DOI:
10.1039/C9BM01580A
(Paper)
Biomater. Sci., 2020,
8, 379-390
The effect of metal ions on endogenous melanin nanoparticles used as magnetic resonance imaging contrast agents†
Received
29th September 2019
, Accepted 30th October 2019
First published on 1st November 2019
Abstract
Melanin nanoparticles are of great importance in biomedicine. They have excellent affinity for metallic cations, especially paramagnetic ions, which has sparked interest in their application in the development of magnetic resonance imaging (MRI) contrast agents. In this work, we prepared ultrasmall water-soluble melanin nanoparticles, and investigated the binding properties of melanin toward different metal cations (Gd3+, Mn2+, Fe3+ and Cu2+), and compared their physicochemical properties and the MRI contrast enhancement ability in various metal chelated forms (MNP-PEG-M) in vitro and in vivo. We show that the saturation binding numbers of Gd3+, Mn2+, Fe3+ and Cu2+ per MNP-PEG were 49, 59, 69 and 62, respectively. MNP-PEG-Gd, MNP-PEG-Mn, MNP-PEG-Fe and MNP-PEG-Cu exhibited the maximum r1 relaxivities at the loading mass ratios of Gd3+
:
MNP = 1
:
1, Mn2+
:
MNP = 0.5
:
1, Fe3+
:
MNP = 0.1
:
1 and Cu2+
:
MNP = 0.1
:
1, corresponding to 49, 57, 54 and 51 chelated metals per MNP-PEG, respectively. The maximal per metal ion r1 relaxivity values were 61.9, 48.7, 11.1 and 9.7 mM−1 s−1 for MNP-PEG-Gd, MNP-PEG-Mn, MNP-PEG-Fe and MNP-PEG-Cu at 1.5 T, respectively. MNP-PEG-Gd and MNP-PEG-Fe presented larger sizes (6.9 nm and 5.8 nm) than MNP-PEG-Mn and MNP-PEG-Cu (3.4 nm and 3.7 nm), all featuring excellent solubility, high stability and ultrasmall size. A significant in vivo MRI signal enhancement in tissues was observed for all MNP-PEG-M after intravenous injection in mice, and these nanoparticles were excreted through renal and hepatobiliary pathways. In agreement with their r1 relaxivity values, MNP-PEG-Gd and MNP-PEG-Mn showed a significantly greater in vivo tissue maximum enhancement than MNP-PEG-Fe and MNP-PEG-Cu. This study could yield valuable insight into the development of a new class of MRI contrast agents.
1. Introduction
Magnetic resonance imaging (MRI), providing noninvasive anatomical and physiological information with high spatial and temporal resolution, has become one of the most prominent imaging techniques in clinics.1,2 In general, MRI mainly measures the magnetic relaxation times of tissue-dependent water protons, either in longitudinal T1 or transversal T2 mode. The sensitivity and diagnostic accuracy of MRI were greatly improved due to the emergence of contrast agents.3,4 At present, gadolinium (Gd)-based small molecule contrast agents are widely used in clinical MRI scans, while their low sensitivity, non-specificity, latent toxicity and fast elimination from the circulation system still remain challenging problems.5,6 The development of nano-contrast agents has attracted great attention due to their targeting ability, long blood circulation, high relaxivity, low dosage and toxicity. Nano-contrast agents based on inorganic and organic nanoparticles with diverse sizes, charges, morphologies and surface chemistry properties have been designed for MRI scans.4,7,8 Inorganic nano-contrast agents exhibit good imaging properties while their potential long-term toxicity limits their clinical translation. Organic nano-contrast agents, including liposomes, micelles, and polymer nanoparticles, highlight the power of biomimicry for the development of biocompatible, bioregenerative, or biodegradable materials for biomedical applications.
Compared with exogenous nanomaterials, endogenous biopolymers in living organisms have inspired the generation of biocompatible, bioregenerative, and biodegradable nanomaterials for avoiding the side effects.9,10 Melanin, a pigment present in most organisms, has attracted much attention for biomedical applications because of its interesting properties and various biological functions, including photoprotection, photosensitization, antibiotic activity, thermoregulation, free radical quenching, metal ion chelation, and even involvement in the nervous system.11–14 Notably, melanin can be used as an MRI contrast agent owing to not only its paramagnetic characteristics but also its strong chelating performance to metal ions. Melanin contains a population of catechol groups which can act as a scaffold for the chelation of paramagnetic metal ions, leading to brighter T1-weighted MR images. It is found that the MRI signal exerted a remarkable enhancement after melanin chelation with metal ions.15,16 Recently, several attempts have been made to develop melanin-based nanoparticles as MRI contrast-enhancing agents with high relaxivity, desirable biosafety and mitigation of side effects.16,17 Ju et al. synthesized water-dispersible Fe3+ chelated melanin-like nanoparticles,18 which showed high biocompatibility and efficient T1-weighted MRI contrast enhancement. Metal ion (gadolinium, iron and manganese) chelated melanin nanoparticles have also been reported as potential MRI T1 contrast agents for diagnostics and interventional guided scanning, with comparable spatial and temporal resolution since fast spin–lattice relaxation processes occur in both free radical systems and paramagnetic metal ions in melanin complexes.17,19,20 Therefore, melanin-based contrast agents with endogenous composition could show great promise in MRI based on their strong binding capacity to paramagnetic metal ions and satisfactory biocompatibility.
Although previous studies have shown the potential of melanin as a contrast agent for MRI, most melanin or melanin-like nanoparticles have large size and poor solubility, and have a latent limitation in their practical application. The pioneering study performed by our research group has obtained ultrasmall soluble melanin nanoparticles.19,21 After chelation with Mn2+ ions, the nanoparticles displayed high relaxivity, and they could be excreted through both renal and hepatobiliary pathways. However, there remains a lack of knowledge regarding the regulation of size, the binding capacity of different metal ions to melanin, the optimization of relaxivity in vitro and in vivo, and the pathway to excretion of melanin in various metal chelated forms. Very few comparative studies have explored their potential as contrast agents for MRI for advanced medical imaging applications.
From this motivation, we prepared ultrasmall water-soluble melanin nanoparticles, and investigated the binding properties of melanin toward different metal cations (Gd3+, Mn2+, Fe3+ and Cu2+), and compared their contrast enhancement effects in various metal chelated forms in vitro or in vivo (Fig. 1). We expect that this study will yield valuable insight into the development of safe and efficient MRI contrast agents, and lay the foundation for future clinical translation of melanin nanoparticles as a new class of MRI contrast agents.
