DOI:
10.1039/C9FO01996K
(Paper)
Food Funct., 2020,
11, 1049-1062
Oligosaccharides from Gracilaria lemaneiformis better attenuated high fat diet-induced metabolic syndrome by promoting the Bacteroidales proliferation†
Received
29th August 2019
, Accepted 12th November 2019
First published on 13th November 2019
Abstract
Polysaccharides from Gracilaria lemaneiformis (GLPs) were shown to reduce high fat diet (HFD)-induced metabolic syndrome (MS), but its high molecular weight (MW) causes lots of barriers to its application in foods as a functional ingredient. In this study, oligosaccharides derived from GLPs (D-GLPs) were prepared, and its effect on HFD-induced MS and inflammation was evaluated in mice. It revealed that D-GLPs reduced fat accumulation, lipid disorders and inflammation in HFD-fed mice accompanied by an improvement of the gut microbiota. D-GLPs reversed HFD-induced gut microbiota dysbiosis characterized by the increased ratio of Bacteroidetes to Firmicutes and the abundance of the Bacteroidales. Spearman correlation analysis showed that some members of the Bacteroidales were positively correlated with reduced fat accumulation, inflammation and lipid disorders. Compared to GLPs, D-GLPs had a better inhibition effect on MS-related parameters, which may be attributed to higher abundance of some Bacteroidales members owing to their prioritization strategy to D-GLPs. The anti-obesity effect was transmissible through fecal transfer from D-GLPs-fed mice to HFD-fed mice, implying that the effect was associated with the gut microbiota. It indicated that D-GLPs can be used as a potential prebiotic agent to MS therapy.
1. Introduction
Metabolic syndrome (MS) is a condition characterized by a constellation of risk factors for cardiovascular disease and type 2 diabetes, ranging in prevalence from 10% to 40%.1,2 Increasing prevalence of MS has been a public health issue throughout the world. The main diagnostic components include reduced high density lipoprotein cholesterol (HDL-c), and raised triglyceride (TG) and fasting plasma glucose, all of which are closely related to body weight gain.3 MS occurs most often in populations of excessive nutrient intake and insufficient physical activity; however the metabolic and genetic susceptibilities are also critical factors.4 Pharmaceutical intervention is required for individuals with MS, but lifestyle changes are an effective low-cost strategy in the prevention of MS and a complement to drug therapy, e.g. high-fiber diets.5
The gut microbiota plays a critical role in the regulation of host metabolism and extraction of energy from ingested food. Accumulating evidence suggests that dysbiosis of the gut microbiota induced by high-fat/high-energy diets plays a critical role in the development of obesity, type 2 diabetes and other MS hallmarks.6,7 It is recognized that reduction in some beneficial bacteria, e.g. Bifidobacterium and increases in pathogenic bacteria, e.g. Desulfovibrionaceae are associated with obesity and its comorbidities.8–10 The evidence for a causal relationship between metabolic dysfunction and the gut microbiota comes from the studies that cohousing or antibiotics treatment can modify obesity and metabolic phenotypes in rodents.11,12 Therefore, it supports the concept that targeting dysbiotic gut microbiota can be an effective strategy for MS therapy.
Dietary fiber is a term that reflects a heterogeneous group of natural food sources, processed grains and commercial supplements that can be used as complementary or alternative agents in the management of manifestation of MS.13 Various polysaccharides from different sources have been shown to improve the gut microbiota community and increase the relative abundance of beneficial bacteria in humans and mice.14,15Gracilaria lemaneiformis has a long edible history in East Asian countries and contains a high content of polysaccharides mainly consisting of agaropectin and agarose, both of which have many bioactivities, e.g. antioxidant, hypolipidemic and antidiabetic activities.16,17 A previous study revealed that polysaccharides of G. lemaneiformis (GLPs) reduced fat accumulation and improved lipid metabolism in HFD-fed mice accompanied by an improvement of the gut microbiota.18 However, its high molecular weight (MW) causes lots of barriers to its application in foods as a functional ingredient.
Reduction in the degree of polymerization (DP) of a polysaccharide can improve its physical properties, e.g. viscosity and gel property and enhance its effect on host health.19 Some oligosaccharides can inhibit weight gain, improve lipid and glucose homeostasis, and decrease inflammatory markers and blood pressure in obese individuals and mice, e.g. oligofructose and galactooligosaccharide.20 Agar-oligosaccharides (AGOs) (2–4 DP) improved HFD-induced MS and gut dysbiosis, but their effect on the gut microbiota was clearly different from GLPs.18,21 It may be explained by the fact that the polymer length is a contributing factor for polysaccharide utilization and can determine the prioritization strategies to polysaccharides by gut microbes.22 Here, oligosaccharides from GLPs (D-GLPs, MW: 1.5 kDa) were prepared by acid hydrolysis, and their effect on HFD-induced MS and dysbiosis of the gut microbiota was evaluated in mice. It showed that D-GLPs reduced fat accumulation and inflammation and improved lipid metabolism in HFD-fed mice accompanied by an improvement of the gut microbiota. The anti-obesity effect was transmissible via fecal transfer from D-GLPs-fed mice to HFD-fed mice, implying the effect involved in the modulation of the gut microbiota. This study indicated that D-GLPs can be used as a potential prebiotic agent to MS therapy, and provides a theoretical basis for precise regulation of the gut microbiota.
2. Materials and methods
2.1 Materials
Dried G. lemaneiformis was purchased from XuFeng Aquatic Products Co., Ltd, and other chemical reagents were of reagent grade and provided by Shanghai Aladdin Bio-Chem Technology Co., Ltd.
