with excessive free TRAIL,45 which could identify and bind to death receptors, the internalization of the RA/RX Liposome was blocked. Because there is no statistical difference of the DiO complexation efficiency in the RA/RX Liposome with (63.63 ± 2.05%) and without DR-5 (63.8 ± 1.73%), the higher intracellular fluorescence was due to the selective uptake of the RA/RX Liposome. Therefore, the targeting specificity of the RA/RX Liposome to the HCT116 cells was aided by the ligand–receptor of anti-DR5 in the RA/RX Liposome.
Colocalization assay
To identify the intracellular location of the RA/RX Liposome, the RA/RX Liposome-loaded HCT116 cells were costained with LysoTracker Red, Mito Tracker Red and Hoechst 33342, respectively. As shown in Fig. 4, the fluorescence of the RA/RX Liposome colocalized well with LysoTracker Red, and the colocalization efficiency was 0.98 ± 0.01; however, it did not match MitoTracker Red and Hoechst 33342. Similar results were observed from the same experiment which was performed with the RX-0047 Liposome alone, and the colocalization efficiency was 0.96 ± 0.02 (Fig. S8†). However, the colocalization efficiency of the RA/RX Liposome (without anti-DR5)-loaded HCT116 cells was 0.68 ± 0.03 (Fig. S9†). These results show that the RA/RX Liposome was apparently located in the lysosome, demonstrating that the liposomes entered the cancer cells through DR5 receptor-mediated endocytosis.
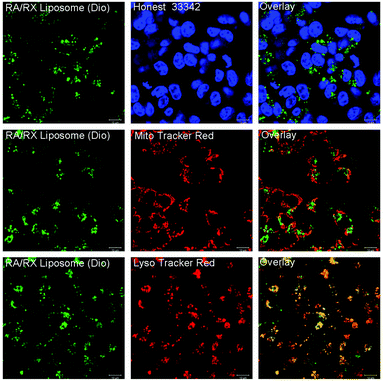 |
| Fig. 4 Colocalization images of the RA/RX Liposome in HCT116 cells. Cells were incubated with the RA/RX Liposome for 3 h and then incubated with 100 nM Hoechst 33342, MitoTracker Red, or LysoTracker Red for 10 minutes. Scale bars: 10 μm. | |
In vitro cytotoxicity of colon cancer cells
The anti-tumor activity of the RA/RX Liposome was first assessed by SRB cytotoxic assay in vitro. As shown in Fig. 5a, the cytotoxicity of the RA/RX Liposome in HT29 cells was obviously higher than those of Free RX-0047, RX-0047 Liposome, Free RA-V and RA-V Liposome. Therefore, it can be concluded that combining RA-V and RX-0047 into a liposome has an apparently synergistic therapeutic effect on colon cancer cells. Of greater significance, the RA/RX Liposome exerts distinct anti-tumor effects on HCT116 cells, which are KRAS-mutation colon cancer cells that are resistant to most of the chemotherapeutic drugs.48–50 As shown in Fig. 5b, the amount of the RA/RX Liposome (6.33 nM, RA-V equiv.) that led to 50% cell death was significantly lower than that required to achieve the same level of cell death with other treatments, suggesting that the programmed delivery system has remarkable therapeutic effects on KRAS-mutation colon cancer cells.
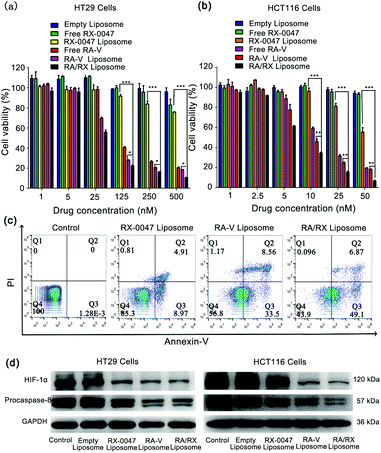 |
| Fig. 5 (a) SRB assay of HT29 cells in the presence of Empty Liposome, Free RX-0047, RX-0047 Liposome, Free RA-V, RA-V Liposome, RA/RX Liposome. (b) SRB assay of HCT116/mutated KRAS cells in the presence of Empty Liposome, Free RX-0047, RX-0047 Liposome, Free RA-V, RA-V Liposome, RA/RX Liposome. The results indicate that the combinations were effective with colon cancer cells. *P < 0.05, **P < 0.01, ***P < 0.001. (c) Flow cytometric analysis of HCT116 cell death using different treatments: cells without any treatment; cells treated with RX-0047 Liposome, RA-V Liposome and RA/RX Liposome. (d) Effects of Empty Liposome, RX-0047 Liposome, RA-V Liposome, RA/RX Liposome on the expression of HIF-1α and procaspase-8. The levels of protein HIF-1α and procaspase-8 were tested in total cell lysates from HT29 and HCT 116 cells. | |
To further confirm the ability of the RA/RX Liposome to promote colon cancer cell death through the apoptosis process, flow cytometry assays were used for the evaluation of the therapeutic effect of the RA/RX Liposome. As shown in Fig. 5c, the population of apoptotic cells treated with the RA/RX Liposome was much higher than that of the cells treated with other treatments. In addition, the results were further confirmed by JC-1 assay (Fig. S10†). All the results showed that the RA/RX Liposome could enhance the efficiency in cancer treatment by taking advantage of the synergistic effect between inducing cell apoptosis and overcoming hypoxia.