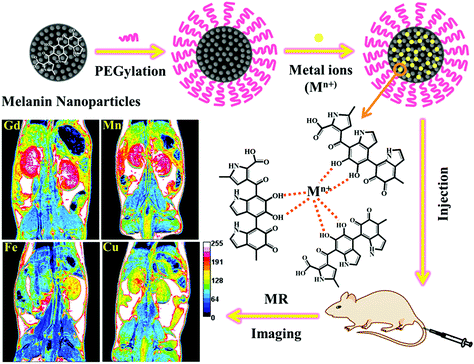 |
| Fig. 1 Preparation scheme of metal ion-loaded melanin nanoparticles for MRI contrast agents. | |
2. Materials and methods
2.1. Materials
Melanin and GdCl3·6H2O were purchased from Sigma-Aldrich. Sodium hydroxide (NaOH), hydrochloric acid (37 wt% HCl), MnCl2·4H2O, CuCl2·2H2O and FeCl3·6H2O were provided by Sinopharm Chemical Reagent Beijing Co., Ltd. Amine-PEG5000-amine (NH2-PEG5000-NH2, 5 kDa) was acquired from Shanghai Zzbio Co., Ltd. Cell Counting Kit-8 (CCK-8) was purchased from Shanghai YEASEN Co., Ltd. All chemical agents were obtained and used directly without further purification. Deionized water was used for all experimental procedures.
2.2. Preparation and characterization of MNP-PEG-M
Melanin nanoparticles (MNPs) were prepared according to our previously published method with some modifications.19 In a typical preparation, 10 mg melanin was dissolved in 0.1 M NaOH aqueous solution with the assistance of ultrasound. After complete dissolution, 0.1 M HCl aqueous solution was dropped to the above mixed solution to adjust the pH to neutral under sonication. The neutralized solution was centrifuged and washed several times using 30 kDa Molecular Weight Cut Off (MWCO) filters to purify the product. Finally, MNP powder was obtained by a freeze-drying process.
In a previous study, it was found that MNPs would be precipitated after combination with excess metal ions. To improve the biocompatibility, physiological stability and metal ion-chelation ability, MNPs were functionalized with NH2-PEG5000-NH2 molecules. Briefly, an MNP aqueous solution (1 mg mL−1) having a pH of 9.5 was added dropwise to the NH2-PEG5000-NH2 solution and stirred overnight, and then the solution was centrifuged and washed several times to remove the free PEG molecules.
A metal ion (Mn+ = Gd3+, Mn2+, Fe3+ and Cu2+) solution was dropped into the PEGylated MNP solution and stirred at room temperature for 4 h. The mass ratios of Mn+ to MNP were 1
:
0.05, 1
:
0.1, 1
:
0.5, 1
:
1, 1
:
3 and 1
:
5. The complexes were centrifuged (3500 rpm, 15 min) several times using 30 kDa MWCO filters. The resulting samples were labeled as MNP-PEG-M (MNP-PEG-Gd, MNP-PEG-Mn, MNP-PEG-Fe and MNP-PEG-Cu).
Radical centers of MNPs were characterized by an EMXplus-10/12 electron paramagnetic resonance system (EPR, Bruker, Germany). The molecular weight of MNPs was measured on a Bruker Ultraflextreme matrix-assisted laser desorption/ionization time of flight (MALDI-TOF)/TOF mass spectrometer (Bruker Daltonics, Inc., Billerica, MA). The concentration of metal ions was characterized by inductively coupled plasma mass spectrometry (ICP-MS, Thermal Elemental X7). The number N of metal ions bound to the MNP was calculated using the following equations:
N
M and NMNP represent the numbers of metal ions and MNP molecules, CM and CMNP represent concentrations, and MM and MMNP represent relative molecular masses. Metal ion-chelated MNP stability was analyzed by a dialysis experiment in PBS solution. The releasing metal ions were tested by ICP-MS. The morphologies of MNP-PEG-M were observed by transmission electron microscopy (JEM-2100F). A Nano-Zetasizer system (Malvern Instruments Ltd) was used to determine the size distribution and zeta-potential of MNP-PEG-M.
2.3. Cell culture and cytotoxicity of MNP-PEG-M
The H9c2 rat ventricular cell line was purchased from the cell bank of the Chinese Academy of Sciences (Shanghai, China). Rat H9c2 cells were cultured in high glucose Dulbecco's modified Eagle's medium (DMEM, GIBCO) supplemented with 10% fetal bovine serum (HyClone, Logan, UT, USA) and 1% penicillin–streptomycin (HyClone, Logan, UT, USA) solution under a humidified atmosphere of 5% CO2 at 37 °C.
To evaluate the cytotoxicity of MNP-PEG-M, H9c2 cells were seeded in 96-well plates (80
000 cells per well) and incubated with a series of concentrations of MNP-PEG-M (800, 400, 200, 100, 50, 25, and 0 μg mL−1) for 24 h at 37 °C under 5% CO2. Then the cellular viability was determined by CCK-8 assay. Four replicates were conducted for each group.
2.4. Evaluation of MRI performance of MNP-PEG-M in vitro and in vivo
In vitro MRI experiments were performed at 25 °C in a 1.5 T MR clinical scanner (Magnetom Trio with Tim, Siemens, Germany) with an abdomen surface coil. 1.5 mL of MNP-PEG-M with various concentrations of metal ions (0, 0.025, 0.05, 0.1, 0.2 and 0.4 mM) in aqueous solutions were transferred to 48-cell plates and imaged. The imaging parameters were as follows: field of view (FOV), 200 × 200 mm2; slice thickness, 8 mm; spacing, 0.5 mm; base matrix resolution, 512 × 512; repetition time (TR), 500 ms; and effective echo time (TE), 12 ms. The r1 values were calculated from the slope of the curve-fitting of 1/T1 (s−1) versus the metal ion concentration (mM).
Female BALB/c mice that were 4–6 weeks old (∼20 g) were obtained from the Animal Culture Center of Shanxi Medical University. All animal experiments were performed in strict accordance with the NIH guidelines for the care and use of laboratory animals (NIH Publication No. 85-23 Rev. 1985) and were approved by the Institutional Animal Use and Care Committee of Shanxi Medical University (Approval No, 2016LL141, Taiyuan, China). The in vivo performance of MNP-PEG-M, as MRI contrast agents, was evaluated with 3.0 T clinical MRI equipment (Trivo, Siemens). Female BALB/c mice were anesthetized via intraperitoneal injection of 10% chloral hydrate (0.3 mg kg−1), followed by collecting the coronal and axial MR images as the data of pre-injection. Afterward, the mice (n = 3 per compound) were intravenously injected with saline solutions at an MNP-PEG-M concentration of 1 mg (50 mg kg−1) per mouse equivalent to a metal ion dose of 1–1.6 μmol per mouse or 0.05–0.08 mmol kg−1. The axial and coronal T1-weighted MR images were acquired at appropriate time intervals (0 h, 0.5 h, 1 h, 2 h, 6 h, 24 h and 48 h) after injection using 3.0 T clinical MRI equipment (Philips) with a small animal magnetic resonance coil (diameter 10 cm). The in vivo MRI effect was evaluated by MR Signal Enhancement (SE) which was calculated as follows:
SE = (SIpost − SIpre)/SIpre × 100% |
SI
pre and SI
post represent the signal intensity in the tissue pre- and post-injection of a melanin contrast agent, respectively.