2.2 Preparation of D-GLPs
GLPs was extracted according to the procedure previously described in ref. 17. Briefly, dried algae powder was extracted with 90-fold distilled water at 90 °C for 5 h. After centrifugation, the supernatants were concentrated in a vacuum rotary evaporator and precipitated by addition of 3 volumes of ethanol at 4 °C for 24 h. The precipitates were collected and dissolved in distilled water, and proteins were removed using the Sevag method.23 After vacuum freeze-drying, GLPs was hydrolyzed in 0.2 M HCl at 90 °C for 30 min and neutralized to pH 7.0 after cooling to room temperature.24 The end products were dialyzed against 3.5 kDa and 1 kDa dialysis membranes, and D-GLPs was collected after vacuum freeze drying (Fig. S1†).
2.3 Animal experiment
BALB/c mice (male, SPF) were purchased from Dalian Medical University and kept under controlled light conditions (12 h light–dark cycle) with free access to food and water. All animal procedures were performed in accordance with the Guidelines for Care and Use of Laboratory Animals of Dalian Medical University, and experiments were approved by the Animal Ethics Committee of Dalian Medical University (ID: LZ0160258). Eight-week-old mice were randomly divided into four groups: NC, HF, GLPs and D-GLPs (n = 7 per group, Fig. 1A), and fed with either a HFD (research diet 12451; HF, GLPs and D-GLPs) or normal chow diet (12450B; NC). Drinking water in the GLPs and D-GLPs groups was supplemented with 0.2% (w/v) of GLPs or D-GLPs from the 1st week, and the other two groups received normal water as the negative control. The feces of mice were collected individually at the 10th week and stored at −80 °C until sequencing analysis. After mice were sacrificed by cervical dislocation with sodium pentobarbital anesthesia, the liver, epididymal adipose tissue and colon were isolated and measured immediately.
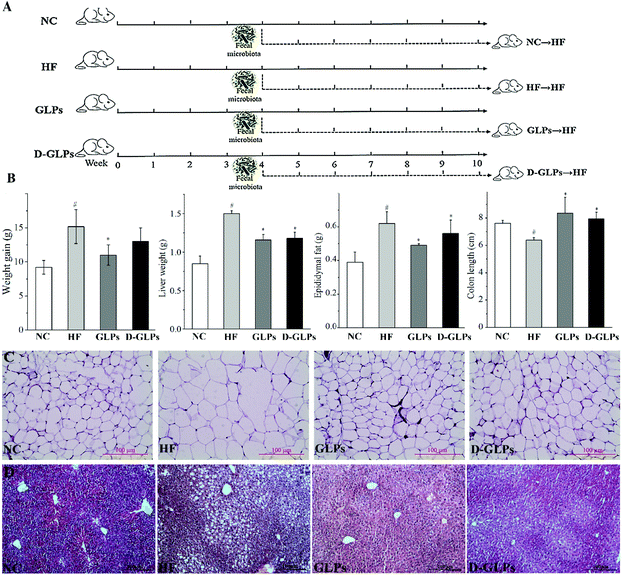 |
| Fig. 1 Effect of D-GLPs on fat accumulation in the organs and tissues of HFD-fed mice. The protocol of mice experiment (A). Weight gain, weights of liver and epididymal fat tissue and the colon length (B). Histology analysis of the liver and epididymal fat tissue (C). #p < 0.05 vs. the NC group and *p < 0.05 vs. the HF group. | |
2.4 Fecal transplant
Fecal transplant was performed based on an established protocol.25 As shown in Fig. 1A, eight-week-old mice were fed with a normal chow diet, HFD, HFD + GLPs and HFD + D-GLPs for ten weeks. After four weeks of feeding, feces were collected daily for the subsequent six weeks under a laminar flow hood under sterile conditions. Feces from donor mice of each group were pooled, and 0.1 g of feces was resuspended in 1 mL of sterile PBS solution. The solution was vigorously mixed for 30 s by using a vortex and centrifuged at 800g for 3 min. A fresh fecal suspension was prepared on the same day of transplant within 10 min before oral gavage to prevent changes in bacterial composition. The recipient mice (BALB/c, male, SPF, n = 6 per group) were fed with the HFD and inoculated daily with a fresh fecal suspension (150 μL per mouse) for six weeks, before being killed for subsequent analysis.
2.5 Biochemical analysis
The collected blood was centrifuged at 4 °C, 2000 rpm for 10 min to obtain serum, and the liver tissues were homogenized with a glass homogenizer. The levels of total cholesterol (TC), TG, catalase (CAT), malonaldehyde (MDA), HDL-c, total superoxide dismutase (T-SOD) and low-density lipoprotein-cholesterol (LDL-c) were measured by using commercialized kits according to the manufacturer's instructions (Nanjing Jiancheng, China).
2.6 Histology analysis
Freshly isolated epididymal adipose tissue and liver were fixed in 10% formalin overnight, followed by dehydration, embedding in paraffin and sectioning according to a standard protocol. Sections of 6 μm were stained with hematoxylin and eosin (H&E) and examined using a light microscope (Leica, Germany).