To further explore the mechanism of the RA/RX Liposome in inducing cancer cell apoptosis and the inhibition of HIF-1α, western blotting analysis was performed to quantify the related proteins including procaspase-8 and HIF-1α (Fig. 5d and Fig. S11 and S12†). Procaspase-8 plays a key role in the activation of caspase-8. Once procaspase-8 has been cleaved, the released activated caspase-8 induces cell apoptosis. Herein, there is a negative correlation between the level of procaspase-8 and the degree of activated caspase-8 in vitro. Quantitative analysis of the western blotting images showed that the level of procaspase-8 in HT29 and HCT116 cells treated with the RA/RX Liposome was lower than that with other treatments, which indicates that the RA/RX Liposome effectively promotes apoptosis through the activation of caspase-8. Furthermore, the level of HIF-1α protein in HT29 and HCT116 cells treated with the RA/RX Liposome was significantly reduced, which indicates that the RA/RX Liposome effectively inhibits the HIF-1α expression during the cell apoptosis process. Therefore, the RA/RX Liposome provides a new perspective in revealing the intrinsic relationship between RA-V-induction apoptosis and hypoxia environment in colon cancer.
Real-time visualization of caspase-8 cascade
Next, we investigated the potential application of the RA/RX Liposome in real-time monitoring of apoptosis in living colon cancer cells. Since the activation of caspase-8 is an early signal in the RA-V-induced mitochondrial apoptotic sequence pathway, real-time imaging of caspase-8 in situ could be very useful for self-monitoring the progression for use in therapy and exploring the exact anti-tumor mechanism of RA-V. As shown in Fig. 6, the HCT116 cells incubated with the RA/RX Liposome exhibited strong florescence signals in mitochondria, which demonstrated the activation of the caspase-8 probe in the RA/RX Liposome. On the other hand, when the HCT116 cells were pre-treated with Z-IETD-FMK (a caspase-8 inhibitor), the fluorescence signals drastically decreased, which confirmed that the “turn-on” fluorescence was caused by the RA/RX Liposome-mediated activation of caspase-8. Moreover, to demonstrate the utility of the RA/RX Liposome in dynamic therapeutic monitoring, HCT116 cells were incubated with the RA/RX Liposome for real-time imaging. As shown in Fig. 7, the fluorescence signals of the caspase-8 probe gradually increased along with the incubation time. The results show that the RA/RX Liposome can provide dynamic visualization of caspase-8 in situ, creating a convenient method for the therapeutic monitoring and investigation of the RA-V-induced mitochondrial apoptosis pathway.
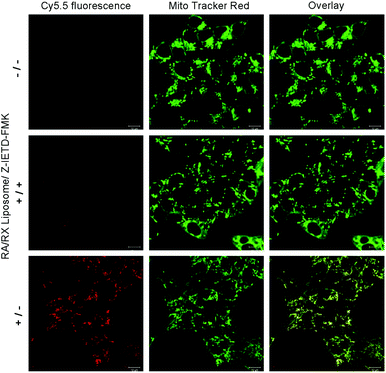 |
| Fig. 6 Confocal images of HCT116 cells incubated with RA/RX Liposome and caspase 8 inhibitors (Z-IETD-FMK, 50 mM). Red fluorescence (Cy5.5) indicates the fluorescence of caspase-8 probe in the RA/RX Liposome; green fluorescence indicates the fluorescence of MitoTracker Red. Scale bars: 10 μm. | |
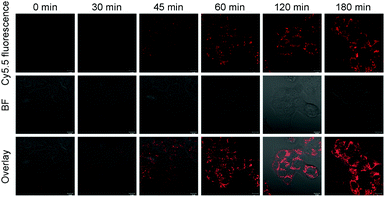 |
| Fig. 7 Activities of caspase-8 in HCT116 cells serially imaged using the RA/RX Liposome. Red fluorescence (Cy5.5) indicates the fluorescence of caspase-8 probe in the RA/RX Liposome. Scale bars: 10 μm. | |
Overcoming tumor hypoxia by the RA/RX Liposome
Since the antisense oligonucleotide (RX-0047) can inhibit the expression of HIF-1α, we therefore exploited the synergy between RX-0047 and RA-V to overcome hypoxia in cancer cells and improve the therapeutic efficacy. According to the results in Fig. 5d, the expression level of HIF-1α in HCT116 cells significantly decreased after treatment with the RA/RX Liposome, suggesting the relieving of the hypoxia conditions in the tumor. Next, the oxygen level in the tumor was further assessed using an oxygen-sensitive probe ([Ru(dpp)3]Cl2).46 When the cells were incubated with the RA/RX Liposome, the fluorescence intensity of [Ru(dpp)3]Cl2 decreased gradually along with the incubation time (Fig. S13†). These results demonstrated that the RA/RX Liposome can overcome a hypoxia environment thus enhancing the efficiency of cancer treatment.
