DOI:
10.1039/C9FO01628G
(Paper)
Food Funct., 2020,
11, 1087-1097
Digestion behavior and gastrointestinal fate of oil-in-water emulsions stabilized by different modified rice starches
Received
23rd July 2019
, Accepted 17th November 2019
First published on 11th December 2019
Abstract
Native rice starch was modified using different methods which included debranching (DB), octenyl succinylation (OSA), debranching followed by octenyl succinylation (DBOS) and octenyl succinylation followed by debranching (OSDB). The effect of different modifications and the impact of modified starch properties (resistant starch content (RS) and degree of substitution (DS)) on the gastrointestinal fate of emulsified lipids are elucidated using an in vitro digestion model that included the mouth, stomach, and small intestine phases in order to understand their functionality for further applications. The effect of the different modified rice starches on the particle size distribution of the lipid droplets, surface charge (ζ-potential), microstructure, lipid digestion (free fatty acid (FFA) release), and starch hydrolysis was also assessed. The OSA-modified starch and DBOS starch-based emulsions were found stable during the mouth phase and were also found to demonstrate lesser flocculation and coalescence in comparison with the other emulsions due to the presence of more OSA groups that provide greater steric hindrance and better protection from the gastrointestinal conditions. Furthermore, the DBOS starch was found to form emulsions that were more resistant to digestion with a degree of FFA release like dietary fibers and a lower extent of starch digestion that can be attributed to their higher resistant starch content (RS). Thus, the DBOS starch-based emulsions were found to be suitable for further applications such as developing functional foods to control satiety or for designing delivery systems for the sustained release of bioactive compounds.
1. Introduction
There has been a considerable interest in recent years for developing functional foods that can improve the health of consumers by exerting certain beneficial effects and reducing the risk of diseases associated with the high absorption of lipids and fats such as obesity and cardiovascular diseases (CVD).1,2 For this purpose, it is important to develop lipid-based colloidal delivery systems that can enhance the satiating properties of food and help reduce the blood serum triacylglycerol levels by releasing the lipids in a more controlled and sustained manner.3 It has been suggested by various studies that different types of dietary fibers can affect lipid behavior in the gastrointestinal tract (GIT) and can be used to delay or inhibit the digestion and absorption of lipids in the body.2,4 Dietary fibers refer to polysaccharides that are resistant to digestion in the small intestine and are fermented in the colon by colonic bacteria and they are known to include pectins, gums, cellulose, chitosan, and resistant starch (RS).5
Resistant starch (RS) refers to the starch fraction that is resistant to digestion by enzymes in the intestine following which it is degraded by microorganisms in the colon.6 Due to its poor digestion in the GIT, it functions as dietary fibers and has been receiving a lot of attention in recent years due to its wide range of health benefits that include prebiotic effects to maintain a healthy colonic microflora and prevention of colon cancer, diabetes, cardiovascular diseases, and obesity.7,8 Also, RS can replace the currently used synthetic and animal-based emulsifiers in the food industries due to an increased demand by the consumers for “clean label” products.9,10 Different approaches are generally employed for the preparation of RS that include physical, chemical, and enzymatic methods. Rice starch is known to be a potential emulsifier as it is known to consist of tiny granules with a narrow size distribution (2–8 μm).11 Hence, rice starch has been modified to produce RS with better functional properties for forming more stable oil-in-water emulsions.12 Furthermore, few studies have been conducted on different modified starch-based emulsions as potential approaches to delay intestinal lipolysis and design target delivery systems for the controlled release of bioactive compounds.13–15 The modified starches stabilize the emulsions mainly through steric repulsions that provide better stability to droplet coalescence due to changes in pH, ionic strength, and temperature. Furthermore, their denser structure and increased digestibility make them difficult to displace and provide greater resistance against the digestive enzymes that helps to slow down lipid digestion.7,14
In this study, rice starch was modified by different modifications that included debranching (DB), esterification with octenyl succinic anhydride (OSA), debranching followed by OSA modification (DBOS) and OSA modification followed by debranching (OSDB). The influence of these different modified starches as emulsifiers on the digestion behavior and gastrointestinal fate of oil-in-water emulsions was then evaluated using a simulated gastrointestinal model that mimics the mouth, stomach, and small intestine phases. The impact of the different modified rice starches on the digestion of lipid droplets was also analyzed. We hypothesized that the different modified rice starches will have different effects on lipid digestion due to their different degree of substitution (DS) and RS content. Furthermore, the structural and physicochemical properties (microstructure, particle size, and charge) of the different samples were measured as they passed through the different phases of the simulated GIT to determine key mechanisms by which the different starches influence lipid digestion. This information can lead to the design and implementation of functional foods for improved health and wellness. To our knowledge, this is the first study that reports the effect of different modified and resistant rice starch-based emulsions on lipid digestion under simulated gastrointestinal conditions.
2. Materials and methods
2.1. Materials
Commercially available native rice starch (NRS) was supplied by Cho Heng Rice Vermicelli Factory Co., Ltd, Nakhon Pathom, Thailand. The resistant starch assay kit was obtained from Megazyme International Ireland Limited, Wicklow, Ireland, which included pancreatic α-amylase, amyloglucosidase, and glucose oxidase/peroxidase (GOPOD) reagent enzymes. The main chemicals and enzymes used for the simulated gastrointestinal digestion model such as mucin from porcine stomach (M2378), pepsin from porcine gastric mucosa (P7000, 800–2500 units per mg), porcine pancreatin (P7545, 100–400 units per mg), and porcine bile extract (B8631) were purchased from Sigma Chemical Co., St Louis, MO, USA. As stated by the manufacturer, the bile extract comprised of 49% total bile salts containing a mixture of 10–15% glycodeoxycholic acid, 3–9% taurodeoxycholic acid, 0.5–7% deoxycholic acid, 1–5% hydrodeoxycholic acid, and 0.5–2% cholic acid. Sodium phosphate buffer (10 mM, pH 7.0) was used for preparing all the emulsions. Soybean oil was purchased from a local supermarket and was used without further purification steps. All other chemicals and reagents used in this work were of analytical grade. Double distilled water was used for all solution preparation.