2.5.
In vivo biodistribution
For in vivo biodistribution of various metal ion-chelated MNPs, BALB/c mice (n = 4) were intravenously injected with MNP-PEG-M (5 mg mL−1, 200 μL). After 24 h, the major organs and tissues (heart, liver, spleen, lung, kidney and small intestine) were dissected, weighed and digested. The concentrations of metal ions were determined by ICP-MS. The levels of MNP-PEG-M in various organs and tissues were presented as the percentage of the injected dose per gram of tissue (%ID g−1).
2.6.
In vivo biosafety assessment
The body weights of BALB/c mice (n = 3) were inspected every other day until 30 days. The mice were randomly divided into five groups (n = 3 per group) as follows: control, MNP-PEG-Gd, MNP-PEG-Mn, MNP-PEG-Fe and MNP-PEG-Cu, which were then intravenously injected with PBS solution (control group) and MNP-PEG-M (50 mg kg−1). The blood samples were collected after 1 day and 7 days. The blood samples before injection were used for comparison. Two important hepatic indicators ALT (alanine aminotransferase) and AST (aspartate aminotransferase) and two indicators for kidney functions SCr (serum creatinine) and BUN (blood urea nitrogen) were measured. The complete blood panel data from the MNP-PEG-M and control group were tested. Parts of the harvested organs were fixed in 4% formalin at room temperature for 48 h, paraffin embedded, sectioned at a thickness of 8 μm and stained with H&E, and then imaged by using a CX-31 optical microscope.
2.7. Statistical analysis
All statistical analyses were performed using SPSS 22.0 software. Data were presented as mean ± SD. Means were compared using t-tests, a 95% confidence level was chosen to determine the significance between groups, and p < 0.05 was considered as statistically significant.
3. Results and discussion
3.1. Preparation and characterization of MNP-PEG-M
A pristine melanin granule was dissolved in 0.1 M NaOH and then neutralized with HCl aqueous solution with the assistance of sonication to decrease interchain aggregation. After centrifuging and washing several times using 30 kDa MWCO filters, water-soluble melanin nanoparticles (MNPs) were obtained. The MNPs not only provided ultrasmall size and favorable water-solubility but also preserved good biocompatibility and biodegradability of natural melanin.19 It is commonly accepted that melanin is a heterogeneous biopolymer composed of 5,6-dihydroxyindole (DHI) and 5,6-dihydroxyindole-2-carboxylic acid (DHCIA) units. One of the most unusual properties of melanin is a conjugated π-electron system, which exhibits a relatively large amount of free radical sites in this unique biopolymer. The EPR signal appeared as a broad peak with no hyperfine splitting which is a hallmark of all melanin, and the axially symmetric g-tensor parameter was 2.02366, indicating the existence of characteristic stable free radical centers in MNPs (Fig. S1†).15,18 Strong interactions of melanin radicals with paramagnetic or diamagnetic ions have also been studied using deliberately designed EPR experiments, and the result indicated that the binding of metal ions on melanin is based on the chelation of metal ions with free radicals rather than physical adsorption or chemical oxidation.15,22,23 However, it has been found that the size of melanin nanoparticles became large and then precipitated after directly chelating excess metal ions, which obviously limited their biomedical application. To retain the water-solubility, prolong the circulation time in blood and further improve the in vivo behavior, NH2-PEG5000-NH2 was introduced to generate ultrasmall MNP-PEG, which was a simple, time-saving, and low energy-consuming modification process and rendered nanoparticles stable against biofouling and aggregation under physiological conditions.24 The MNP-PEG has been demonstrated in a previous report,19 and showed excellent solubility and dispersion after binding with metal ions. The successful PEGylation was confirmed by the subsequently synthesized metal ion chelated MNPs which exhibited excellent water-dispersibility and a characteristic stable free radical feature.
Next, we investigated the binding abilities of MNP-PEG towards various paramagnetic metal ions including Gd3+, Mn2+, Fe3+ and Cu2+. The molecular weight of the MNPs was about 40 kDa according to the MALDI-TOF result (Fig. 2a), and the concentration of metal ions can be obtained from the ICP results; thus the number N of metal ions bound to one MNP, manifested as the binding capacities, was given as a function of the mass ratio of initially loaded amounts of metal ions to the specific amount of MNPs (Fig. 2b). It is found that the binding capacities of metal ions steadily increased on increasing the loaded mass ratio of metal ions to melanin. When the mass ratio was 1, the loaded amounts of various metal ions reached the saturation point of binding and excess amounts of metal ions were removed through centrifugation. The maximum binding number of Gd3+, Mn2+, Fe3+ and Cu2+ to MNP-PEG was calculated as 49 ± 3, 59 ± 3, 69 ± 4 and 62 ± 2 per melanin nanoparticle, respectively; thus the maximum binding capacities of various metal ions to MNP-PEG were in the order Fe3+ > Cu2+ > Mn2+ > Gd3+. The difference of binding capacities is likely related to the ionic radius and ligand geometry of the metal ions. The ionic radii of Gd3+, Mn2+, Fe3+ and Cu2+ are 93.8, 80, 64 and 72 pm, respectively. The larger ionic radius of Gd3+ leads to a lower chelated metal ion number, and Fe3+ with a smaller ionic radius leads to a greater chelated metal ion number. Moreover, fewer metal ion coordination ligands may contribute to more number of chelated metal ions to melanin. In the case of binding Fe3+, the o-dihydroxyl groups of the catechol have been proven to be much more important to form 4–6 coordinate high-spin complexes with distorted octahedral or rhombic symmetry.18,20 The Cu2+ coordination in melanin was reported as four ligands,22 while the ligand coordination of Gd3+ is six.