In vivo therapy and therapeutic self-monitoring in subcutaneous tumor-bearing mice
Given the satisfactory results of the RA/RX Liposome in vitro, the treatment efficacy in vivo of the RA/RX Liposome was estimated. The therapeutic effects were assessed by monitoring the changes of the relative tumor volumes, hematoxylin and eosin (H&E) staining and terminal deoxynucleotidyl transferase Dutp nick end labeling (TUNEL) staining. As shown in Fig. 8a and Fig. S14,† the tumor growth in mice was clearly inhibited after the administration of the RA/RX Liposome and the tumor volume was decreased in vivo with an increase in the RA/RX Liposome dose (Fig. S15†). However, no significant therapeutic effect was observed in the mice treated with the RX-0047 Liposome or in the control group. To further confirm the high antitumor efficacy of the RA/RX Liposome in vivo, hematoxylin and eosin (H&E) staining on tissue sections after different treatments was investigated. A small number of necrotic cells appeared in the tumors treated with the RX-0047 Liposome and RA-V Liposome; in contrast, a large area of necrosis was observed in the tumor tissue of the mice treated with the RA/RX Liposome (Fig. 8c). The results were further confirmed by TUNEL staining and HIF-1α staining assay. The in vivo experiments showed that the therapy efficiency has been remarkably improved by combining RA-V with RX-0047. The high combination therapy efficiency was due to the synergistic effect between the anti-tumor activity of RA-V and the reduction of hypoxia induced by RX-0047.
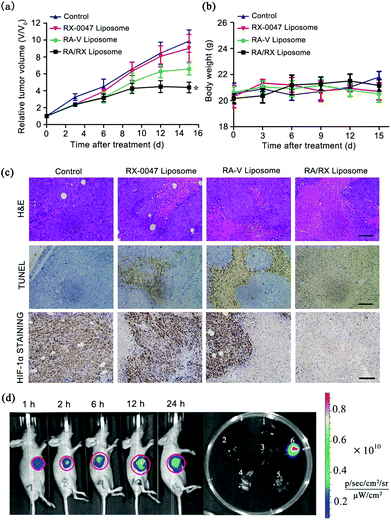 |
| Fig. 8 (a) Change in the relative tumor volume (V/V0) for different treatments. *P < 0.05. (b) Change in the body weight associated with different treatments. (c) Representative H&E stained images, TUNEL and HIF-1α staining analysis of tumor slices collected from different groups of mice with different treatments. Scale bar: 100 μm. (d) Time-dependent images in vivo and ex vivo fluorescence images. In vivo: Activities of caspase-8 of fluorescence images in HCT116 tumor-bearing mice with i.v. injection of the RA/RX Liposome recorded at different time intervals, fluorescence intensity successfully increased. Ex vivo images: 1: heart; 2: liver; 3: spleen; 4: lung; 5: kidney; 6: tumor, these indicate that apoptosis occurs mostly in tumor. | |
Moreover, the H&E-stained organ slices from the mice treated with the RA/RX Liposome showed no abnormalities in organ tissues compared with those of the age-matched healthy mice which received no treatment (Fig. S16†); in addition, the levels of serum ALT, AST, creatinine and BUN were also measured to assess liver and kidney toxicity, respectively. As shown in Fig. S17,† no significant changes were observed, confirming that the RA/RX Liposome can selectively kill cancer cells and prevent the damage to normal cells in vivo as a result of the targeting specificity and pH-controlled drug release.
Furthermore, the self-monitoring ability of the RA/RX Liposome was studied in HCT116 tumor-bearing BALB/c nude mice. After the intravenous (i.v.) injection of the RA/RX Liposome into the subcutaneous HCT116 tumor-bearing mice, an IVIS spectrum instrument was used for the imaging of the therapy process in vivo. As shown in Fig. 8d, the fluorescence intensity increases with time, reaching a maximum at 12 h post-treatment with the RA/RX Liposome, demonstrating the targeted delivery and caspase-activatable fluorescence of the RA/RX Liposome in the tumor. Moreover, the tumor tissue harvested from the RA/RX Liposome-treated mice displayed bright fluorescence, while very weak fluorescence was observed in other organs, indicating the specific activation properties of the RA/RX Liposome in tumor tissue. The results demonstrate that the anti-DR5 targeted delivery of the RA/RX Liposome and the reliability of therapeutic self-monitoring properties. To further confirm the synergistic effect between RA-V and RX-0047 on inducing apoptosis, the caspase 8 probe Liposome, RX-0047 Liposome and RA-V Liposome were used to produce in vivo imaging as controls. At 12 h post injection of the liposomes (three controls and the RA/RX Liposome), the fluorescence in the tumor region of the RA/RX Liposome-treated mice was much higher than those of three control-treated mice, which demonstrates that the high tumor-targeting imaging capability can be attributed to the increased level of caspase 8 after the highly efficient combination therapy (Fig. S18†).