2.7 Quantitative reverse-transcription PCR
Total mRNA was isolated from tissues using Trizol® Reagent (Invitrogen, USA) and then reverse transcribed into cDNA with the FastQuant RT kit (KR116, TIANGEN, China) according to the manufacturer's instruction. Relative quantitative PCR was performed in a qTower real-time PCR system by using the Super Real Premix plus Kit (SYBR Green, FP205, TIANGEN, China). Changes in gene expression were calculated by using the 2−ΔΔCT method with β-actin as the housekeeping gene and normalized with the untreated control. The primers used in this study are shown in Table S1.†
2.8 High throughput sequencing analysis
Microbial DNA was extracted from mice feces using the E.Z.N.A® Soil DNA Kit (Omega, USA) in accordance with the manufacturer's protocol. The concentration and purification of DNA were determined using a NanoDrop spectrophotometer, and its quality was evaluated by electrophoresis on 1% agarose gel. The V3–V4 region of 16S rRNA gene was amplified with the primers 338F (5′-ACTCCTACGGGAGGCAGC AG-3′) and 806R (5′-GGACTACHVGGGTWTCTAAT-3′). The PCR products were extracted from a 2% agarose gel and purified using the AxyPrep DNA Gel Exaction Kit (Axygen, USA), and quantified by using QuantiFluor™-ST (Promega, USA). Purified amplicons were pooled in equimolar amounts and paired-end sequenced (2 × 300) on an Illumina MiSeq platform according to a standard protocol by Majorbio Bio-Pharm Technology Co., Ltd (Shanghai, China).
2.9 Bioinformatic analysis
Raw fastq files were quality-filtered using Trimmomatic and merged by FLASH. Operational taxonomic units (OTUs) were clustered with a 97% similarity cutoff using UPARSE with a greedy algorithm that performs chimera filtering and OTU clustering simultaneously. The taxonomy of the 16S rRNA gene sequence was analyzed by the RDP classifier algorithm against the Silva database using a confidence threshold of 70%. Alpha diversity included the Chao1, Ace and Shannon indexes, and beta diversity was visualized by weighted unifrac-based principal coordinate analysis (PCoA). Linear discriminant analysis coupled with effect size (LEfSe) was performed to identify the bacterial taxa differentially represented between groups at different taxonomic levels. Analysis of similarities (ANOSIM) was used to test for difference in the gut microbiota communities between groups.
2.10
In vitro fermentation model
Fresh fecal samples were collected from three healthy volunteers (22–23 years, no bowel disorders, no probiotics or antibiotics for 3 months) and dissolved in sterile 0.1 M PBS (20%, w/v) under anaerobic conditions. After filtration with four layers of sterile gauze, 1 mL of fecal suspension from each honor was added into 9 mL of gut microbiota medium (GMM, tryptone 2 g L−1, yeast extract 1 g L−1, L-cysteine 0.5 g L−1, meat extract 5 g L−1, KH2PO4 100 mM, MgSO4·7H2O 0.008 mM, NaHCO3 4.8 mM, NaCl 1.37 mM, CaCl2 4.8 mM, Vk1 5.8 mM, FeSO4 1.44 mM, histidine 5 mM, Tween-80 2 mL, vitamin mix (ATCC) 10 mL L−1, trace mineral m (ATCC) 10 mL L−1 and resazurin 4 mL L−1) containing 0.4% of GLPs or D-GLPs, and GMM without any carbon source was used as the negative control. After incubation at 37 °C for 72 h, the amounts of Bacteroides strains were determined on the BBE plate, and the carbohydrate contents in the broth were measured by the phenol-sulfuric acid method.
2.11 Analysis of Bacteroides strains on D-GLPs utilization
The ability of Bacteroides fragilis, Bacteroides ovatus, Bacteroides vulgatus and Parabacteroides distasonis on D-GLPs use was evaluated as previously described in ref. 26. For growth in chemically defined medium (DM, NH4Cl 1 g L−1, Na2HPO4 6 g L−1, KH2PO4 3 g L−1, NaCl 0.5 g L−1, CaCl2·2H2O 0.015 g L−1, MgSO4·7H2O 0.25 g L−1, L-cysteine 0.5 g L−1, hemin 0.02 g L−1, FeSO4·7H2O 0.004 g L−1, Vk1 0.0025 g L−1, VB12 0.005 g L−1 and D-GLPs 4 g L−1), Bacteroides strains were inoculated into the basal medium (BS, tryptone 20 g L−1, yeast extract 5 g L−1, NaCl 5 g L−1, glucose 5 g L−1, K2HPO3 5 g L−1, L-cysteine 0.5 g L−1, hemin 0.02 g L−1 and Vk1 0.0025 g L−1), cultured to the stationary phase and diluted 1
:
10 in fresh BS medium. After incubation at 37 °C for 24 h, bacteria in the mid-log phase were collected, washed twice with PBS and inoculated in DM with GLPs or D-GLPs. After incubation at 37 °C for 72 h, the amounts of Bacteroides strains were measured by the plate count method.
2.12 Measurement of short chain fatty acids (SCFAs)
SCFAs in mice feces were analyzed by GC as previously described in ref. 18. Briefly, 0.1 g of feces was homogenized in 0.5 mL of saturated NaCl solution, and acidified with 0.02 mL of dilute sulfuric acid (10%). 1 mL of diethyl ether was added to extract SCFAs, and the collected supernatants were reacted with 0.25 g of anhydrous Na2SO4 to remove the water inside. The levels of SCFAs were calculated according to a calibration curve. Nitrogen was supplied as carrier gas at a flow rate of 15 mL min−1, and the flow rates of air, hydrogen and nitrogen were 300, 30 and 30 mL min−1. The initial column temperature was maintained at 100 °C for 1 min, and then the temperature was increased to 200 °C at 5 °C min−1 and maintained at 200 °C for 5 min.
2.13 Statistical analysis
Data were expressed as mean ± standard deviation (SD) or box-and-whisker plots. Statistical analysis was carried out using one-way ANOVA followed by Duncan's test. Spearman correlation analysis was performed to evaluate the correlation between the key microbial phylotypes and obesity-related parameters. p < 0.05 was considered to be statistically significant.