2.2. Preparation of modified rice starch samples
The native rice starch (NRS) was subjected to different methods described previously to prepare different modified rice starches.12 Briefly, the NRS was suspended in 0.05 M sodium acetate buffer (pH 5.0) at a concentration of 10% (w/v) and gelatinized in a boiling water bath for 1 h. The resultant gel was cooled to 55 °C and hydrolyzed with pullulanase enzyme (45 units per g starch db) at 55 °C for 24 h. Enzyme inactivation was done by heating the starch slurry at 100 °C for 30 min followed by precipitation using 2 volumes of 95% ethanol. The precipitate obtained by centrifugation (1500g, 10 min) was then dried in a hot air oven (type UM 400, Memmert, Germany) at 45 °C for 24 h to get debranched rice starch (DB). The NRS and DB starches were then suspended in distilled water to prepare a 35% (w/w) starch slurry which was adjusted to pH 8.5 using 3% NaOH solution. The slurry was continuously stirred to which 3% (starch db) octenyl succinic anhydride (OSA) diluted thrice with isopropanol was added and the OSA modification reaction took place at 35 °C for 2 h. After that the slurry was adjusted to pH 6.0 using 1 M HCl, centrifuged (1500g, 10 min) and dried in an oven at 45 °C for 24 h. The NRS modified with OSA produced OSA starch and the DB starch modified with OSA produced the DBOS starch. OSA modified + debranched starch (OSDB) was prepared according to the methods described above for OSA modification and debranching where the NRS was first modified with OSA and then subjected to debranching. The resistant starch content of the native and all the modified rice starches was then determined using the Megazyme resistant starch assay kit according to the AACC approved method 32–40.16 This assay involves the use of enzymes (α-amylase and amyloglucosidase) to hydrolyze and solubilize the starch present after which the enzymes are inactivated using ethanol. The pellet collected is dissolved in potassium hydroxide which is hydrolyzed to D-glucose using amyloglucosidase that is quantified with the glucose oxidase/peroxidase reagent for the determination of the resistant starch content. Next, the degree of substitution (DS) of OSA modified starch samples was determined according to a previous study.12 It refers to the average number of hydroxyl groups that are substituted for every glucose unit and is determined using the titration method.17
2.3. Preparation of emulsions
Oil-in-water emulsions were prepared by homogenization of 10% (w/w) oil phase (soybean oil) and 90% (w/w) aqueous phase that comprised of the native (NRS) or modified rice starches, including DB, OSA, DBOS, and OSDB, (5% w/w) in buffer solution (10 mM sodium phosphate buffer, pH 7.0) as emulsifiers. A high-shear rotor-stator (model Ultra Turrax T18, IKA works, Inc., Wilmington, NC, USA) was used for blending the oil and aqueous phases for 5 min to produce coarse emulsions. The coarse emulsions were then passed through a two-stage high pressure homogenizer (type Panda, Niro-Soavi S.p.A, Parma, Italy) operating at first and second stage pressures of 400 and 40 bars, respectively for 3 passes in order to get fine emulsions.
For the NRS and OSA starch-based emulsions, the starches used for emulsion preparation were in their granular form with an intact granular structure. Hence, these two starches will adsorb on the oil–water interface in the form of solid particles and stabilize the emulsions by the Pickering mechanism. The Pickering mechanism is based on the adsorption of solid particles such as starch granules on the oil–water interface where they form steric barriers that help prevent droplet coalescence.18 On the other hand, for the DB, DBOS and OSDB starch-based emulsions, the starches used were subjected to gelatinization and enzymatic debranching during the modification process, resulting in the formation of dispersed and hydrolyzed starch molecules which stabilize the emulsions as molecules of dissolved starch.
2.4.
In vitro digestion model
The digestion behavior and gastrointestinal fate of the emulsions were monitored using a static simulated multi-stage GIT model that included the mouth, stomach, and small intestine phases. Each of the samples was passed through the different phases and physicochemical and microstructural measurements were carried out. Prior to subjecting the samples to in vitro digestion, the emulsion samples were diluted with 10 mM phosphate buffer (pH 7.0) at a ratio of 1
:
5 to obtain emulsions containing 2% oil content.
2.4.1. Mouth phase.
Artificial saliva solution (ASS) containing various salts and mucin (0.03 g mL−1) was prepared according to a previous study.19 The initial emulsion samples (20 mL) were mixed with 20 mL of ASS and the pH of the mixture was adjusted to 6.8 using NaOH/HCl solution. The mixture was then incubated at 37 °C for 5 min with continuous agitation at 100 rpm in a temperature-controlled water bath in order to mimic the conditions in the mouth.
2.4.2. Stomach phase.
Simulated gastric fluid (SGF) was prepared by adding 2 g of sodium chloride (NaCl) and 7 mL of concentrated hydrochloric acid (HCl) in 1 L of water.20 The bolus samples obtained from the mouth phase (20 mL) were then mixed with 20 mL of SGF containing 0.064 g of pepsin and the pH of the mixture was adjusted to 2.5 using NaOH/HCl solution. The mixture was then incubated at 37 °C for 2 h with continuous agitation at 100 rpm to mimic the stomach conditions.
2.4.3. Small intestine phase.
Samples obtained from the stomach phase (30 mL) were transferred into beakers in a water bath at 37 °C and their pH was adjusted to 7.0 using NaOH solution. Then, simulated intestinal fluid (SIF; 1.5 mL) containing 0.25 M CaCl2 and 3.75 M NaCl was added to the reaction vessel followed by the addition of 3.5 mL of bile extract solution (187.5 mg/3.5 mL) with continuous stirring and the pH of the system was readjusted to 7.0 using NaOH solution. Freshly prepared porcine pancreatin solution (2.5 mL, 60 mg/2.5 mL) was then added to the reaction vessel and a pH-stat automatic titration device (835 Titrando, Metrohm, USA Inc., Riverview, FL, USA) was then used to maintain the pH at 7.0 and to determine the volume of 0.1 M NaOH solution needed to maintain the pH at 7.0 and to neutralize the free fatty acids (FFAs) released following lipid digestion throughout the 2 h incubation period at 37 °C. The samples were taken for physicochemical and microstructural characterization at the end of the 2 h digestion period and the amount of FFAs released from the system was calculated by the following equation: | 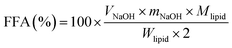 | (1) |
where VNaOH is the volume of NaOH (L) titrated to neutralize the FFAs generated, mNaOH is the molarity of the NaOH solution (0.1 M), Mlipid is the average molecular weight of soybean oil (920 g mol−1), and Wlipid is the total weight of oil in the digestion system (0.15 g).
2.5. Particle size determination
The particle size and particle size distribution of the samples were determined using a laser diffraction particle size analyzer (Mastersizer 2000, Malvern Instruments Ltd, Worcestershire, UK). The samples obtained from the mouth and small intestine phases were diluted with 10 mM phosphate buffer (pH 7.0) and the samples obtained from the stomach phase were diluted with double distilled water (pH 2.5) to avoid multiple scattering effects. Refractive indices of 1.46 and 1.33 for the oil and water phases respectively were used for the calculation of the particle size.12 The particle size was then reported as surface-weighted mean diameter (d32).