Experimental section
Materials
Cholesteryl hemisuccinate (CHEMS) was purchased from Avanti Polar Lipids (USA). 1,2-Distearoyl-sn-glycero-3-phosphoethanolamine-N-[carboxy(polyethylene glycol)-2000] (DSPE-mPEG2000-COOH) and 1,2-dioleoyl-sn-glycero-3-phosphoethanolamine (DOPE) were purchased from Nanocs (New York, USA). N-Ethyl-N′-(3-(dimethylamino)propyl)carbodiimide (EDC), N-hydroxysuccinimide (NHS), and N-2-hydroxyethylpiperazine-N′-2-ethanesulfonic acid (HEPES) were obtained from Sigma-Aldrich (St Louis, MO, USA). Human caspase-3 recombinant proteins (active form, 25 units), human caspase-8 recombinant proteins (active form, 25 units), and human caspase-9 recombinant proteins (active form, 25 units) were purchased from BioVision (Milpitas, California, USA). Hoechst 33342, LysoTracker Red, MitoTracker Red and Annexin V-FITC/PI cell apoptosis kit were obtained from Invitrogen (Carlsbad, CA, USA). RX-0047 (d(P-thio)(AATGAGCCACCAGTGTCCAA)) was obtained from Sangon Biotech Ltd Co (Shanghai, China). RA-V (purity >99%) was prepared in our laboratory, and identified by MS and NMR spectroscopy. Anti-HIF-1α antibody was purchased from Abcam (Cambridge, UK) and anti-DR5 antibody was obtained from Bioworld (Minnesota, USA). Cy5.5-IETD-BHQ-3 (caspase-8 probe) was synthesized by Xi'an Ruixi Biological Technology Co., Ltd (Xi'an, China). Ultrapure water was prepared using a Millipore Simplicity System (Millipore, Bedford, USA).
Apparatus
Fluorescence spectra were measured using a fluorescence spectrophotometer (Shimadzu Company, Japan). Zeta potential and average hydrodynamic diameter measurements were performed using a Malvern Zetasizer Nano instrument (Malvern Company, UK) at 25 °C. Cytotoxicity assay was performed using a microplate reader (Biotek, USA). Fluorescence imaging of the cells was performed using a confocal laser scanning microscope (LSM700, Zeiss, Germany). Images were analyzed by a version of ZEN imaging software. Flow cytometric assay was performed with an Attune NxT flow cytometer (Thermo Fisher Scientific, USA). The liposome was sonicated using an ultrasonic processor (SCIENTZ, China).
Fabrication and characterization of the RA/RX Liposome
Liposomes were prepared by a thin film hydration method.29,46 Briefly, DOPE (32 mg), CHEMS (16 mg), DSPE-mPEG2000-COOH (3 mg), Dio (1 mg) and RA-V (0.42 mg) were dissolved in 10 mL of chloroform in a 50 mL round-bottom flask and then dried using a rotary evaporator under vacuum at 45 °C to obtain a thin lipid film, which was left under vacuum drying overnight to allow organic solvents to evaporate. Then, the lipid film was hydrated in 8 mL solution (with total 2 mg RX-0047 and 1 mg caspase-8 probe) by rotating the round-bottom flask at 180 rpm at 60 °C for 2 h. The resulting suspension was then sonicated with a probe sonicator (total for 2 minutes at a power of 100 W, with 1 second on/off pulse). In order to make the particles uniform, the liposomes were extruded through a 0.22 μm membrane. The liposomes were lastly purified using a dialysis membrane (MWCO = 14 kDa, Millpore) for 2 h to detach free agents. For modification of anti-DR5 at the surface of the liposomes, EDC and NHS at a ratio of 1
:
10 (mol/mol) dissolved in MES buffer at pH 5.0 was used for the activation of the carboxy group in the liposomes, which was added to 3 mL liposome suspension for 1 h at room temperature with moderate stirring. After centrifugation to eliminate the unused reagents, the liposomes were resuspended in 3 mL phosphate buffer saline (PBS). Anti-DR5 solution (40 μg) was added to the activated liposomes which were incubated overnight at 4 °C with the reaction of the carboxy group in the liposomes and the amino group of the peptide. Similar procedures were used to prepare the Empty Liposome, RX-0047 Liposome, and RA-V Liposome. The morphology of the liposomes was observed using TEM measurements. The RA/RX Liposome was dropped onto a carbon-coated copper grid, and 1.0% (w/v) phosphotungstic acid was added, allowing for negative staining. The size distribution and apparent zeta potential of the liposomes were measured using a Malvern Zetasizer Nano instrument. To conduct the experiments, 100 μL of the liposomes were added to 900 μL of ultrapure water (final volume = 1 mL), and the measurements were carried out in triplicate at 25 °C. Encapsulation efficiency (EE) determination process: after the destruction of the liposomes with acetonitrile in maximal intense vortex, the suspension was centrifuged at 12
000 rpm, for 30 minutes, to completely release the RA-V and RX-0047. The amounts of RA-V, RX-0047 and caspase-8 probe were determined using High Performance Liquid Chromatography (HPLC). EE was calculated using the following equation:
In the preparation of the RA/RX Liposome, the adding order was RA-V, RX-0047 and caspase-8 probe. The encapsulation efficiencies of RA-V, RX-0047 and caspase-8 probe in the particle were 62.7%, 24.7% and 25.5%, respectively. On changing the incubation order (RA-V, caspase-8 probe, RX-0047), the efficiencies of the RA-V/RX-0047/caspase-8 probe were 61.9%, 25.1% and 25.8%, respectively.
In vitro release of RA-V from the RA/RX Liposome
A dialysis bag (MWCO = 14 kDa, Millpore) with 3 mL of the RA/RX Liposome was introduced into a 50 mL beaker with 20 mL of the HEPES medium gently shaken at 100 rpm and 37 °C. 1 mL samples were selected for the determination of the concentration at a fixed time and immediately replaced with the same volume to maintain a fixed volume. The samples were assayed for RA-V by HPLC at 230 nm. The percentage of RA-V released was calculated using the equation:
RA-V released% = (Ct × Vt + Y)/M × 100% |
where Vt is the volume in the container at time t; Ct is the concentration of RA-V at t time, Y is total amount of RA-V that has already been collected, and M is the total amount of RA-V in the dialysis bag at the beginning.