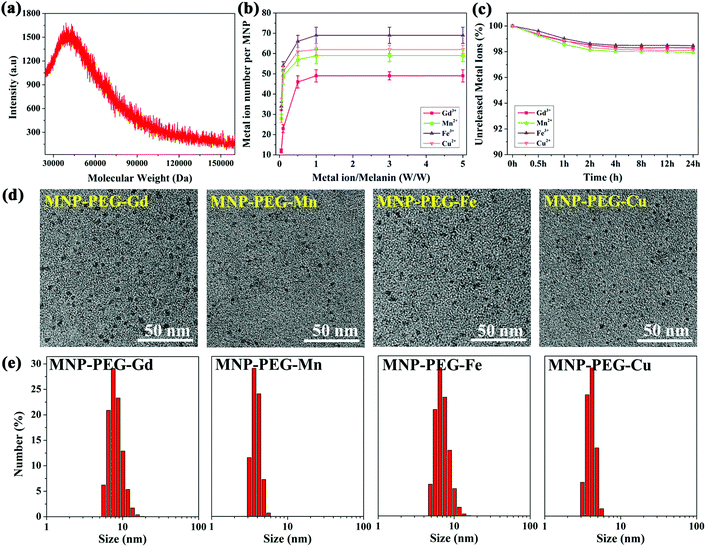 |
| Fig. 2 Characterization of physicochemical properties: (a) molecular weight of melanin nanoparticles measured by MALDI-TOF MS. (b) Chelated number of different metal ions on per melanin nanoparticles as a function of initially loaded mass ratio of metal ion to melanin. (c) Stability of MNP-PEG-M with various metal ions in PBS (pH = 7.4). (d) TEM images and (e) hydrodynamic diameters of MNP-PEG-Gd, MNP-PEG-Mn, MNP-PEG-Fe, and MNP-PEG-Cu with initial loaded metal ion to melanin mass ratios of 1 : 1, 0.5 : 1, 0.1 : 1 and 0.1 : 1, respectively. | |
The stability of chelated metal ions is a prerequisite to ensure their in vitro and in vivo MR imaging performance. In pH = 7.4 PBS solution, the nanoparticles demonstrate high stability. Notably, the metal ion content of each nanoparticle was still up to 98% compared with the original value after 24 h exposure to PBS (Fig. 2c). The less than 2% release of metal ions before 2 h may derive from those that were absorbed on the nanoparticles through weak electrostatic interactions.
Reflecting the optimized chelating conditions of various metal ion chelated nanoparticles, we further investigated the physicochemical properties, and the corresponding results are shown in Fig. 2d, e and Table 1. TEM images of four nanoparticles in Fig. 2d show the morphology and dispersity, which feature ultrasmall size and high dispersity. MNP-PEG-Gd and MNP-PEG-Fe presented larger size (6.9 nm and 5.8 nm) than MNP-PEG-Mn and MNP-PEG-Cu (3.4 nm and 3.7 nm). The average hydrodynamic diameters of MNP-PEG-M were confirmed by the typical DLS (Fig. 2e), which showed similar results to TEM. The zeta potentials of MNP-PEG-M were also determined and are shown in Table 1. All the nanoparticles exhibited a negative zeta potential, and were stable in physiological environments.
Table 1 Physicochemical parameters of MNP-PEG-M nanoparticles as MRI contrast agents
|
Size/TEM (nm) |
Hydrodynamic size/DLS (nm) |
Zeta potential (mV) |
Relaxivity/max (mM−1 s−1) |
MNP-PEG-Gd |
6.9 |
7.5 |
−9.8 ± 3.1 |
61.853 |
MNP-PEG-Mn |
3.4 |
3.6 |
−17.3 ± 4.2 |
48.721 |
MNP-PEG-Fe |
5.8 |
6.5 |
−6.5 ± 3.4 |
11.085 |
MNP-PEG-Cu |
3.7 |
4.1 |
−18.6 ± 3.8 |
9.732 |
3.2.
In vitro MRI
To investigate the MRI signal-enhancing capability of various metal ions, the relaxivity values of MNP-PEG-M with different loading mass ratios of metal ions to MNPs were measured using a 1.5 T clinical MR scanner. No appreciable signal enhancement was observed in the samples of MNPs (Fig. S2†), suggesting that melanin had a negligible effect on the MRI contrast. A similar result was indicated by Godechal and coworkers who found no significant correlation between the melanin and the relaxation time.25 Moreover, the PEGylation process of melanin did not show obvious changes in relaxation performance.18,19T1-Weighted images and the relaxivity values of paramagnetic metal ion (Gd3+, Mn2+, Fe3+ and Cu2+) chelated MNP-PEG nanoparticles are shown in Fig. 3. Phantom images of MNP-PEG-M all displayed positive T1-weighted contrast signals confirming that chelation with paramagnetic metal ions was necessary for melanin as an MRI contrast agent. Specifically, in Fig. 3a, MNP-PEG-Gd with different initial loading mass ratios of Gd3+ ions to melanin presented significant brighter images than Gd-DTPA (a commercial Gd based T1 contrast agent) at the same Gd3+ concentrations. Moreover, apparent differences of T1-weighted images were observed among MNP-PEG-Gd with various loading mass ratios of Gd3+
:
MNP, and the samples with a mass ratio of 1
:
1 showed a set of brightest images. By measuring the relaxation times of samples at different molar concentrations of metal ions, the relaxivity (r1, mM−1 s−1) was determined for each MNP-PEG-M from the slope of each fitting curve and is shown in Fig. 3. MNP-PEG-Gd with a loading mass ratio of Gd3+
:
MNP = 1
:
1 had the maximum relaxivity of 61.853 mM−1 s−1 which was about 10.3 times as high as that of commercial Gd-DTPA (5.978 mM−1 s−1). On increasing the Gd3+ contents, the T1 contrast effects gradually enhanced, and the relaxivity values were 38.2, 44.6, and 55.4 mM−1 s−1 with the loading mass ratios of Gd3+
:
MNP = 0.05
:
1, 0.1
:
1, and 0.5
:
1 (or 12, 23, and 46 chelated Gd3+ ions per MNP-PEG), respectively. When the loading mass ratio was 1
:
1, the number of Gd3+ ions (49 per MNP-PEG) no longer increased, and the relaxivity reached the maximum, which means that the exchange of MNP-PEG-Gd with water molecules approached saturation. As shown in the TEM images (Fig. S3†), the sizes of MNP-PEG-Gd showed little change at low loading mass ratios from 0.05
:
1 to 1
:
1. Hence neither the overall rotational time nor the nanoparticle size but rather the interaction mode of the bound Gd3+ ions with water molecules had an effect on the relaxivity differences among them. The outer surface bound Gd3+ ions have more exposure to the bulk water than the inner core bound Gd3+ ions. Therefore, as the surface bound Gd3+ ions increased, the exchange rate with water molecules increased to induce higher relaxivity. The relaxivity value approached the maximum when the surface binding saturated. The result revealed a binding order “from the inner core to surface” for Gd3+ ions to MNP-PEG, that is, the proportion of Gd3+ ions bound to the outer surface area over the inner core area increases as the Gd3+ content increases. Further increasing the loading mass ratio of Gd3+
:
MNP-PEG to 3
:
1 or 5
:
1, however, decreased the relaxivity value (Fig. 3a). To explain this phenomenon, we compared the TEM images of MNP-PEG-Gd with various loading mass ratios as shown in Fig. S3.† Aggregation occurred among the nanoparticles and the sizes increased at high loading mass ratios of 3
:
1 and 5
:
1. It has been reported that strong surface effects of ultrasmall scale nanostructures play an important part in high relaxivity which exposed more metal ions and hydrophilic ligands.26 Therefore, the reduced relaxivity at a high loading mass ratio of MNP-PEG-Gd was likely caused by the aggregation and low surface area which decreased the exchange with water molecules.