3. Results and discussion
3.1 D-GLPs attenuated HFD-induced adverse effects on various organs of mice
Consumption of energy-dense/high-fat diets is strongly and positively associated with overweight that deteriorates insulin sensitivity, when excessive fat is located in the abdominal region. GLPs reduced weight gain and fat accumulation in the liver and adipose tissue of HFD-fed mice,18 which was consistent with other polysaccharides, e.g. Ganoderma lucidum.14 Compared to the HF group, D-GLPs led to significant reduction in liver weight, epididymal fat accumulation and lipid deposition in adipocytes and hepatocytes in HFD-fed mice, but no obvious difference was found in weight gain (p > 0.05) (Fig. 1B, C and D). A greater colon length can be observed in mice fed with dietary polysaccharides, whereas in other mice with experimentally-induced colitis or obesity, the colon length was reduced.27,28 D-GLPs increased the colon length in HFD-fed mice, which can be attributed to increased SCFA production from D-GLPs fermentation.29 No differences were observed between GLPs and D-GLPs in the liver, epididymal fat tissue and colon, indicating that D-GLPs can be used as a potential prebiotic agent to obesity and MS therapy.
3.2 D-GLPs improved HFD-induced dyslipidemia
A recent study has attributed the deleterious effect of HFD intake to its effect on lipoproteins and lipids, e.g. increases in LDL-c, TC and TG and reduction in HDL-c.30 In comparison with the NC group, the mice fed with a HFD were expected to be vulnerable to develop dyslipidemia characterized by increases in serum TC, TG and LDL-c and a reduction in serum HDL-c (Table 1). D-GLPs improved the serum lipid profile in HFD-fed mice by reducing TC, TG and LDL-c while elevating HDL-c, which was consistent with the fact that intake of AGOs reduced serum TC and TG in HFD-fed mice.31 Fucosylated chondroitin sulfate oligosaccharides alleviated HFD-induced lipid disorder by reducing lipid synthesis and promoting lipid lipidolysis.32 It should be noted that increased levels of TC and LDL-c accompanied by a low level of HDL-c are major risk factors for atherosclerosis and cardiovascular disease.33 HDL-c can play an essential role in the transport of cholesterol from extrahepatic tissues to liver, and a high concentration of LDL-c is regarded as a high-risk factor for atherosclerosis.34 It implied that D-GLPs can improve the anomalous change of serum lipid and prevent the development of MS.
Table 1 Effect of D-GLPs on the lipid profile and activities of antioxidant enzymes
|
NC |
HF |
GLPs |
D-GLPs |
The data within the same row followed by different superscript letters differ significantly (p < 0.05). |
Serum
|
TC (mmol L−1) |
3.64 ± 0.23c |
6.35 ± 0.37a |
4.92 ± 0.19b |
4.69 ± 0.21b |
TG (mmol L−1) |
1.36 ± 0.31b |
1.86 ± 0.23a |
1.02 ± 0.11c |
1.01 ± 0.07c |
LDL-c (mmol L−1) |
0.12 ± 0.01c |
0.43 ± 0.03a |
0.34 ± 0.01b |
0.30 ± 0.08bc |
HDL-c (mmol L−1) |
1.68 ± 0.08a |
1.20 ± 0.10b |
1.50 ± 0.20ab |
1.44 ± 0.19ab |
T-SOD (U mL−1) |
221.71 ± 18.94a |
167.73 ± 14.05a |
239.97 ± 27.96a |
250.86 ± 20.30a |
|
Liver
|
TC (μmol gprot−1) |
238.26 ± 16.45b |
477.81 ± 33.82a |
371.64 ± 36.28a |
224.17 ± 7.19b |
TG (μmol gprot−1) |
101.72 ± 3.81c |
212.60 ± 19.09a |
136.98 ± 23.31b |
61.49 ± 14.33d |
MDA(nmol mgprot−1) |
1.55 ± 0.12c |
2.38 ± 0.17a |
1.91 ± 0.24b |
2.28 ± 0.25a |
T-SOD (U mg−1) |
52.57 ± 1.31b |
46.77 ± 1.36c |
50.49 ± 0.74b |
71.49 ± 0.72a |
CAT (U g−1) |
799.35 ± 36.31a |
516.40 ± 17.86b |
634.76 ± 123.69ab |
919.69 ± 103.25a |
3.3 D-GLPs improved lipid metabolism and oxidative stress in liver
Non-alcoholic fatty liver disease (NAFLD) is a hepatic manifestation of obesity and MS and characterized by excessive lipid accumulation in the liver.35 The levels of TC, TG and MDA were markedly increased in the liver of HFD-fed mice, whereas D-GLPs reduced their levels (Table 1). Excessive lipid accumulation provides substrates for lipid peroxidation and oxidative stress in the liver, in which superoxide ions and hydroxyl ions can cause marked injuries to the surrounding tissues.36 Work on the pathogenesis of NAFLD indicates that the amelioration and prevention of oxidative stress can be an effective treatment of NAFLD. The activities of SOD and CAT that help to scavenge superoxide ions and hydroxyl ions were increased in D-GLPs-fed mice compared to the HF group. Notably, the levels of TC and TG were lower in the D-GLPs group than the GLPs group which was accompanied by higher activities of SOD and CAT. Polysaccharides from Lysium barbarum caused significant increases in the activities of enzymic antioxidants (SOD, CAT, etc.) and levels of non-enzymic antioxidants (GSH, Vc, etc.) in HFD-fed mice accompanied by an amelioration of oxidative stress and lipid oxidation.37 It indicated that D-GLPs can counteract the decline in the activities of antioxidant enzymes and thus attenuate free radical-induced lipid oxidation in obese individuals.