Fluorescence assay
The fluorescence activation of the caspase-8 probe was investigated. Caspase-8 probe (100 nM) was added to 1 mL reaction buffer (pH 7.2, 50 mM HEPES, 10 mM DTT, 50 mM NaCl, 10 mM EDTA, 0.1% w/v CHAPS, 5% v/v glycerol), followed by a sequentially diluted activated caspase-8 (0, 0.25, 0.50, 1.00, 1.50, 3.00, and 6.00 nM) for 1 h at 37 °C. For further investigation of the specificity response of the caspase-8 probe to caspase-8, caspase-8 (2 nM), caspase-3 (2 nM), caspase-9 (2 nM), BSA (2 nM), pancreatin (2 nM), and caspase-8 plus Z-IETD-FMK (10 μM) were respectively added to a 1 mL reaction buffer containing 100 nM caspase-8 probe (pH 7.2, 50 mM HEPES, 10 mM DTT, 50 mM NaCl, 10 mM EDTA, 0.1% w/v CHAPS, 5% v/v glycerol) and allowed to react for 1 h, which were later measured with a fluorescence spectrophotometer. Excitation is at 685 nm, and emission is between 690 nm to 750 nm.
Cell culture and confocal fluorescence imaging
A human colon cancer cell line (HCT116 cells and HT29 cells) and human normal intestinal epithelial cells (NCM460 cells) were purchased from the Cell Bank of Chinese Academy of Sciences (Shanghai, China). HCT116 cells were cultured in an IMDM medium containing 10% fetal bovine serum and HT29 cells and NCM460 cells were cultured in a DMEM medium containing 10% fetal bovine serum. All cells were cultured in an incubator at 37 °C under a humidified 5% CO2/95% air atmosphere. For intracellular fluorescence imaging, HCT116 cells and HT29 cells were seeded with a density of 4.5 × 105 cells per mL and NCM460 cells were seeded with a density of 2.5 × 105 cells per mL in glass bottom dishes for one day before imaging. Cell imaging was performed after washing with PBS three times. The confocal images were obtained using a ZEISS Laser Scanning Microscope (LSM700, Zeiss, Germany) with a 63× oil-immersion objective.
In vitro cytotoxicity assay
Sulforhodamine B (SRB) assay was used for the evaluation of the cytotoxicity of the RA/RX Liposome on HCT116 cells and on HT29 cells. Briefly, HCT116 cells were seeded in 96-well plates with a density of 4000 cells in each well in a 200 μL medium the day before incubation with Empty Liposome, Free RX-0047, RX-0047 Liposome, Free RA-V, RA-V Liposome, and RA/RX Liposome for 48 h. Similarly for HCT116 cells, HT29 cells were also seeded in 96-well plates with a density of 10
000 cells in each well in a 200 μL medium the day before treatments for 48 h. The dose of Free RA-V in the cytotoxicity assay was equal to the amount of RA-V in the RA/RX Liposome. 50 μL of 50% TCA was added to each well and fixed for 1 h after treatments, and then washed three times with water; later, 100 μL of 4% SRB was added to each well and staining was performed for 15 minutes. Then we washed each well three times with 1% TCA to remove excess dye, and then dried in an oven. Finally, 100 μL of 10 mM unbuffered Trisbase (pH = 10.5) was used to dissolve the stained cells, and measurements were made at 540 nm using a microplate reader (Biotek, USA).
Western blotting analysis
The protein of HIF-1α and procaspase-8 expression was measured using a western blotting technique. HT29 and HCT116 cells were harvested at 6 h after treatment with Empty Liposome, RX-0047 Liposome, RA-V Liposome (100 nM RA-V equiv.), RA/RX Liposome (100 nM RA-V equiv.), and total protein extracts were prepared using Novex™ Tris-Glycine SDS Sample Buffer (2×) (Carlsbad, CA, USA). The protein lysates were equally subjected to sodium dodecyl sulfate polyacrylamide gel electrophoresis (SDS-PAGE), followed by their transfer to polyvinylidene difluoride membranes (Millipore). Then, we blocked the membranes for 2 h with 5% milk in TBST, which was prepared in our laboratory. Afterwards, the membranes were incubated with the primary antibodies overnight at 4 °C, and then incubated with HRP-label antibodies for 2 h. Finally, a chemiluminescent substrate (by HRP catalysis) was used to detect protein bands.
Mitochondrial membrane potential (JC-1) assay
HCT116 cells and HT29 cells were treated with the RA/RX Liposome (100 nM RA-V equiv.) for 12 h, followed by incubation with 10 μg mL−1 JC-1 (5,5,6,6-tetrachloro-1,1,3,3-tetraethylbenzimidazol-carbocyanineiodide) for 30 minutes and then the cells were imaged after washing three times with cold-PBS. A ZEISS Laser Scanning Microscope (LSM700, Zeiss, Germany) with a 63× oil-immersion objective was used for imaging.