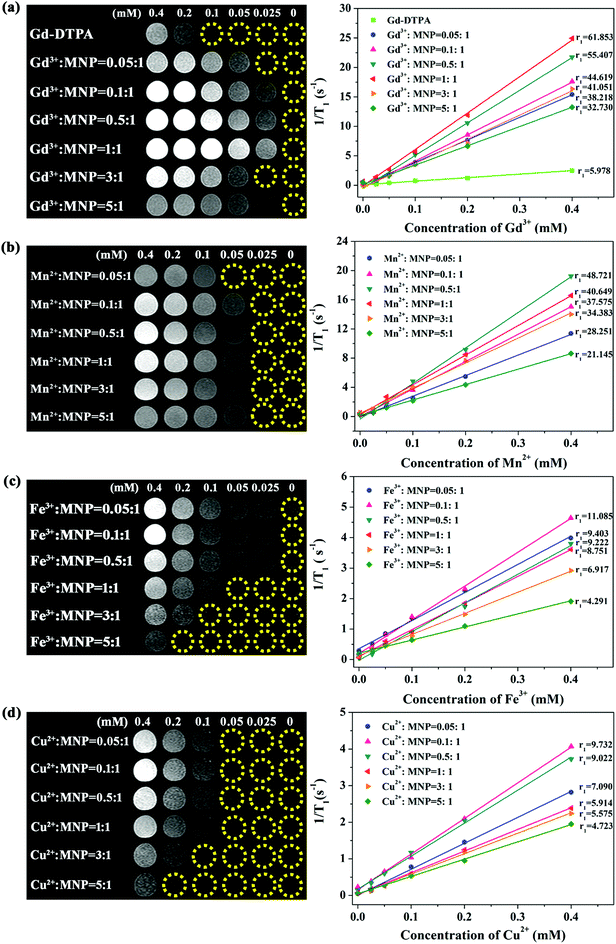 |
| Fig. 3 Comparison study of MRI performance in vitro. (a) T1-Weighted phantom images and relaxivity measurements of Gd-DTPA and MNP-PEG-Gd with different Gd3+ : MNP mass ratios. (b) T1-Weighted phantom images and relaxivity measurements of MNP-PEG-Mn with different Mn2+ : MNP mass ratios. (c) T1-Weighted phantom images and relaxivity measurements of MNP-PEG-Fe with different Fe3+ : MNP mass ratios. (d) T1-Weighted phantom images and relaxivity measurements of MNP-PEG-Cu with different Cu2+ : MNP mass ratios. The paramagnetic metal ion concentrations are indicated as 0.4, 0.2, 0.1, 0.5, 0.25 and 0 mM. | |
Longitudinal relaxivity (r1), reflecting the ability to shorten the T1 longitudinal relaxation time of water protons, is divided into two parts according to the Solomon–Bloembergen–Morgan (SBM) theory: one is inner sphere relaxation, which is influenced by directly coordinated water molecules and is the predominant effect on r1 relaxivity. For paramagnetic complexes in solution, inner-sphere relaxation mainly depends on both the number of sites on the metal available for coordination with water molecules and the rotational correlation time of the complex. The other part is outer sphere relaxation, which arises from the interaction of the complex with water molecules in the second and outer spheres.18,27 For melanin-based nanoparticles, the inner sphere relaxation was associated with the o-dihydroxyl group of the catechol unit, which has been proven to be much more important for the binding of metal ions and dominated the exchange with water molecules. In addition, water molecules can bind to oxygen atoms of the catecholate–metal ion complex to cause outer sphere relaxation in a hydrogen bonding manner, which leads to a further enhancement in r1 relaxivity.
The contrast enhancement capability of other metal ion (Mn2+, Fe3+ and Cu2+) chelated MNP-PEG is shown in Fig. 3b–d. The relaxivities of MNP-PEG-Mn, MNP-PEG-Fe, and MNP-PEG-Cu are noticeably higher than those of the clinical Gd-based small molecule contrast agents, indicating good detection sensitivity. The maximal relaxivity values were in the order Gd3+ > Mn2+ > Fe3+ > Cu2+ for the MNP-PEG-M complexes, with 48.7, 11.1 and 9.7 mM−1 s−1 for MNP-PEG-Mn, MNP-PEG-Fe and MNP-PEG-Cu, respectively. Like MNP-PEG-Gd, the relaxivities appeared increased firstly and then decreased with the increase in the mass ratio of metal ions to MNPs. However, the mass ratios that reach the maximum relaxivities were different among various metal ion chelated melanin nanoparticles. MNP-PEG-Mn, MNP-PEG-Fe and MNP-PEG-Cu exhibited the maximum relaxivities at the loading mass ratios of Mn2+
:
MNP = 0.5
:
1, Fe3+
:
MNP = 0.1
:
1 and Cu2+
:
MNP = 0.1
:
1, corresponding to 57, 54 and 51 chelated metals per MNP-PEG, respectively. Moreover, the maximal relaxivities were not matched to the maximal number of chelated metal ions except for MNP-PEG-Gd. There may be two reasons: (1) the bound metal ions were not only on the periphery but also inside the core of melanin nanoparticles. The number of metal ions bound on the periphery of nanoparticles is confined; thus as the bound metal ions increased, a large fraction of the metal ions (Fe3+ > Cu2+ > Mn2+) was bound deep within the biopolymer and was relatively inaccessible to solvent water molecules. (2) There may be magnetically coupled Fe(III)–Fe(III) or Cu(II)–Cu(II) interactions which can alter the nature of the relaxation, resulting in shifting from T1 toward T2-contrast agents.20
Therefore, the high signal intensity of the melanin-based material in T1-weighted MRI is grossly attributed to the chelation of melanin with paramagnetic metal ions Gd3+, Mn2+, Fe3+ and Cu2+. The above results indicated that there is an optimal mass ratio of various metal ions with MNP-PEG, which enables the best in vitro MRI performance.
Although the cell viability of MNP and MNP-PEG had already been confirmed,19 before in vivo application of four metal ion chelated melanin nanoparticles, rat H9c2 cardiomyocytes were employed to evaluate the cytotoxicity of MNP-PEG-M with various concentrations for 24 h using the standard CCK-8 assay (Fig. S4†). The results revealed no apparent acute cytotoxicity or morphological changes after incubation with four particles at levels up to 800 μg mL−1 for 24 h, demonstrating promising nontoxic applications to living systems.
3.3.