To elucidate the mechanisms of D-GLPs on fat accumulation, the mRNA levels of genes related to cholesterol metabolism were measured. Compared to the NC group, HFD feeding led to increases in the levels of 3-hydroxy-3-methylglutaryl-coenzyme A (HMG-CoA) reductase, Farnesoid X receptor (FXR) and LDL receptor (LDL-R) and a reduction in the level of Lecithin: cholesterol acyltransferase (LCAT) (Fig. 2). HMG-CoA reductase that leads to cholesterol biosynthesis was increased in the liver of obese patients with NAFLD and HFD-fed mice.38 FXR, as a multipurpose nuclear receptor, regulates expression of genes involved in bile acid homoeostasis, lipid metabolism and glucose balance.39 LCAT is an enzyme that is capable of HDL-c esterification, HDL maturation and reverse cholesterol transport.40 D-GLPs down-regulated the levels of HMG-CoA reductase and FXR and up-regulated the level of LCAT in HFD-fed mice, but no difference was observed in LDL-R. A previous study revealed that Baker's yeast glucan improved glucose and lipid homeostasis in the liver of ob/ob mice accompanied by changes in the expression of genes responsible for gluconeogenesis, cholesterol synthesis and fatty acid biosynthesis.41 It indicated that D-GLPs can improve lipid metabolism via modulation of expression of genes involved in cholesterol synthesis.
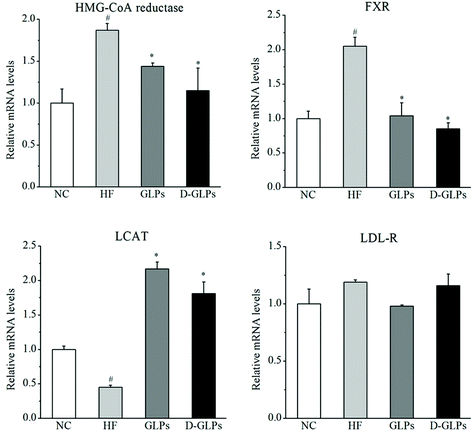 |
| Fig. 2 Effect of D-GLPs on the expression of genes related to cholesterol metabolism, HMG-CoA reductase (A), FXR (B), LCAT (C), and LDL-R (D). #p < 0.05 vs. the NC group and *p < 0.05 vs. the HF group. | |
3.4 D-GLPs attenuated HFD-induced chronic inflammation
HFD-fed obese mice can produce higher levels of pro-inflammatory cytokines and inflammatory markers in hepatic and intestinal tissues, such as tumor necrosis factor-α (TNF-α), interleukin-1β (IL-1β) and inducible nitric oxide synthase (iNOS).42,43 By contrast, production of anti-inflammatory cytokine IL-10 was detected to be reduced in obese animals.44 In this study, HFD feeding resulted in significant increases in TNF-α and iNOS and a reduction in IL-10 in the liver compared with the NC group, and no difference was found in IL-1β (Fig. 3A). D-GLPs decreased the levels of IL-1β, TNF-α and iNOS and increased IL-10 secretion in HFD-fed mice, indicating that D-GLPs attenuated HFD-induced chronic inflammation in mice. In addition, the expression of IL-1β and TNF-α was higher in colonic tissues of HFD-fed mice compared to normal chow-fed mice, whereas D-GLPs reduced their levels (Fig. 3B). It was consistent with the fact that polysaccharides of G. lucidum decreased the levels of pro-inflammatory cytokines (TNF-α, IL-1β, etc.) and promoted IL-10 secretion in HFD-fed mice.14 Compared with GLPs, D-GLPs had a better modulatory effect on the cytokine secretion profile in HFD-fed mice, implying that D-GLPs may better attenuate HFD-induced inflammation.
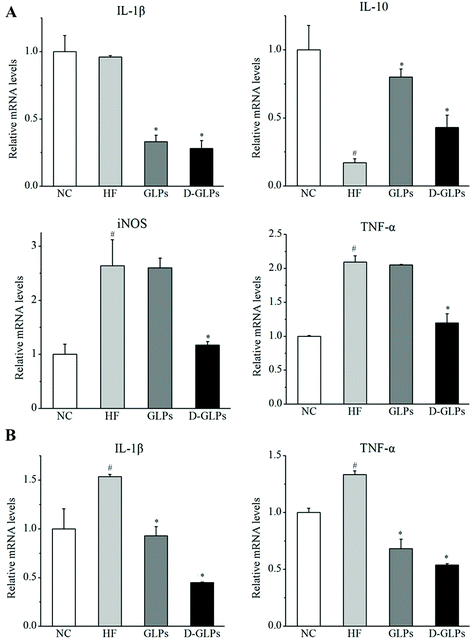 |
| Fig. 3 Effect of D-GLPs on the mRNA levels of pro-inflammatory cytokines, TNF-α, IL-1β, IL-6, and iNOS and anti-inflammatory cytokine IL-10 in liver (A) and colon (B). #p < 0.05 vs. the NC group and *p < 0.05 vs. the HF group. | |
3.5 D-GLPs reversed HFD-induced gut microbiota dysbiosis
The effect of D-GLPs on HFD-induced gut microbiota dysbiosis was analyzed by 16S rRNA sequencing. Compared with the NC group, the Ace and Chao1 indexes were lower in the HF group, but no difference was found in the Shannon index, implying that HFD feeding led to a reduction in species richness (Fig. 4A). D-GLPs up-regulated the Ace and Chao1 indexes, suggesting that it can increase gut microbiota richness. Then, the relationships between the gut microbiota communities of groups were evaluated by weighted unifrac-based PCoA (Fig. 4B). It revealed a distinct clustering of microbiota between the NC and HF groups (r = 0.86, p = 0.01), HF and GLPs groups (r = 0.23, p = 0.07), and HF and D-GLPs groups (i = 0.20, p = 0.07), and no difference was found between the GLPs and D-GLPs groups (r = −0.12, p = 0.83). Typing analysis revealed that the gut microbiota of groups can be assigned to two distinct types, and the NC and HF groups were significantly separated (Fig. 4C). The gut microbiota of both D-GLPs and GLPs groups was closer to T1 type characterized by normal gut microbiota. It indicated that HFD-induced gut microbiota dysbiosis was improved by D-GLPs as well as GLPs, and no differences were found on the overall structure of the gut microbiota.