Overcoming tumor hypoxia by the RA/RX Liposome
[(Ru(dpp)3)]Cl2 is an O2 sensing probe, which is used to investigate the relieving of hypoxia conditions in cancer cells. HCT116 cells were pre-incubated with 5 μM [Ru(dpp)3]Cl2 for 4 h, and then treated with the RA/RX Liposome (100 nM RA-V equiv.) for 0 h, 3 h, 6 h, and 12 h. The fluorescence of [(Ru(dpp)3)]Cl2 in HCT116 cells was imaged at 488 nm excitation, and the emission was collected between 600 nm and 700 nm.
Modeling subcutaneous tumor-bearing nude mice and in vivo visualization of apoptosis and therapy
2 × 106 HCT116 cells were subcutaneously injected into the right dorsal flank of 6 to 8 week-old female athymic nude BALB/c mice. All animal procedures were performed in accordance with the Guidelines for Care and Use of Laboratory Animals of China Pharmaceutical University and were approved by the Animal Care Committee of China Pharmaceutical University. All animals were raised in a sterile environment. The tumor-bearing mice were divided into several groups when tumor volumes reached 100 mm3. PBS, RX-0047 Liposome, RA-V Liposome, and RA/RX Liposome were injected into the tail vein of the tumor-bearing mice at a dosage of 2.5 mg kg−1 RA-V. From Day 0, the tumor-bearing nude mice were intravenously injected every three days for 15 days, and during this time, the tumor size was measured. The mice were sacrificed at the end of the study in order to obtain the organ and tumor for H&E staining and immunohistochemistry of TUNEL analysis. In order to visualize the apoptosis in vivo, the RA/RX Liposome containing RA-V at a dosage of 2.5 mg kg−1 was injected into the tumor-bearing mice through the tail vein. At 1 h, 2 h, 6 h, 12 h and 24 h, an IVIS spectrum instrument was used to obtain the fluorescence images with the mice being anesthetized. The ex vivo fluorescence biodistribution image of the organs and tumor tissue from HCT116 tumor-bearing mice was taken at 12 h post injection of the RA/RX Liposome.
Conclusions
In conclusion, we have established a new anti-DR5 targeted, chemosensitization system which provides a highly selective and efficient combination therapy in hypoxia tumors. In this work, the anti-DR5 targeting specificity and pH-responsive feature identify the RA/RX Liposome to have high selectivity for programmed drug delivery at the cellular level. The RA/RX Liposome also achieved significant HIF-1α silencing in the RA-V-mediated apoptosis process to enhance the chemotherapy efficacy in hypoxic tumors. More importantly, the RA/RX Liposome achieved therapeutic self-monitoring using dynamic visualization of the caspase-8 activation in situ. Briefly, this chemosensitization system provides a new strategy for a more efficient combination therapy in O2-deprived tumors and provides deep insight into the mechanisms of the mitochondria-related apoptotic process.
Conflicts of interest
There are no conflicts to declare.
Acknowledgements
We thank the National Natural Science Foundation of China (21602255 and 31470428), the Natural Science Foundation of Jiangsu Province (BK20160745), the National New Drug Innovation Major Project of Ministry of Science and Technology of China (2017ZX09309027), the Program of Innovative Research Team of Jiangsu Province, “Double First-Class” University Project of China Pharmaceutical University (CPU2018GF05), the Fund of Chinese Academy of Sciences (XDA09030301-4), and the Fundamental Research Funds for the Central Universities (2632018ZD08). The authors thank Dr Robert Paugh for help with English writing.
Notes and references
- K. D. Miller, R. L. Siegel, C. C. Lin, A. B. Mariotto, J. L. Kramer, J. H. Rowland, K. D. Stein, R. Alteri and A. Jemal, CA Cancer J. Clin., 2016, 66, 271–289 CrossRef PubMed
.
- R. L. Siegel, K. D. Miller, S. A. Fedewa, D. J. Ahnen, R. G. S. Meester, A. Barzi and A. Jemal, CA Cancer J. Clin., 2017, 67, 177–193 CrossRef PubMed
.
- E. J. Kuipers, W. M. Grady, D. Lieberman, T. Seufferlein, J. J. Sung, P. G. Boelens, C. J. Van De Velde and T. Watanabe, Nat. Rev. Dis. Primers, 2015, 1, 15065 CrossRef PubMed
.
- R. Dienstmann, R. Salazar and J. Tabernero, J. Clin. Oncol., 2015, 33, 1787–1796 CrossRef CAS PubMed
.
- R. Zhang, L. Z. Feng, Z. L. Dong, L. Wang, C. Liang, J. W. Chen, Q. X. Ma, R. Zhang, Q. Chen, Y. C. Wang and Z. Liu, Biomaterials, 2018, 162, 123–131 CrossRef CAS PubMed
.
- Y. C. Chae, V. Vaira, M. C. Caino, H. Y. Tang, J. H. Seo, A. V. Kossenkov, L. Ottobrini, C. Martelli, G. Lucignani, I. Bertolini, M. Locatelli, K. G. Bryant, J. C. Ghosh, S. Lisanti, B. Ku, S. Bosari, L. R. Languino, D. W. Speicher and D. C. Altieri, Cancer Cell, 2016, 30, 257–272 CrossRef CAS
.