In vivo MRI
Inspired by the promising results in vitro, the efficacy of MNP-PEG-Gd, MNP-PEG-Mn, MNP-PEG-Fe and MNP-PEG-Cu for in vivo MRI imaging was evaluated with the 3.0 T clinical MRI equipment and the results are shown in Fig. 4. Mice were intravenously injected (n = 3 per compound) at a melanin concentration of 1 mg per mouse, and the coronal T1-weighted MR images as a function of time were acquired at appropriate time intervals (0 h, 0.5 h, 1 h, 2 h, 6 h, 24 h and 48 h) after injection. As demonstrated in Fig. 4a, a notably enhanced signal was achieved in the liver and kidneys after the injection of MNP-PEG-M at 0.5 h, perhaps because of the selective accumulation of the reticuloendothelial system and the clearance of ultrasmall nanoparticles through renal and hepatobiliary pathways. Impressively, the MR signals reached a maximum in the liver and kidneys at 1 h of administration of MNP-PEG-Gd, MNP-PEG-Mn and MNP-PEG-Fe and 2 h of administration of MNP-PEG-Cu. With time elapse, the MR signals of the liver and kidneys became weaker. After 24 h, the liver and kidneys seemed to return to a similar contrast to that before administration due to the clearance of nanoparticles. These results also indicated prolonged blood circulation and an enhanced imaging effect of the nano-contrast agent. Fig. 4b shows a side-by-side comparison of the MRI enhancement in tissues among the four MNP-PEG-M. The maximum MRI enhancement of MNP-PEG-Gd and MNP-PEG-Mn in tissues was significantly greater than that of MNP-PEG-Fe and MNP-PEG-Cu, indicated especially by the enhanced signals in the kidneys. The in vivo MRI enhancement ability was in agreement with the order of their r1 relaxivity values among the four MNP-PEG-M, with MNP-PEG-Gd and MNP-PEG-Mn showing much greater relaxivity (61.9 and 48.7 mM−1 s−1) than MNP-PEG-Fe and MNP-PEG-Cu (11.1 and 9.7 mM−1 s−1).
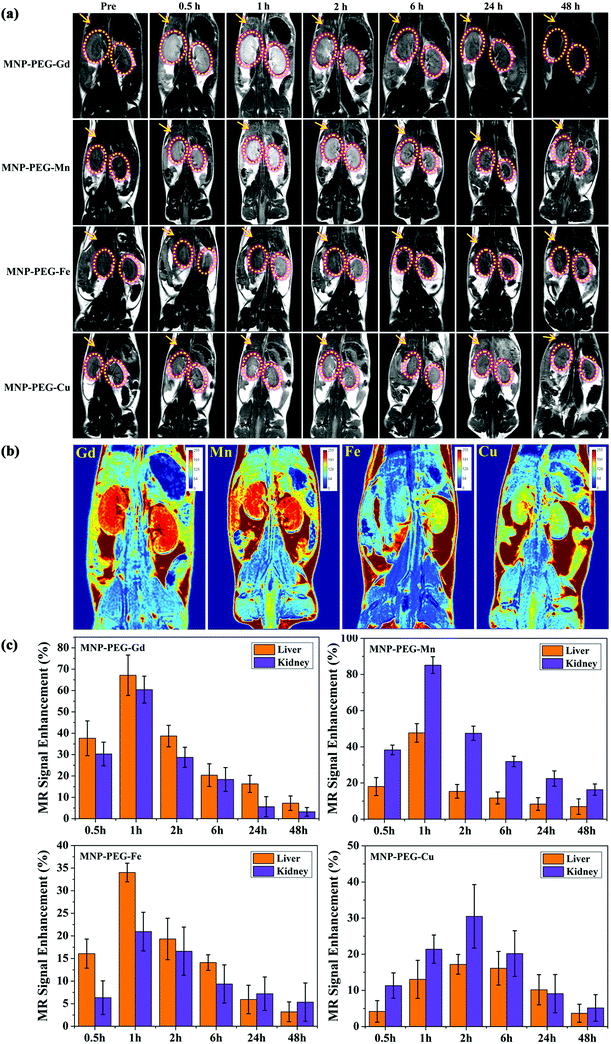 |
| Fig. 4 Comparison study of MRI performance in vivo: (a) representative T1-weighted MR images of mice at different time intervals of 0, 1, 2, 6, 24 and 48 h after intravenous administration of different metal ion chelated melanin nanoparticles. (b) Comparison of the maximum MRI enhancement of MNP-PEG-M. (c) MR signal enhancement (SE) of MNP-PEG-M at various time points. | |
To better understand the change of the MR signal and the metabolic behavior of the nanoparticles in vivo, the MR Signal Enhancement (SE) of the liver and kidneys was quantitatively analyzed and the fractions are shown in Fig. 4c. After injection, the SE values of the liver and kidneys in various metal ion chelated nanoparticles all increased to the maximum at 1 h or 2 h, and then decreased to the minimum at 48 h. The enhanced liver and renal accumulation is beneficial for liver and kidney imaging applications. In detail, the SE of MNP-PEG-Gd exhibited the maximum enhancement of about 65% in the liver and 60% in the kidneys. Impressively, an 84% enhancement in the kidneys for MNP-PEG-Mn was observed, and only a 50% SE in the liver. This can be attributed to MNP-PEG-Mn having an ultrasmall size of 3.4 nm, which could mainly be excreted by the kidneys. MNP-PEG-Fe and MNP-PEG-Cu had a lower SE due to their poor relaxivities. Moreover, it was found that the liver showed higher MRI SE values with MNP-PEG-Fe than the kidneys, suggesting that most of the nanoparticles were metabolized and cleared by the liver. MNP-PEG-Gd showed comparable MRI SE values in the liver and kidneys, with that of the liver being slightly higher. In contrast, the kidneys showed a higher MRI SE value with MNP-PEG-Mn and MNP-PEG-Cu than the liver, indicating that they were mainly excreted by the kidneys in agreement with previous reports.19,28,29 After 24 h, the SE of the liver and kidneys remarkably decreased, revealing that MNP-PEG-M could be completely excreted via renal and hepatobiliary pathways. In addition, the axial and coronal MR images of the bladder also showed higher brightness than that at pre-injection (Fig. S5†), showing another indication that the four nanoparticles were partly cleared through the kidney urinary excretion.
Therefore, the results of MR imaging in vivo suggested that various metal ion chelated melanin nanoparticles not only displayed excellent contrast performance but also could be highly efficiently excreted by both renal and hepatobiliary pathways, which reduced the potential toxic effect due to long-term accumulation of conventional nanoparticles in various organs and further enhanced the biosafety.
3.4.
In vivo biosafety
The biodistribution of metal ions was investigated in BALB/c mice (n = 3 per compound) 24 h after tail vein injection of MNP-PEG-M at the same concentration as in the MRI study and is shown in Fig. 5a. The mice were sacrificed and then the levels of metal ions were measured in main organs with ICP-MS. The result revealed that the nanoparticles were mainly cleared by the kidney and liver, and was consistent with the MRI results in vivo. Moreover, there were no noticeable abnormal behavior and weight change observed among those mice intravenously injected with MNP-PEG-M nanoparticles (Fig. 5b), suggesting favorable biosafety.