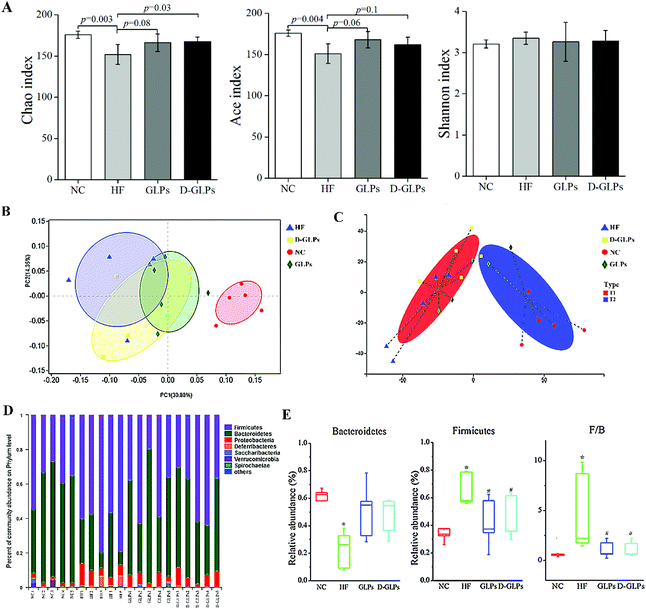 |
| Fig. 4 Effect of D-GLPs on the gut microbiota was analyzed by 16S rRNA sequencing (number of effective reads per sample >40 000). The species richness and richness of gut microbiota were evaluated by the Ace, Chao1 and Shannon index (A). Differences between groups were evaluated by PCoA (B) and typing analysis (C). Taxonomic profiling was performed to display changes in the gut microbiota at the phylum level (D and E). #p < 0.05 vs. the NC group and *p < 0.05 vs. the HF group. | |
The gut microbiota is composed of trillions of bacteria, with more than 90% of the members belonging to two phyla: Bacteroidetes and Firmicutes. The balance between them is considered to be associated with obesity, because these two phyla have different efficiency on energy extraction and possess different effect on host metabolism of the absorbed calories.45 The gut microbiota of diets-induced obese mice is characterized by an increased ratio of Firmicutes to Bacteroidetes and an elevated endotoxin-producing Proteobacteria. Taxonomic profiling revealed that D-GLPs treatment reduced the ratio of Firmicutes to Bacteroidetes in HFD-fed mice to level similar to that of the NC group, and no differences were found between D-GLPs and GLPs (Fig. 4D and E). This was consistent with previous studies that polysaccharides reduced HFD-induced obesity and MS accompanied with an improved intestinal micro-ecological balance.14,18,19
LEfSe was used to identify the different abundant taxa of bacteria that respond to D-GLPs, which emphasizes both statistical significance and biological relevance. HFD feeding led to increases in the Lachnospiraceae and Ruminococcaceae family and some members of the Proteobacteria and Deferribacteres and a reduction in the Bacteroidales within the Bacteroidetes (Fig. 5A). GLPs increased relative abundances of the Alistipes, Prevotellaceae_UCG_001_group, and Parabacteroides (Bacteroidales order) in HFD-fed mice (Fig. 5B). The effect of D-GLPs on the gut microbiota was similar to GLPs, but it better promoted some Bacteroidales members proliferation, e.g. Bacteroides and Paraprevotella (Fig. 5C and D). This was different from that AGOs attenuated HFD-induced MS and gut dysbiosis, in which AGOs led to increases in some Lactobacillales members, but no obvious differences were found in the Bacteroides and Prevotella.21 The above differences may be caused by the polymer length, which can determine the prioritization strategies to polysaccharides by gut microbes.22
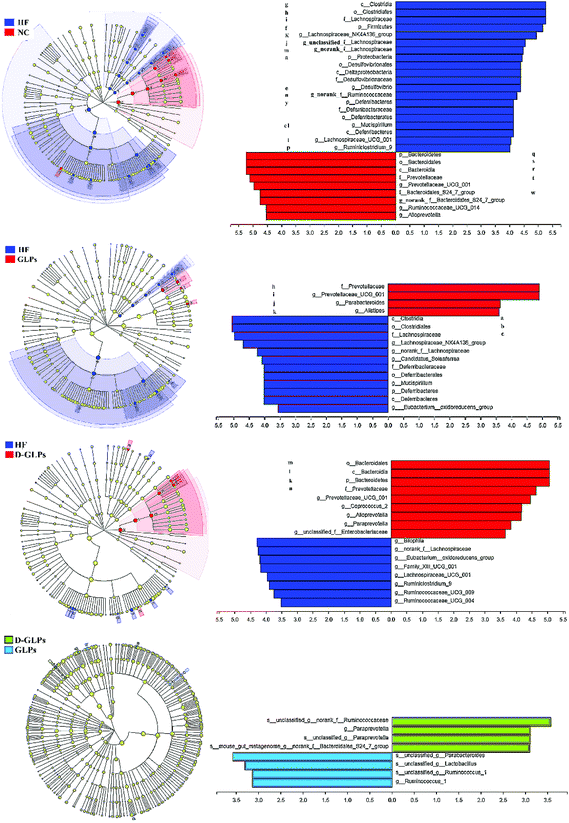 |
| Fig. 5 LEfSe was performed to identify the different abundant taxa of bacteria between groups at the different taxonomic levels. | |
Accumulating studies showed that various polysaccharides had many activities in humans and mice accompanied by an increase in some Bacteroidales members.14,26,46 The Bacteroidales is one order that consists of closely related members, e.g. Prevotella, Bacteroides and Parabacteroides. Individual species of the Bacteroidales are abundant and stable over the lifetime and across generations of humans owing to the genetically encoded polysaccharide-degradation ability.47 The in vitro model revealed that the amounts of Bacteroides strains were higher in D-GLPs-treated microbiota than GLPs, and the carbohydrate contents were lower in the former (Fig. 6A), indicating that D-GLPs was better utilized by gut microbes. To further elucidate the effect of the polymer length on Bacteroides strains, the growth properties of B. ovatus, B. fragilis, B. vulgatus and P. goldsteinii were evaluated (Fig. 6B). The result showed that B. ovatus, B. fragilis and B. vulgatus were able to live with GLPs, and the levels of B. ovatus and B. fragilis were higher in DM with D-GLPs. Notably, P. goldsteinii that are unable to grow on GLPs can live with D-GLPs. Some Bacteroides members that are unable to grow on a particular polysaccharide can utilize its breakdown products from growth of other bacteria that are able to utilize that polysaccharide.26 It implied that proper reduction in the DP of GLPs can better promote the proliferation of Bacteroides strains within the gut microbiota.