2.6. Confocal laser scanning microscopy
The microstructure of the emulsion samples at different stages in the GIT was characterized by confocal laser scanning microscopy (CLSM). The emulsions were stirred gently to make them homogeneous after which their oil phase was stained with Nile red dye (0.01% (w/v) in ethanol). A small aliquot of the stained emulsion samples was then transferred to a microscope slide and covered with a cover slip. A confocal laser scanning microscope with a 60× oil immersion objective lens (Fluoview FV10i-DOC, Olympus Corporation, Tokyo, Japan) was used to acquire the confocal micrographs. The excitation and emission wavelengths used for the Nile red were 488 nm and 605 nm, respectively.
2.7. ζ-Potential measurement
The ζ-potential of the emulsion droplets was measured using a particle electrophoresis instrument (Zetasizer Nano ZS, Malvern Instruments, Worcestershire, UK). Prior to measurement, the samples were diluted with appropriate buffer solutions to approximately 0.005% (w/w) droplet concentration and gently agitated to ensure their homogeneity. Each ζ-potential measurement was then reported as the average of two freshly prepared samples with two readings taken per sample.
2.8. Determination of starch digestion percentage
Aliquots of hydrolyzed starch solutions (1 mL) were taken following the intestinal digestion phase and mixed with 20 mL of 66% (v/v) ethanol to deactivate the enzymes. The glucose concentration of the starch hydrolysates was then measured using a glucose oxidase/peroxidase assay kit (Megazyme International, Wicklow, Ireland) and the percentage of starch hydrolyzed was determined by multiplying the glucose concentration by a factor of 0.9.
2.9. Statistical analysis
All experiments were carried out in duplicate for each sample except for the resistant starch content and the degree of substitution which were done in triplicate. The results are expressed as mean values ± standard deviations. The data were analyzed using one-way analysis of variance (ANOVA) using a statistical software package (SPSS, Version 16, Chicago, IL, USA). Duncan's multiple range test was employed to determine significance differences (p ≤ 0.05) among the samples.
3. Results and discussion
3.1. Resistant starch content and degree of substitution
In this study, rice starch was modified using different methods to enhance their functional, nutritional, and emulsifying properties. The resistant starch content of the native and the four differently modified rice starches is shown in Table 1. The highest resistant starch content was observed for the DB and DBOS starches that can be attributed to the enzymatic debranching step. This is because the debranching enzyme cleaves the α-1,6 linkages in amylopectin to form short linear molecules that form a new crystalline structure upon retrogradation which is resistant to digestion by the digestive enzymes.21–23
Table 1 Resistant starch content and degree of substitution of the native and different modified rice starchesa
Sampleb |
NRS |
DB |
OSA |
DBOS |
OSDB |
Values are given as means of triplicate determinations ± standard deviations. Values in the same row with different letters are statistically different (p ≤ 0.05).
NRS represents native rice starch; DB represents debranched rice starch; OSA represents OSA modified rice starch; DBOS represents debranched and OSA modified rice starch; OSDB represents OSA modified and debranched rice starch.
|
Resistant starch content (%) |
3.89 ± 0.65c |
34.96 ± 2.74a |
10.73 ± 1.81c |
35.80 ± 1.94a |
26.69 ± 1.98b |
Degree of substitution |
— |
— |
0.0138 ± 0.01b |
0.0195 ± 0.01a |
0.0110 ± 0.01c |
The degree of substitution of all OSA modified starches was also calculated to determine the efficiency of the OSA esterification reaction (Table 1). Interestingly, it was observed that the degree of substitution was significantly (p ≤ 0.05) higher for the DBOS starch than that for the other OSA modified starches which indicates that debranching prior to OSA esterification results in more substitution of OSA. This is in good agreement with previous studies which have reported that enzymatic treatment of native starch with a debranching enzyme results in the starch chains to be more in the dispersed form which allows the OSA to penetrate and bind the starch chains more easily and esterification takes place more efficiently.24,25
3.2. Influence of starch type on the digestion behavior and gastrointestinal fate of emulsion droplets
An in vitro GIT model was used to evaluate the impact of starch type used for coating the lipid droplets on the digestion behavior and gastrointestinal fate of starch-based oil-in-water emulsions. The mean particle size, particle size distribution, electrical charge, and microstructure of the emulsions were measured following their passage through each stage of the GIT to understand the physicochemical mechanisms through which the modified starches affect lipid digestion.
3.2.1. Initial systems.
Oil-in-water emulsions were prepared using the native and different modified rice starches and their surface-weighted mean droplet diameter (d32), ζ-potential, and microstructure were determined to corroborate their stability following a 24 h storage period. Despite the native and DB starches not possessing amphiphilic properties, they were able to adsorb at the oil–water interface and stabilize an emulsion to some extent as emulsifying capacity is known to be inversely related to the size of the starch granules.26 It has been previously reported that rice starch comprises tiny granules with a narrow size distribution (2–8 μm) making it a good candidate material as a particle stabilizer and emulsifier.11 Also, the contact angle of rice starch has been reported to be around 48°, indicating some amounts of hydrophobicity of the native rice starch granules.26 However, this is less than 90° which is the contact angle required for high emulsion stability which is one reason why the native and DB starches were not able to form stable emulsions. The NRS and DB starch based emulsions were found to demonstrate creaming and instability after 24 h of storage as mentioned in our previous study.12