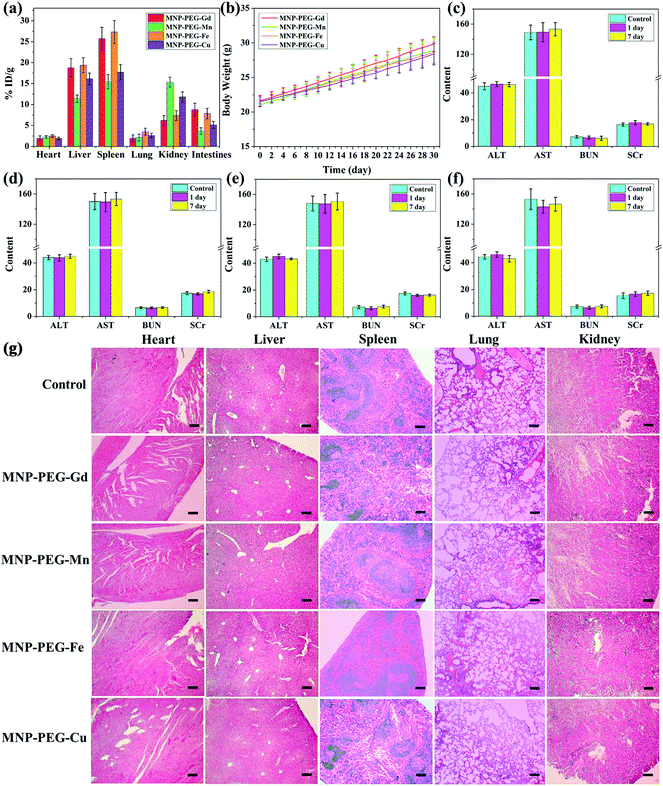 |
| Fig. 5 Biosafety of melanin chelated with various metal ions. (a) Biodistribution of MNP-PEG-M in BALB/c mice represented by the metal ion percentage in each tissue. Groups of mice (n = 3) were sacrificed at 24 h after tail vein injection and metal ion levels were measured in various organs with ICP-MS. (b) Body weight changes of mice (n = 3) 30 days after tail vein injection of MNP-PEG-M. Blood chemistry indexes (ALT, AST, BUN and SCr) of mice for the control, and 1 d and 7 d after treatment with MNP-PEG-Gd (c), MNP-PEG-Mn (d), MNP-PEG-Fe (e) and MNP-PEG-Cu (f). (g) Images of H&E-stained major organs (heart, liver, spleen, lung and kidney) from healthy control mice and MNP-PEG-M-injected mice at 24 h. The scale bar of the images of H&E-stained organs are 200 μm. | |
To investigate any potential toxic effect of the four nanoparticles, blood biochemistry examination was performed after injection at 1 d and 7 d (Fig. 5c–f). Blood biochemical tests can monitor the response to nanoparticle exposure and are widely used in disease diagnoses of the liver, kidney, etc.30 ALT and AST are crucial enzymes in the liver and are commonly used as 429 markers of liver disorders or injury. When the liver is dysfunctional, the levels of the aforementioned enzymes rise. The levels of ALT and AST were not significantly different from those of the control. Correspondingly, BUN and SCr are good indicators of kidney damage and 434 nephrotoxicity. No obvious increase was observed in the MNP-PEG-M group compared with that of the saline group. Then complete blood panel tests were performed for the systematic hematological assessment of MNP-PEG-M (Table S1†): white blood cells (WBC), red blood cells (RBC), hemoglobin (HGB), hematocrit (HCT), mean corpuscular volume (MCV), mean corpuscular hemoglobin (MCH), mean corpuscular hemoglobin concentration (MCHC) and platelets (PLT). The hematology parameters in the test groups exhibit no significant variation compared to the control group injected with saline. The above results showed that the four MNP-PEG-M nanoparticles had no obvious hepatic and renal toxicity at a dose of 1 mg MNP per mouse in 1 d and 7 d. This also suggested that our synthesized metal ion chelated melanin nanoparticles had good biosafety and negligible toxicity. The main organs (heart, liver, spleen, lungs, and kidneys) were analyzed by H&E staining to further confirm the biosafety of the MNP-PEG-M nanoparticles (Fig. 5g). The results indicated that the vital organs had no appreciable adverse effect and injury after treatment with the MNP-PEG-M nanoparticles. Therefore, the above results validated that MNP-PEG-M had an excellent biocompatibility and negligible toxicity, and they could be used as promising contrast agents for MRI imaging.
4. Conclusions
In summary, comparative studies were carried out for various paramagnetic metal ion chelated ultrasmall melanin nanoparticles as MRI contrast agents. In particular, the binding capacities and relaxivities were investigated by exploring the loading mass ratios of metal ions to melanin. The optimal MRI performance in vitro could be obtained at a fixed mass ratio. According to MRI in vivo, the nanoparticles exhibited notable signal enhancement and finally were efficiently eliminated through both renal and hepatobiliary pathways avoiding the potential toxicity due to their ultrasmall size. Besides, the nanoparticles had high chelation stability and biosafety. Therefore, the optimal MRI contrast performance was determined for melanin nanoparticles directly chelated with various metal ions, which provides some insight into potential translation of melanin nanoparticles as a new class of MRI contrast agents.
Conflicts of interest
The authors declare no competing financial interest.
Acknowledgements
This work has been financially supported by the National Natural Science Foundation of China (Grant No. 81801767, 81771907 and 81571747), Shanxi Province Science Foundation for Youths (No. 201701D221257), Science and technology innovation team project (No. 201705D131026), Engineering Technology Research Center of Shanxi Province No. 201805D121008, Scientific and technological achievements transformation project of Shanxi Province (No. 201704D131006), Laboratory Construction Project of Shanxi Province, the Projects for Local Science and Technology Development Guided by the Central Committee (YDZX20191400002537) and Startup Foundation for Doctors of Shanxi Medical University (No. 03201549).
References
- Y. Li, Y. Huang, Z. Wang, F. Carniato, Y. Xie, J. Patterson, M. P. Thompson, C. M. Andolina, T. B. Ditri, J. E. Millstone, J. S. Figueroa, J. D. Rinehart, M. Scadeng, M. Botta and N. C. Gianneschi, Small, 2016, 12, 668–677 CrossRef CAS.
- R. Chen, D. Ling, L. Zhao, S. Wang, Y. Liu, R. Bai, S. Baik, Y. Zhao, C. Chen and T. Hyeon, ACS Nano, 2015, 9, 12425–12435 CrossRef CAS.