- R. Dubey, M. D. Levin, L. Z. Szabo, C. F. Laszlo, S. Kushal, J. B. Singh, P. Oh, J. E. Schnitzer and B. Z. Olenyuk, J. Am. Chem. Soc., 2013, 135, 4537–4549 CrossRef CAS PubMed
.
- A. Patel and S. Sant, Biotechnol. Adv., 2016, 34, 803–812 CrossRef CAS
.
- L. C. Baker, J. K. Boult, S. Walker-Samuel, Y. L. Chung, Y. Jamin, M. Ashcroft and S. P. Robinson, Br. J. Cancer, 2012, 106, 1638–1647 CrossRef CAS
.
- H. S. Ban, B. K. Kim, H. Lee, H. M. Kim, D. Harmalkar, M. Nam, S. K. Park, K. Lee, J. T. Park, I. Kim, K. Lee, G. S. Hwang and M. Won, Cell Death Dis., 2017, 8, e2843 CrossRef CAS
.
- Y. Wang, M. Saad, R. I. Pakunlu, J. J. Khandare, O. B. Garbuzenko, A. A. Vetcher, V. A. Soldatenkov, V. P. Pozharov and T. Minko, Clin. Cancer Res., 2008, 14, 3607–3616 CrossRef CAS PubMed
.
- Y. Wang, R. I. Pakunlu, W. Tsao, V. Pozharov and T. Minko, Mol. Pharm., 2004, 1, 156–165 CrossRef CAS
.
- S. Dai, M. L. Huang, C. Y. Hsu and K. S. Chao, Int. J. Radiat. Oncol., Biol., Phys., 2003, 55, 1027–1036 CrossRef CAS
.
- Y. Wang, R. I. Pakunlu, W. Tsao, V. Pozharov and T. Minko, Mol. Pharm., 2004, 1, 156–165 CrossRef CAS
.
- M. Sowter, H. P. J. Ratcliffe, P. Watson, A. H. Greenberg and A. L. Harris, Cancer Res., 2001, 61, 6669–6673 Search PubMed
.
- Q. Wang, P. Zhang, J. Z. Xu, B. Xia, L. Tian, J. Q. Chen, J. Li, F. Lu, Q. M. Shen, X. M. Lu, W. Huang and Q. L. Fan, ACS Appl. Bio Mater., 2018, 1, 70–78 CrossRef CAS
.
- Q. Wang, L. Tian, J. Z. Xu, B. Xia, J. Li, F. Lu, X. M. Lu, W. J. Wang, W. Huang and Q. L. Fan, Chem. Commun., 2018, 54, 10328–10331 RSC
.
- J. Z. Xu, B. Xia, X. R. Niu, J. Cai, Z. Han, Q. Wang, X. M. Liu, Q. L. Lu and W. Huang, Dyes Pigm., 2019, 170, 107664 CrossRef CAS
.
- Q. Wang, B. Xia, J. Z. Xu, X. R. Niu, J. Cai, Q. M. Shen, W. J. Wang, W. Huang and Q. L. Fan, Mater. Chem. Front., 2019, 3, 650–655 RSC
.
- Q. Wang, Y. N. Dai, J. Z. Xu, J. Cai, X. R. Niu, L. Zhang, R. F. Chen and Q. M. Shen, Adv. Funct. Mater., 2019, 29, 1901480–1901492 CrossRef
.
- K. H. Bae, J. Y. Lee, S. H. Lee, T. G. Park and Y. S. Nam, Adv. Healthcare Mater., 2013, 2, 576–584 CrossRef CAS
.
- J. Zhu, H. Huang, S. W. Dong, L. Ge and Y. Zhang, Theranostics, 2014, 4, 931–944 CrossRef CAS
.
- D. B. Yang, T. F. Wang, Z. G. Su, L. J. Xue, R. Mo and C. Zhang, ACS Appl. Mater. Interfaces, 2016, 8, 22431–22441 CrossRef CAS
.
- N. H. Tan and J. Zhou, Chem. Rev., 2006, 106, 840–895 CrossRef CAS
.
- J. T. Fan, J. Su, Y. M. Peng, Y. Li, J. Li, Y. B. Zhou, G. Z. Zeng, H. Yan and N. H. Tan, Bioorg. Med. Chem., 2010, 18, 8226–8234 CrossRef CAS
.
- J. T. Fan, Y. S. Chen, W. Y. Xu, L. C. Du, G. Z. Zeng, Y. M. Zhang, J. Su, Y. Li and N. H. Tan, Tetrahedron Lett., 2010, 51, 6810–6813 CrossRef CAS
.
- G. G. Yue, J. T. Fan, J. K. Lee, G. Z. Zeng, T. W. Ho, K. P. Fung, P. C. Leung, N. H. Tan and C. B. Lau, Br. J. Pharmacol., 2011, 164, 1883–1898 CrossRef CAS
.
- Z. Y. Qiao, D. Zhang, C. Y. Hou, S. M. Zhao, Y. Liu, Y. J. Gao, N. H. Tan and H. Wang, J. Mater. Chem. B, 2015, 3, 4514–4523 RSC
.
- H. C. Chen, Y. R. Wang, Y. R. Yao, S. L. Qiao, H. Wang and N. H. Tan, Theranostics, 2017, 7, 3781–3793 CrossRef CAS
.