Initially, the d32 values of NRS and DB starch-based emulsions were in the size range of 1.03–1.27 μm whereas all the OSA modified starch (OSA, DBOS, OSDB)-based emulsions had d32 values around 0.63–0.73 μm (Fig. 1). Also, all the OSA starch-based emulsions demonstrated a monomodal and narrow particle size distribution in comparison with the NRS and DB starch-based emulsions that demonstrated bimodal size distribution (Fig. 2). These results suggest that all the OSA modified starches were more effective in producing smaller emulsion droplets during the homogenization process by preventing coalescence. This can be attributed to OSA substitution that provides an amphiphilic nature resulting in a lower surface tension at the oil–water interface, increased viscosity, and steric hindrance that further improve the emulsifying capacity of starch.14,27 From all the OSA starch-based emulsions, the DBOS starch-based emulsions had the smallest droplet size that is due to a higher degree of OSA substitution in the DBOS starch (Table 1) in comparison with the other OSA modified starches. From the results obtained, it can be observed that the starch granules were able to produce smaller emulsion droplets than their apparent size which was found to be similar to a previous study.28 This can be attributed to the breaking of some of the starch granules during emulsification in the high pressure homogenizer which might have stabilized the oil droplets which were much smaller than the original size of the starch granules.28 Furthermore, the particle size and fitted distributions obtained for all the emulsions (Fig. 1 and 2, respectively) correspond to the scattering of the oil droplets rather than scattering from the starch population because a very high volume fraction of starch particles is required in comparison with the oil droplets in order to provide sufficient scattering intensity at which concentration they become severely aggregated to affect the scattering distribution.28
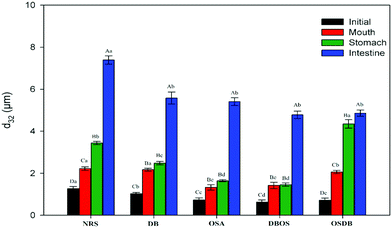 |
| Fig. 1 Influence of starch type on the mean droplet diameter (d32) of 10% oil-in-water emulsions stabilized by the native and different modified rice starches during different stages of in vitro digestion. Different capital letters indicate significant differences (p ≤ 0.05) in the droplet diameter of emulsions between different stages of the GIT using the same starch as the emulsifier. Different lowercase letters indicate significant differences (p ≤ 0.05) in the droplet diameter of emulsions stabilized by different starches in the same digestion stage. | |
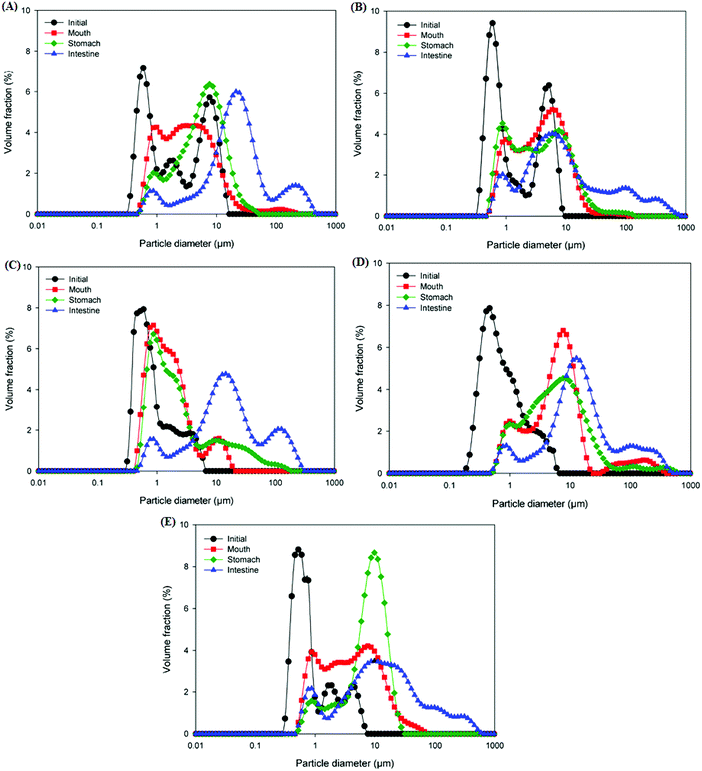 |
| Fig. 2 Influence of starch type on the particle size distribution of 10% oil-in-water emulsions stabilized by the native and different modified rice starches during different stages of in vitro digestion: (A) NRS; (B) DB; (C) OSA; (D) DBOS; (E) OSDB. | |
Furthermore, the microstructures of the emulsions obtained by confocal laser scanning microscopy (Fig. 3) helped to confirm that all the OSA starch-based emulsions had a uniform oil droplet distribution that was in accordance with the droplet size distribution data obtained by static light scattering. On the other hand, the NRS and DB starch-based emulsions were found to have lipid droplets with a broader size distribution with a range of large droplet sizes. Lastly, the magnitude of ζ-potential of all the starch-based emulsions was examined to understand the role of electrostatic repulsions on emulsion stability (Fig. 4). The OSA starch-based emulsions were found to have higher negative ζ-potential values than the NRS and DB starch-based emulsions which suggest a greater electrostatic repulsion between the droplets and better emulsion stability.29 The strong negative charge of these emulsions can be attributed to the presence of OSA groups on the surface of the modified starch.30 The emulsions prepared using NRS and DB starches were found to have negative ζ-potential values greater than −30 mV. The very large negative charge of the NRS and DB starch-based emulsions observed can be attributed to the presence of proteins and lipids on the surface of the starch granules.26 However, despite their large ζ-potential values, they were not able to form stable emulsions and demonstrated evidence of creaming and phase separation.12 This can be attributed to their larger oil droplet size which resulted in the formation of unstable emulsions with a higher creaming rate which was in accordance with the Stokes’ law stating that droplet size and density differences between the dispersed and aqueous phase can also affect the stability of an emulsion.31 A similar behaviour of starch-stabilized emulsions has been reported for rice, waxy maize and wheat starch.26
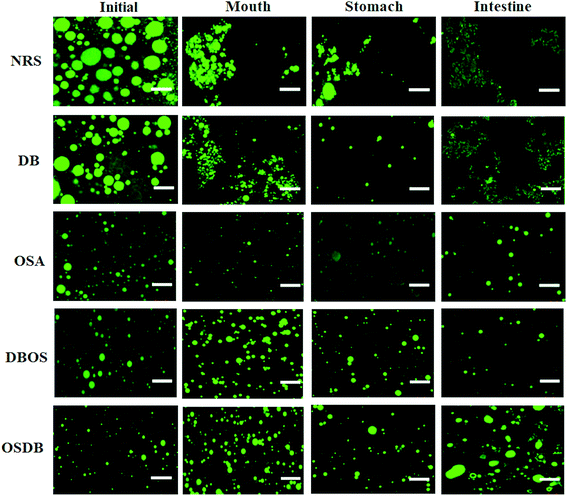 |
| Fig. 3 Influence of starch type on the microstructure (confocal fluorescence microscopy images) of 10% oil-in-water emulsions stabilized by the native and different modified rice starches during different stages of in vitro digestion. The scale bar is 10 μm. | |
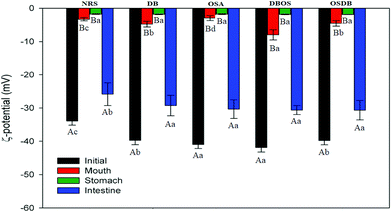 |
| Fig. 4 Influence of starch type on the ζ-potential of 10% oil-in-water emulsions stabilized by the native and different modified rice starches during different stages of in vitro digestion. Different capital letters indicate significant differences (p ≤ 0.05) in the ζ-potential of emulsions between different stages of the GIT using the same starch as the emulsifier. Different lowercase letters indicate significant differences (p ≤ 0.05) in the ζ-potential of emulsions stabilized by different starches in the same digestion stage. | |
3.2.2. Mouth phase.
An emulsion is a liquid colloidal system that can be rapidly swallowed in the mouth without any significant effect on the amylose and triacylglycerol as the residence time in the mouth is very small.3 Also, it is assumed that pancreatic amylase present in the small intestine is mainly responsible for the starch digestion due to which α-amylase in the mouth phase is generally omitted out.32 For this reason, α-amylase was not included in the artificial saliva solution in this study.