- T.-H. Shin, Y. Choi, S. Kim and J. Cheon, Chem. Soc. Rev., 2015, 44, 4501–4516 RSC.
- X. Mao, J. Xu and H. Cui, WIREs Nanomed. Nanobiotechnol., 2016, 8, 814–841 CrossRef CAS.
- C. Guo, L. Sun, W. She, N. Li, L. Jiang, K. Luo, Q. Gong and Z. Gu, Polym. Chem., 2016, 7, 2531–2541 RSC.
- Y. Cao, L. Xu, Y. Kuang, D. Xiong and R. Pei, J. Mater. Chem. B, 2017, 5, 3431–3461 RSC.
- J. Wahsner, E. M. Gale, A. R. Rodríguez and P. Caravan, Chem. Rev., 2019, 119, 957–1057 CrossRef CAS.
- W. Zhang, L. Liu, H. Chen, K. Hu, I. Delahunty, S. Gao and J. Xie, Theranostics, 2018, 8, 2521–2548 CrossRef CAS.
- D. J. E. Huard, K. M. Kane and F. A. Tezcan, Nat. Chem. Biol., 2013, 9, 169–176 CrossRef CAS.
- Y. Lee, S. Lee, D. Y. Lee, B. Yu, W. Miao and S. Jon, Angew. Chem., Int. Ed., 2016, 55, 10676–10680 CrossRef CAS PubMed.
- Y. Liu, K. Ai and L. Lu, Chem. Rev., 2014, 114, 5057–5115 CrossRef CAS.
- R. Zhang, Q. Fan, M. Yang, K. Cheng, X. Lu, L. Zhang, W. Huang and Z. Cheng, Adv. Mater., 2015, 27, 5063–5069 CrossRef CAS.
- Y. Liu, K. Ai, X. Ji, D. Askhatova, R. Du, L. Lu and J. Shi, J. Am. Chem. Soc., 2017, 139, 856–862 CrossRef CAS.
- C. Qi, L. Fu, H. Xu, T. Wang, J. Lin and P. Huang, Sci. China: Chem., 2019, 62, 162–188 CrossRef CAS.
- D. J. Kim, K.-Y. Ju and J.-K. Lee, Bull. Korean Chem. Soc., 2012, 33, 3788–3792 CrossRef CAS.
- S. Cho, W. Park and D. H. Kim, ACS Appl. Mater. Interfaces, 2017, 9, 101–111 CrossRef CAS.
- Z. Wang, F. Carniato, Y. Xie, Y. Huang, Y. Li, S. He, N. Zang, J. D. Rinehart, M. Botta and N. C. Gianneschi, Small, 2017, 1701830 CrossRef.
- K. Y. Ju, J. W. Lee, G. H. Im, S. Lee, J. Pyo, S. B. Park, J. H. Lee and J. K. Lee, Biomacromolecules, 2013, 14, 3491–3497 CrossRef CAS.
- J. Sun, W. Xu, L. Li, B. Fan, X. Peng, B. Qu, L. Wang, T. Li, S. Li and R. Zhang, Nanoscale, 2018, 10, 10584–10595 RSC.
- Y. Li, Y. Xie, Z. Wang, N. Zang, F. Carniato, Y. Huang, C. M. Andolina, L. R. Parent, T. B. Ditri, E. D. Walter, M. Botta, J. D. Rinehart and N. C. Gianneschi, ACS Nano, 2016, 10, 10186–10194 CrossRef CAS.
- Q. Fan, K. Cheng, X. Hu, X. Ma, R. Zhang, M. Yang, X. Lu, L. Xing, W. Huang, S. S. Gambhir and Z. Cheng, J. Am. Chem. Soc., 2014, 136, 15185–15194 CrossRef CAS.
- L. Najder-Kozdrowska, B. Pilawa, A. B. Więckowski, E. Buszman and D. Wrześniok, Appl. Magn. Reson., 2009, 36, 81–88 CrossRef CAS.
- M. Zdybel, E. Chodurek and B. Pilawa, Appl. Magn. Reson., 2011, 40, 113–123 CrossRef CAS.
- P. M. Leal, S. Rivera-Fernandez, J. M. Franco, D. Pozo, J. M. De la Fuente and M. L. Garcia-Martin, Nanoscale, 2015, 7, 2050–2059 RSC.
- Q. Godechal, L. Mignion, O. Karroum, J. Magat, P. Danhier, R. Morandini, G. E. Ghanem, P. Leveque and B. Gallez, Contrast Media Mol. Imaging, 2014, 9, 154–160 CrossRef CAS.
- J. Sherwood, K. Lovas, M. Rich, Q. Yin, K. Lackey, M. S. Bolding and Y. Bao, Nanoscale, 2016, 8, 17506–17515 RSC.
- W. Scott Enochs, P. Petherick, A. Bogdanova, U. Mohr and R. Weissleder, Radiology, 1997, 204, 417–423 CrossRef.
- S. Zhang, Z. Chu, C. Yin, C. Zhang, G. Lin and Q. Li, J. Am. Chem. Soc., 2013, 135, 5709–5716 CrossRef CAS.
- M. Longmire, P. L. Choyke and H. Kobayashi, Nanomedicine, 2008, 3, 703–717 CrossRef CAS.
- X. Hao, X. Hu, C. Zhang, S. Chen, Z. Li, X. Yang, H. Liu, G. Jia, D. Liu, K. Ge, X. Liang and J. Zhang, ACS Nano, 2015, 9, 9614–9625 CrossRef CAS.
Footnotes |
† Electronic supplementary information (ESI) available: Fig. S1. EPR spectrum of prepared melanin nanoparticles. Fig. S2. T1-Weighted images of melanin nanoparticles with different concentrations. Fig. S3. TEM images of MNP-PEG-Gd with different Gd3+ : MNP mass ratios: (a) Gd3+ : MNP = 0.05 : 1, (b) Gd3+ : MNP = 0.1 : 1, (c) Gd3+ : MNP = 0.5 : 1, (d) Gd3+ : MNP = 1 : 1, (e) Gd3+ : MNP = 3 : 1 and (f) Gd3+ : MNP = 5 : 1. Fig. S4. Relative viabilities of Rat H9c2 cardiomyocytes incubated with MNP-PEG-M nanoparticles at various concentrations for 24 h by CCK-8 assay. Fig. S5. T1-Weighted MR axial and coronal images of BALB/c mice at 4 h after intravenously injecting MNP-PEG-M using 3.0 T clinical MRI scanning. Table S1. The hematological data after intravenous injection with either MNP-PEG-M or with saline (control). Error bars represent the standard deviation from 3 to 4 independent replicates. Reference ranges of hematology data of healthy Balb/c mice. See DOI: 10.1039/c9bm01580a |
‡ These authors contributed equally to this work. |
|
This journal is © The Royal Society of Chemistry 2020 |
Click here to see how this site uses Cookies. View our privacy policy here.