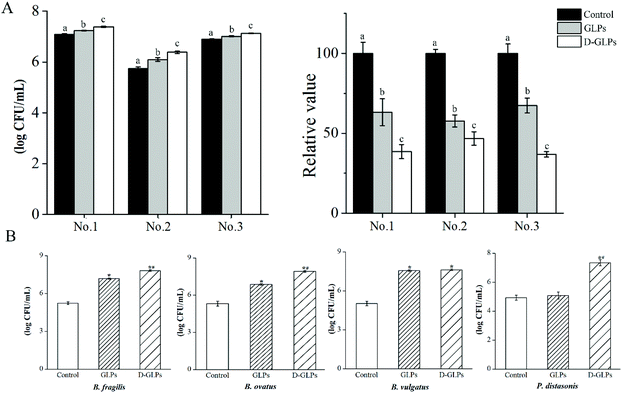 |
| Fig. 6 Effect of D-GLPs on Bacteroides strains within gut microbiota. The contents of Bacteroides strains were measured after D-GLPs fermentation, and the carbohydrate contents was determined by the phenol-sulfuric acid method (A). Data followed by different superscript letters differ significantly. Differences between D-GLPs and GLPs on B. ovatus, B. fragilis, B. vulgatus and P. goldsteinii were determined by sing-strain culture (B). *p < 0.05 vs. the control group and #p < 0.05 vs. the GLPs group. | |
Spearman correlation analysis revealed that the Prevotellaceae_UCG_001_group, Paraprevotella and Alistipes were positively correlated with IL-10, SCFAs, HDL-c and colon length, and negatively correlated with TC, TG, LDL-c, weights of epididymal fat tissue and liver, HMG-CoA reductase, LDL-R, FXR, IL-1β and TNF-α (Fig. 7). Some bacteria showed to some extent positive correlations with the improvement of inflammation and MS, e.g. some Bacteroidales_S24-7_group members, Rikenellaceae_RC9_gut_group and Bacteroides. A previous study revealed that the Prevotellaceae_UCG_001_group had a negative correlation with the liver index and TC in HFD-fed mice; however Alistipes was positively correlated with these parameters.48 Another study showed that Bacteroides, Paraprevotella and Parabacteroides were obviously increased in atorvastatin-treated HFD mice compared to the HFD group.49 The abundances of Bacteroides species in the gut were depleted in the obese microbiome, and exhibited a considerable increase in obese individuals following weight-loss intervention.50 These results indicated that the Bacteroidales played an important role in HFD-induced MS, and D-GLPs can be used for MS therapy by increasing the abundances of some Bacteroidales members. Moreover, relative abundances of some bacteria that were positively correlated with inflammation and MS were decreased in D-GLPs-fed mice, e.g. Desulfovibrio and Intestinimonas.51
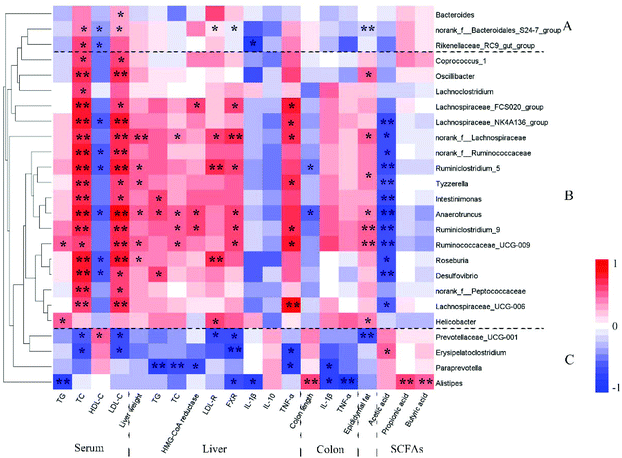 |
| Fig. 7 Spearman correlation analysis was used to calculate the correlation between the key microbial phylotypes and obesity-related traits. *p < 0.05 and **p < 0.01. | |
3.6 D-GLPs increased SCFA production in HFD-fed mice
SCFAs, as the end products of fermentation of dietary fiber by the gut microbiota, have been shown to exert many beneficial effects on host energy metabolism, which is regulated by many different host, environmental, dietary and microbial factors.52 HFD feeding was shown to reduce the production of SCFAs (acetate, propionate and butyrate) in mice accompanied by increased inflammation and fat accumulation.53 As shown in Fig. 8A, both D-GLPs and GLPs increased SCFAs (acetate, propionate and butyrate) production in HFD-fed mice as well as other polysaccharides,18,54 and a higher content of acetate was observed in the former, indicating that D-GLPs can be better utilized by gut microbes. SCFAs contribute to a normal large bowel function and prevent pathology through their action in the lumen and on the colonic musculature and vasculature and through their metabolism by colonocytes. SCFAs can enter the systemic circulation and directly influence metabolism and function of peripheral tissues.55 SCFAs differ in their fate and tissue, with butyrate being used preferentially as an energy source by the gut mucosa, propionate contributing to gluconeogenesis in the liver and acetate achieving the highest systemic concentration in blood.56 Supplementation with acetate, propionate or butyrate was shown to inhibit weight gain in HFD-fed mice, which was associated with SCFA-mediated activation of G-protein coupled receptor (GPR) 41 and GPR43 in the adipose tissue.57 It suggested that D-GLPs can maintain metabolic homoeostasis via increased SCFA production from anaerobic fermentation of D-GLPs.