Following exposure to the simulated mouth phase, the changes observed were found to be dependent on the type of modified starch present. A significant increase (p ≤ 0.05) in the mean particle diameter was observed for all the emulsions tested (Fig. 1). All the emulsions exhibited multimodal particle size distributions (Fig. 2). The OSA and DBOS starch stabilized emulsions exhibited the smallest oil droplet size (p ≤ 0.05) as compared with the other emulsions. This can be attributed to their higher degree of OSA substitution (Table 1) that provides more resistance against changes in ionic strength in the mouth and aggregation similar to that reported in a previous study.14 It was further confirmed by the confocal microscopy images (Fig. 3) that there were some large droplet aggregates and network like flocculation present in the NRS and DB starch stabilized emulsions under the simulated mouth conditions and the OSA and DBOS starch-stabilized emulsions demonstrated comparatively lesser flocculation with smaller size droplets. This effect can be attributed to the presence of mucin, a large anionic biopolymer in artificial saliva that possesses both ionic and hydrophobic groups which can interact with the droplets and promote aggregation through depletion flocculation.4,33 Depletion flocculation is known to occur when relatively high amounts of non-adsorbed mucin is present in the aqueous phase. It has been reported that at low mucin concentrations (<1%, w/w), repulsive forces between two negatively charged biopolymers (in this case, starch and mucin) can successfully dominate the van der Waal's attractive forces between them. In this study, a mucin concentration of 1.5% (w/v) was used which is much higher than the concentration (0.5% w/v) at which the repulsive forces can no longer overcome the van der Waal's attractive forces, resulting in flocculation.19,34 Larger droplet aggregation was observed for the NRS and DB stabilized emulsions which can be attributed to their larger droplet size which makes them susceptible to depletion flocculation.35
Furthermore, a significant decrease (p ≤ 0.05) was observed in the magnitude of ζ-potential for all the emulsions after exposure to the mouth phase (Fig. 4) which can be attributed to the electrostatic screening effects due to the presence of mineral ions in the simulated saliva fluid or due to some mucin adsorption at the droplet surfaces.19,31 Mucin is known to have an overall negative charge of around −20 mV at neutral pH which is lesser than that of the starch stabilized emulsions and the less negative ζ-potential values of all emulsions following the mouth phase indicate some amount of mucin coverage on the droplet surface through electrostatic or hydrophobic interactions.19
3.2.3. Stomach phase.
Appreciable changes in the properties of the starch-based emulsions were observed after their exposure to the simulated gastric fluid where the nature and extent of these changes were found to depend mainly on the starch type. There was a marked increase (p ≤ 0.05) in the mean particle diameter for the emulsions stabilized by the NRS and OSDB starches, whereas those stabilized by the DB, OSA, and DBOS starches illustrated no significant changes (p > 0.05) in their droplet sizes (Fig. 1). The increase in particle sizes of NRS and DB emulsion systems following oil digestion can be attributed to aggregation of oil droplets due to oil digestion and changes in interfacial composition, lipid digestion products incorporated in mixed micelles, vesicles and colloidal structures and the formation of calcium soaps and complexes of calcium and bile acids.14 The particle size distributions of the emulsions stabilized by NRS, DB and OSDB starches exhibited bimodal and broad distributions with the OSDB starch stabilized emulsions demonstrating a large proportion of the oil droplets in the size range of 1–10 μm (Fig. 2A, B, and E, respectively). In contrast, the particle size distributions of emulsions stabilized by OSA and DBOS starches were found similar to their particle size distributions following the mouth phase (Fig. 2C and D, respectively). These emulsions were found to be more stable to flocculation and coalescence due to the amphiphilic nature of the starch with both hydrophilic and hydrophobic groups. The hydrophobic groups present help to decrease interfacial tension at the oil–water interface. Furthermore, the higher degree of OSA substitution to the starch chains results in more binding of the amphiphilic starch molecules to the surface of the oil droplets and an increased steric stabilization between them.12
In the confocal microscopy images (Fig. 3) of all starch-based emulsions after the gastric phase, the morphology of the aggregates was different. For all the emulsions, lesser flocculation was observed when they moved from the mouth phase to the stomach phase. This can be attributed to various physiochemical changes occurring in the stomach phase that may have reduced the attractive forces between the droplets. These include changes in pH and ionic strength that can alter the magnitude and strength of some electrostatic interactions between the droplets and dilution of the emulsion samples with simulated gastric fluid that reduces the concentration of non-adsorbed mucin in the aqueous phase resulting in reduced depletion flocculation.31,36,37 Furthermore, it is known that no enzymatic starch hydrolysis occurs during the gastric phase as the pepsin enzyme does not affect fats and carbohydrates which may have resulted in lesser droplet aggregation for all emulsions.38
The surface potential of all the emulsions following the gastric phase was also determined (Fig. 4) and it was observed that all the emulsions depicted a slight decrease in the magnitude of the ζ-potential. This may be attributed to electrostatic screening effects or due to the protonation of the carboxyl and amino groups that reduce the negative charge of the lipid droplets.39 A similar kind of behavior has already been reported for lipid droplets stabilized by different emulsifiers following gastric digestion.14,18,40
3.2.4. Intestine phase.
After passing through the small intestinal stage, all the emulsions were found to contain relatively large particles as observed by both static light scattering (Fig. 1) and confocal microscopy (Fig. 3). Particle size distributions of all the emulsions (Fig. 2) were found to be multimodal and small predominant peaks were observed for all the emulsions in the range of 0.7–3.0 μm. This may be attributed to small colloidal particles generated due to the digestion of triacylglycerol molecules such as mixed micelles, vesicles, and other colloidal structures.1,18,41 The microstructural behavior of the emulsions was analyzed by the confocal microscopy images (Fig. 3) which showed that the NRS and DB starch-stabilized emulsions underwent aggregation and flocs were present following the intestinal phase that can be attributed to high ionic strength, changes in interfacial composition and the effect of bile salts that can adsorb to the surface of the lipid droplets and contribute to droplet coalescence.1 From our hypothesis, the DB starch-stabilized emulsions should have demonstrated lesser aggregation owing to their high RS content that provides them resistance against the digestive enzymes. However, the emulsions formed using DB starch were found not to be stable initially prior to their passage through the gastrointestinal tract. This indicates that the interfacial layer contributed by the DB starch is not sufficiently rigid to arrest the destabilizing process of droplet coalescence and prevent the lipase enzyme from accessing the oil droplets to promote greater aggregation. Conversely, the microstructure of all the OSA starch-stabilized emulsions depicted a decrease in the number of visible oil droplets with the presence of some large oil droplets. A similar kind of behavior has been reported for emulsions stabilized by OSA modified waxy maize starch following intestinal digestion.14 This decrease was probably due to dilution of the emulsion samples when the simulated intestinal fluid was added to them, formation of calcium-bile salt complexes, triacylglycerol hydrolysis by lipase, and the incorporation of products obtained by lipid digestion within micelles, vesicles, and other colloidal particles.14,38,42,43 Lesser flocculation and coalescence in the only OSA modified starch stabilized emulsions can be attributed to their better stability against changes in pH, ionic strength and enzymes and their Pickering stabilization mechanism that provides a steric barrier around the oil droplets making them difficult to displace.18 Also, the high RS content of DBOS and OSDB starch stabilized emulsions may have provided them with a greater resistance to the digestive enzymes in the gastrointestinal tract. Also, they are known to form a protective coating around the lipid droplets that prevents adsorption by lipase or bile salts and they may also interact with the bile salts and calcium ions reducing their activity.5,34,44
Furthermore, all the emulsions were found to have a high negative surface potential following exposure to the small intestinal phase (Fig. 4) which can be attributed to the presence of a complex mixture of bile salts, phospholipids, and free fatty acids in the intestinal digesta that are anionic in nature. The results obtained indicate that the modified starch type had an impact on the particle size, microstructure, and electrical characteristics of the digesta obtained after the small intestine phase and the characteristics of the emulsions after they passed through each phase of the simulated gastrointestinal tract. Also, the initial size of the different modified starch stabilized emulsions was significantly different (Fig. 1) which may have somewhat influenced the lipid digestion in the emulsions.