- X. Y. Fang, W. Chen, J. T. Fan, R. Song, L. Wang, Y. H. Gu, G. Z. Zeng, Y. Shen, X. F. Wu, N. H. Tan, Q. Xu and Y. Sun, Toxicol. Appl. Pharmacol., 2013, 267, 95–103 CrossRef CAS
.
- H. W. Leung, Z. Wang, G. G. Yue, S. M. Zhao, J. K. Lee, K. P. Fung, P. C. Leung, C. B. Lau and N. H. Tan, Biochim. Biophys. Acta, 2015, 1853, 1827–1840 CrossRef CAS
.
- J. H. Yang, T. Yang, W. Yan, D. Li, F. Wang, Z. Wang, Y. J. Guo, P. Bai, N. H. Tan and L. J. Chen, RSC Adv., 2018, 8, 23451–23458 RSC
.
- X. Y. Ji, L. H. Song, L. Sheng, A. H. Gao, Y. Zhao, S. X. Han, Y. C. Zhang, C. Zhu, S. M. Zhao, Z. Wang, B. H. Xu, L. Li, J. Li, N. H. Tan and B. Zhao, Cancers, 2018, 10, 449 CrossRef CAS
.
- H. C. Chen, F. Li, Y. R. Yao, Z. Wang, Z. H. Zhang and N. H. Tan, Theranostics, 2019, 9, 90–103 CrossRef CAS
.
- A. Adhikari, M. Xu and Z. J. Chen, Oncogene, 2007, 26, 3214–3226 CrossRef CAS
.
- Q. H. Liu, J. C. Busby and J. D. Molkentin, Nat. Cell Biol., 2009, 11, 154–161 CrossRef CAS
.
- Z. Wang, S. M. Zhao, L. H. Song, Y. Z. Pu, Q. Wang, G. Z. Zeng, X. Liu, M. Bai, S. Li, F. B. Gao, L. J. Chen, C. Wang and N. H. Tan, Cell Death Dis., 2018, 9, 715 CrossRef
.
- L. D'Ignazio, M. Batie and S. Rocha, Cells, 2018, 7, 102 CrossRef
.
- F. Fay, K. M. McLaughlin, D. M. Small, D. A. Fennell, P. G. Johnston, D. B. Longley and C. J. Scott, Biomaterials, 2011, 32, 8645–8653 CrossRef CAS
.
- D. Schmid, F. Fay, D. M. Small, J. Jaworski, J. S. Riley, D. Tegazzini, C. Fenning, D. S. Jones, P. G. Johnston, D. B. Longley and C. J. Scott, Mol. Ther., 2014, 22, 2083–2092 CrossRef CAS
.
- S. M. Abdelghany, D. Schmid, J. Deacon, J. Jaworski, F. Fay, K. M. McLaughlin, J. A. Gormley, J. F. Burrows, D. B. Longley, R. F. Donnelly and C. J. Scott, Biomacromolecules, 2013, 14, 302–310 CrossRef CAS
.
- Y. Yuan, C. J. Zhang, R. T. Kwok, D. Mao, B. Z. Tang and B. Liu, Chem. Sci., 2017, 8, 2723–2728 RSC
.
- W. Liu, S. J. Liu, Y. Q. Kuang, F. Y. Luo and J. H. Jiang, Anal. Chem., 2016, 88, 7867–7872 CrossRef CAS
.
- H. Li, H. Zhu, C. J. Xu and J. Yuan, Cell, 1998, 94, 491–501 CrossRef CAS
.
- L. Zhu, X. Huang, K. Y. Choi, Y. Ma, F. Zhang, G. Liu, S. Lee and X. Chen, J. Controlled Release, 2012, 163, 55–62 CrossRef CAS
.
- H. C. Chen, J. W. Tian, W. J. He and Z. J. Guo, J. Am. Chem. Soc., 2015, 137, 1539–1547 CrossRef CAS
.
- S. Draffehn and M. U. Kumke, Mol. Pharmaceutics, 2016, 13, 1608–1617 CrossRef CAS
.
- H. Ebi, R. B. Corcoran, A. Singh, Z. Chen, Y. Song, E. Lifshits, D. P. Ryan, J. A. Meyerhardt, C. Benes, J. Settleman, K. K. Wong, L. C. Cantley and J. A. Engelman, J. Clin. Invest., 2011, 121, 4311–4321 CrossRef CAS
.
- S. Misale, R. Yaeger, S. Hobor, E. Scala, M. Janakiraman, D. Liska, E. Valtorta, R. Schiavo, M. Buscarino, G. Siravegna, K. Bencardino, A. Cercek, C. T. Chen, S. Veronese, C. Zanon, A. Sartore-Bianchi, M. Gambacorta, M. Gallicchio, E. Vakiani, V. Boscaro, E. Medico, M. Weiser, S. Siena, F. Di Nicolantonio, D. Solit and A. Bardelli, Nature, 2012, 486, 532–536 CrossRef CAS
.
- I. N. Mistry and A. Tavassoli, ACS Synth. Biol., 2017, 6, 518–527 CrossRef CAS
.
Footnotes |
† Electronic supplementary information (ESI) available. See DOI: 10.1039/c9bm00905a |
‡ These authors contributed equally. |
|
This journal is © The Royal Society of Chemistry 2020 |
Click here to see how this site uses Cookies. View our privacy policy here.