3.3. Influence of starch type on lipid digestion
The influence of the native and different kinds of modified starch on the lipolysis profiles of the starch-stabilized emulsions was determined using a pH-stat method as shown in Fig. 5. The initial rate of lipid digestion was calculated from the slope of FFAs versus time in the first 5 min and the final digestion extent was determined from the average of the last 5 min of digestion. For all the samples, a rapid increase was observed in the amount of FFAs released during the first 5 min of digestion (Fig. 6A). It was observed that the lipid digestion profile depended mainly on the type of starch present in the emulsion system and pronounced differences were observed in the lipid digestion profiles of the different starch-stabilized emulsions. The final extent of lipid digestion was also determined (Fig. 6B) and it was found to be the highest for the NRS-based emulsions (87.80%) and lowest for the DBOS starch-based emulsions (36.37%), respectively. These results suggest that the extent of lipid digestion was influenced by the resistant starch content of the modified starches where a higher concentration of resistant starch resulted in a lower extent of lipid digestion. However, the lipid digestion rate for the DB starch-stabilized emulsions was found to be high despite their high resistant starch content which may be attributed to weaker adsorption of DB starch at the oil–water interface because the DB starch-stabilized emulsions were found to be unstable after a short period of time. This may have led to greater adsorption of lipase and bile salts on the surface of the oil droplets due to which they are unable to protect the lipid droplets from being digested by the enzymes. This indicates that lipid digestion is somewhat correlated with changes in emulsion properties following their passage through the mouth and stomach phases as the DB starch-stabilized emulsions were flocculated with droplet coalescence after the mouth and stomach phases which may have altered the interfacial layer surrounding the droplets.4 Hence, the extent of lipid digestion was found to be high for the DB starch-stabilized emulsions even when they were having a high resistant starch content.
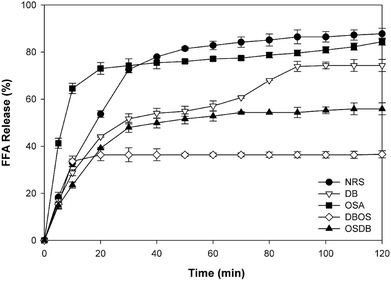 |
| Fig. 5 Influence of starch type on the free fatty acid (FFA) release from 10% oil-in-water emulsions stabilized by the native and different modified rice starches under simulated small intestine conditions. | |
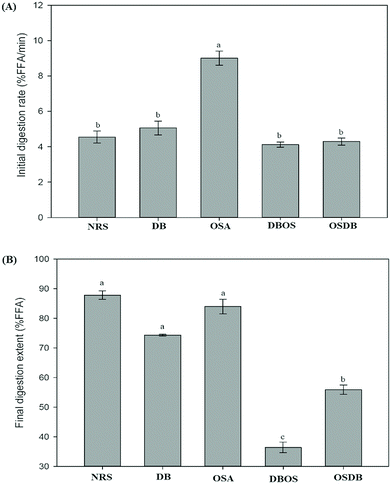 |
| Fig. 6 Initial lipid digestion rate (A) and final digestion extent (B) of 10% oil-in-water emulsions stabilized by the native and different modified rice starches under simulated small intestine conditions. Samples with different superscript letters are significantly different (p ≤ 0.05). | |
In principle, there are various structural and physicochemical mechanisms by which a high resistant starch content of the modified starch-stabilized emulsions can alter the process of lipid digestion. The presence of a higher concentration of resistant starch results in lesser hydrolysis of the starch molecules present at the oil–water interface and the formation of a protective layer around the lipid droplets that prevents the access of lipase and bile salts to the droplet surfaces.4,18 Furthermore, it has been reported by studies that the presence of more resistant starch molecules at the interface leads to increased viscosity that can increase the diffusion path length of lipase and inhibit their movement to the droplet surfaces and prevent the conversion of triacylglycerols to monoacylglycerols and FFAs.45
From the results obtained, it can be suggested that the DBOS starch-stabilized emulsions were able to reduce lipid digestion to a large extent in comparison with the other emulsions due to their high resistant starch content. Moreover, designing functional foods using these emulsions can be a good approach for increasing satiation and satiety and for combating obesity and many other chronic diseases.
3.4. Influence of starch type on starch digestion
Pancreatin added along with the simulated intestinal fluid in the small intestinal digestion stage is a combination of several digestive enzymes such as amylase, lipase and protease. The pancreatic amylase in the small intestine contributes to major starch digestion. The influence of starch type and resistant starch content on the digestion of starch in the emulsions following exposure to the simulated GIT system was studied (Fig. 7). The final extent of starch digestion was found to be the highest for the NRS starch-based emulsions (69.93%) and the lowest for the DBOS starch-based emulsion (19.34%) which is similar to the results obtained for lipid digestion. These results suggest that the final percentage of starch digestion reduced with an increase in the resistant starch content of the modified starches. This is because a higher resistant starch content provides a greater resistance to starch digestion by inhibiting the enzymatic degradation of starch which in turn helps to reduce the glycemic and insulinemic responses.46 The DB starch-stabilized emulsions despite their high resistant starch content were found to have a high amount of lipid digestion. However, starch digestion was low indicating that resistant starch molecules were present in the sample but were not strongly adsorbed to the interfacial layer.
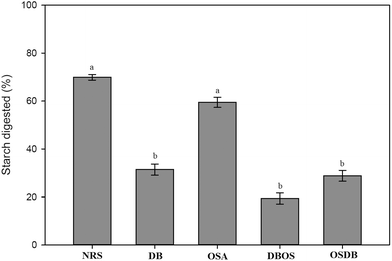 |
| Fig. 7 Influence of starch type on the digestion of starch in 10% oil-in-water emulsions stabilized by the native and different modified rice starches under simulated small intestine conditions. Samples with different superscript letters are significantly different (p ≤ 0.05). | |
4. Conclusions
In this study, the effect of the different modified rice starches on the digestion behavior of oil-in-water emulsions and their gastrointestinal fate was studied following their passage through the simulated GIT model. All starches were found to promote flocculation of the lipid droplets to some extent, however the NRS and DB starch-stabilized emulsions were found to have greater flocculation and droplet coalescence after incubation in the mouth and gastric phases in comparison with all the OSA modified starch-based emulsions. The higher emulsion stability of these emulsions due to OSA esterification may have contributed to their lesser droplet flocculation and coalescence. For lipid digestion, except for the DB starch-based emulsions, the starch molecules were found to decrease the extent of lipid digestion with an increasing resistant starch content. This may be because a higher resistant starch content provides greater resistance to hydrolysis by the digestive enzymes. Delayed digestion will help to slow down glucose absorption which will help promote satiety. These results may be beneficial for the design of emulsion-based functional foods using the modified rice starches with high resistant starch content that will help reduce glycemic index, control diabetes and obesity, and promote health and wellness.
Conflicts of interest
There are no conflicts of interest to declare.
Acknowledgements
The authors acknowledge Mahidol University, Thailand for the award of a Postdoctoral Fellowship (Contract No. MU-PD_2017_11) to the primary author Dr Surangna Jain and the financial support received for conducting research at Mahidol University, Thailand.
References
- F. A. Bellesi, M. J. Martinez, V. M. Pizones Ruiz-Henestrosa and A. M. R. Pilosof, Comparative behavior of protein or polysaccharide stabilized emulsion under in vitro gastrointestinal conditions, Food Hydrocolloids, 2016, 52, 47–56 CrossRef CAS.
- M. Espinal-Ruiz, L. P. Restrepo-Sánchez, C. E. Narváez-Cuenca and D. J. McClements, Impact of pectin properties on lipid digestion under simulated gastrointestinal conditions: Comparison of citrus and banana passion fruit (Passifloratripartita var. mollissima) pectins, Food Hydrocolloids, 2016, 52, 329–342 CrossRef CAS.
- D. J. McClements and Y. Li, Structured emulsion-based delivery systems: Controlling the digestion and release of lipophilic food components, Adv. Colloid Interface Sci., 2010, 159, 213–228 CrossRef CAS.
- D. Qin, X. Yang, S. Gao, J. Yao and D. J. McClements, Influence of dietary fibers on lipid digestion: Comparison of single-stage and multiple-stage gastrointestinal models, Food Hydrocolloids, 2017, 69, 382–392 CrossRef CAS.
- M. Espinal-Ruiz, F. Parada-Alfonso, L. P. Restrepo-Sánchez, C. E. Narváez-Cuenca and D. J. McClements, Impact of dietary fibers [methyl cellulose, chitosan, and pectin] on digestion of lipids under simulated gastrointestinal conditions, Food Funct., 2014, 5, 3083–3095 RSC.
- B. Zhang, J. Q. Mei, B. Chen and H. Chen, Digestibility, physicochemical and structural properties of octenyl succinic anhydride-modified cassava starches with different degree of substitution, Food Chem., 2017, 229, 136–141 CrossRef CAS.
- B. A. Ashwar, A. Gani, I. A. Wani, A. Shah, F. A. Masoodi and D. C. Saxena, Production of resistant starch from rice by dual autoclaving-retrogradation treatment: In vitro digestibility, thermal and structural characterization, Food Hydrocolloids, 2016, 56, 108–117 CrossRef CAS.
- A. Sharma and B. S. Yadav, Resistant starch: Physiological roles and food applications, Food Rev. Int., 2008, 24, 193–234 CrossRef CAS.
- C. E. Gumus, E. A. Decker and D. J. McClements, Gastrointestinal fate of emulsion-based ω-3 oil delivery systems stabilized by plant proteins: Lentil, pea, and faba bean proteins, J. Food Eng., 2017, 207, 90–98 CrossRef CAS.
- R. S. H. Lam and M. T. Nickerson, Food proteins: A review on their emulsifying properties using a structure–function approach, Food Chem., 2013, 141, 975–984 CrossRef CAS.
- X. Song, Y. Pei, M. Qiao, F. Ma, H. Ren and Q. Zhao, Preparation and characterization of Pickering emulsions stabilized by hydrophobic starch particles, Food Hydrocolloids, 2015, 45, 256–263 CrossRef CAS.
- S. Jain, T. Winuprasith and M. Suphantharika, Design and synthesis of modified and resistant starch-based oil-in-water emulsions, Food Hydrocolloids, 2019, 89, 153–162 CrossRef CAS.
- M. Jo, C. Ban, K. K. Goh and Y. J. Choi, Gastrointestinal digestion and stability of submicron-sized emulsions stabilized using waxy maize starch crystals, Food Hydrocolloids, 2018, 84, 343–352 CrossRef CAS.
- Q. Lin, R. Liang, F. Zhong, A. Ye and H. Singh, Effect of degree of octenyl succinic anhydride (OSA) substitution on the digestion of emulsions and the bioaccessibility of β-carotene in OSA-modified-starch-stabilized-emulsions, Food Hydrocolloids, 2018, 84, 303–312 CrossRef CAS.
- R. Liang, C. F. Shoemaker, X. Yang, F. Zhong and Q. Huang, Stability and bioaccessibility of β-carotene in nanoemulsions stabilized by modified starches, J. Agric. Food Chem., 2013, 61, 1249–1257 CrossRef CAS.
-
American Association of Cereal Chemists, Approved Methods of AACC, AACC, St. Paul, MN, 2000 Search PubMed.
- S. Jiang, L. Dai, Y. Qin, L. Xiong and Q. Sun, Preparation and characterization of octenyl succinic anhydride modified taro starch nanoparticles, PLoS One, 2016, 11, e0150043 CrossRef.
- T. Winuprasith, P. Khomein, W. Mitbumrung, M. Suphantharika, A. Nitithamyong and D. J. McClements, Encapsulation of vitamin D3 in Pickering emulsions stabilized
by nanofibrillated mangosteen cellulose: Impact on in vitro digestion and bioaccessibility, Food Hydrocolloids, 2018, 83, 153–164 CrossRef CAS.
- A. Sarkar, K. K. T. Goh and H. Singh, Colloidal stability and interactions of milk-protein-stabilized emulsions in an artificial saliva, Food Hydrocolloids, 2009a, 23, 1270–1278 Search PubMed.
- A. Sarkar, K. K. T. Goh, R. R. P. Singh and H. Singh, Behavior of an oil-in-water emulsion stabilized by β-lactoglobulin in an in vitro gastric model, Food Hydrocolloids, 2009b, 23, 1563–1569 Search PubMed.
- J. H. Lin and Y. H. Chang, Effects of type and concentration of polyols on the molecular structure of corn starch kneaded with pullulanase in a Farinograph, Food Hydrocolloids, 2006, 20, 340–347 CrossRef CAS.
- S. Ozturk, H. Koksel, K. Kahraman and P. K. W. Ng, Effect of debranching and heat treatments on formation and functional properties of resistant starch from high-amylose corn starches, Eur. Food Res. Technol., 2009, 229, 115–125 CrossRef CAS.
- M. Pratiwi, D. N. Faridah and H. N. Lioe, Structural changes to starch after acid hydrolysis, debranching, autoclaving-cooling cycles, and heat moisture treatment (HMT): A review, Starch/Staerke, 2018, 70, 1700028 CrossRef.
- N. Klaochanpong, S. Puncha-arnon, D. Uttapap, C. Puttanlek and V. Rungsardthong, Octenyl succinylation of granular and debranched waxy starches and their application in low-fat salad dressing, Food Hydrocolloids, 2017, 66, 296–306 CrossRef CAS.
- K. Lu, M. Miao, F. Ye, S. W. Cui, X. Li and B. Jiang, Impact of dual-enzyme treatment on the octenyl succinic anhydride esterification of soluble starch nanoparticle, Carbohydr. Polym., 2016, 147, 392–400 CrossRef CAS.
- C. Li, Y. Li, P. Sun and C. Yang, Pickering emulsions stabilized by native starch granules, Colloids Surf., A, 2013, 431, 142–149 CrossRef CAS.
- K. Królikowska, T. Fortuna, S. Pietrzyk and A. Gryszkin, Effect of modification of octenyl succinate starch with mineral elements on the stability and rheological properties of oil-in-water emulsions, Food Hydrocolloids, 2017, 66, 118–127 CrossRef.
- A. Yusoff and B. S. Murray, Modified starch granules as particle stabilizers of oil-in-water emulsions, Food Hydrocolloids, 2011, 25, 42–55 CrossRef CAS.
- E. Dickinson, Hydrocolloids as emulsifiers and emulsion stabilizers, Food Hydrocolloids, 2009, 23, 1473–1482 CrossRef CAS.
- M. Miao, R. Li, B. Jiang, S. W. Cui, T. Zhang and Z. Jin, Structure and physicochemical properties of octenyl succinic esters of sugary maize soluble starch and waxy maize starch, Food Chem., 2014, 151, 154–160 CrossRef CAS.
-
D. J. McClements, Food emulsions: Principles, practices, and techniques, CRC Press, Boca Raton, 2015 Search PubMed.
- S. Park, S. Mun and Y. R. Kin, Effect of xanthan gum on lipid digestion and bioaccessibility of β-carotene-loaded rice starch-based filled hydrogels, Food Res. Int., 2018, 105, 440–445 CrossRef CAS.
- M. H. Vingerhoeds, T. B. J. Blijdenstein, F. D. Zoet and G. A. van Aken, Emulsion flocculation induced by saliva and mucin, Food Hydrocolloids, 2005, 19, 915–922 CrossRef CAS.
- R. Zhang, Z. Zhang, H. Zhang, E. A. Decker and D. J. McClements, Influence of emulsifier type on gastrointestinal fate of oil-in-water emulsions containing anionic dietary fiber (pectin), Food Hydrocolloids, 2015, 45, 175–185 CrossRef CAS.
- R. Chanamai and D. J. McClements, Depletion flocculation of beverage emulsions by gum arabic and modified starch, J. Food Sci., 2001, 66, 457–463 CrossRef CAS.
- E. Dickinson, Hydrocolloids at interfaces and the influence on the properties of dispersed systems, Food Hydrocolloids, 2003, 17, 25–39 CrossRef CAS.
- L. Salvia-Trujillo, C. Qian, O. Martín-Belloso and D. J. McClements, Influence of particle size on lipid digestion and β-carotene bioaccessibility in emulsions and nanoemulsions, Food Chem., 2013, 141, 1472–1480 CrossRef CAS.
- J. Borreani, M. Espert, A. Salvador, T. Sanz, A. Quiles and I. Hernando, Oil-in-water emulsions stabilized by cellulose ethers: stability, structure and in vitro digestion, Food Funct., 2017, 8, 1547–1557 RSC.
-
J. N. Israelachvili, Intermolecular and surface forces, Academic Press, London, UK, 3rd edn, 2011 Search PubMed.
- B. Ozturk, S. Argin, M. Ozilgen and D. J. McClements, Formation and stabilization of nanoemulsion-based vitamin E delivery systems using natural biopolymers: Whey protein isolate and gum arabic, Food Chem., 2015, 188, 256–263 CrossRef CAS.
- D. J. McClements, E. A. Decker, Y. Park and J. Weiss, Structural design principles for delivery of bioactive components in nutraceuticals and functional foods, Crit. Rev. Food Sci. Nutr., 2009, 49, 577–606 CrossRef CAS.
- S. J. Hur, E. A. Decker and D. J. McClements, Influence of initial emulsifier type on microstructural changes occurring in emulsified lipids during in vitro digestion, Food Chem., 2009, 114, 253–262 CrossRef CAS.
- L. Zou, B. Zheng, W. Liu, C. Liu, H. Xiao and D. J. McClements, Enhancing nutraceutical bioavailability using excipient emulsions: Influence of lipid droplet size on solubility and bioaccessibility of powdered curcumin, J. Funct. Foods, 2015, 15, 72–83 CrossRef CAS.
- A. Torcello-Gómez and T. J. Foster, Interactions between cellulose ethers and a bile salt in the control of lipid digestion of lipid-based systems, Carbohydr. Polym., 2014, 113, 53–61 CrossRef.
- Y. Li, M. Hu, Y. Du, H. Xiao and D. J. McClements, Control of lipase digestibility of emulsified lipids by encapsulation within calcium alginate beads, Food Hydrocolloids, 2011, 25, 122–130 CrossRef CAS.
- E. Fuentes-Zaragoza, M. J. Riquelme-Navarrete, E. Sánchez-Zapata and J. A. Pérez-Álvarez, Resistant starch as functional ingredient: A review, Food Res. Int., 2010, 43, 931–942 CrossRef CAS.
|
This journal is © The Royal Society of Chemistry 2020 |
Click here to see how this site uses Cookies. View our privacy policy here.