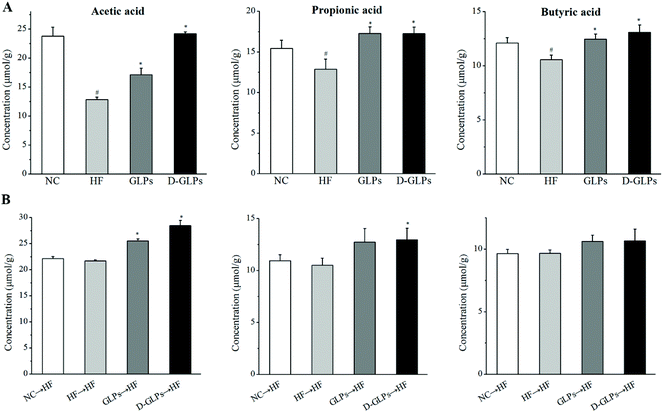 |
| Fig. 8 Effect of D-GLPs (A) and fecal transfer from D-GLPs-treated mice (B) on SCFAs production in HFD-fed mice. #p < 0.05 vs. the NC group and *p < 0.05 vs. the HF group. | |
3.7 D-GLPs fecal transplant prevented development of HFD-induced obesity
Transfer of the gut microbiota from obese individuals to HFD-fed mice can convey larger weight gain and higher levels of obesity-associated metabolic disorders than fecal transfer from the individual's lean twin.58 To determine whether the gut microbiota of D-GLPs-fed mice improved the condition of HFD-fed mice, the gut microbiota from D-GLPs-fed mice was transferred to HFD-fed mice. As shown in Fig. 9, fecal transfer from D-GLPs-fed mice reduced weight gain, fat accumulation and liver weight in HFD-fed mice compared to fecal transfer from HFD-fed mice. Compared with the NC → HF group, fecal transfer from D-GLPs-fed mice produced more robust effects on obesity-related traits, and no obvious differences were found between D-GLPs and GLPs. Fecal transfer from G. lucidum polysaccharides-fed mice reduced fat accumulation, weight gain and inflammation in HFD-fed mice.14 It indicated that the weight-lowering effect of D-GLPs was associated with the modulation of the gut microbiota.
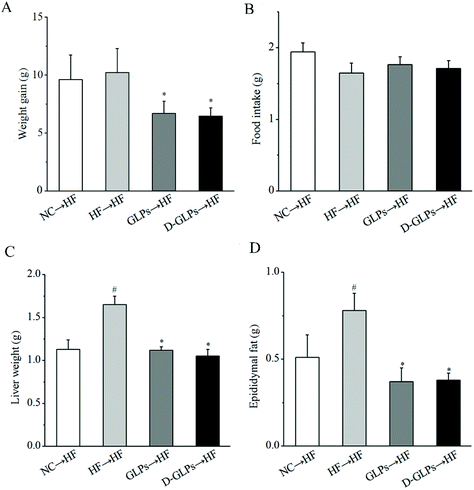 |
| Fig. 9 Effect of fecal transfer of D-GLPs-fed mice on weight gain (A), food intake (B), weights of liver (C) and epididymal fat tissue (D) in HFD-fed mice. #p < 0.05 vs. the NC group and *p < 0.05 vs. the HF group. | |
To determine the effect of the gut microbiota of D-GLPs-fed mice on the lipid profile, the levels of TC, TG, HDL-c and LDL-c in serum and liver were measured (Table 2). Fecal transfer from both GLPs and D-GLPs-fed mice had no effect on serum TC, TG and LDL-c in HFD-fed mice compared to fecal transfer from HFD-fed mice, but fecal transfer from D-GLPs-fed mice increased HDL-c in HFD-fed mice compared with fecal transfer from HFD-fed mice. Fecal transfer from GLPs- and D-GLPs-fed mice reduced the levels of TC and TG in the liver of HFD-fed mice compared to the HF → HF group, and the latter exerted a better inhibition effect on TC and TG, suggesting that D-GLPs-induced gut microbiota alteration better regulated lipid metabolism. On the other hand, SCFAs were also increased in HFD-fed mice after fecal transfer from GLPs- and D-GLPs-fed mice (Fig. 8B), indicating that the altered gut microbiota affected intestinal metabolism under the same diet conditions.52 This supports the concept that targeting dysbiotic gut microbiota can be an effective strategy to obesity and MS therapy.
Table 2 Effect of fecal transplant from D-GLPs-fed mice on lipid metabolism
|
NC → HF |
HF → HF |
GLPs → HF |
D-GLPs → HF |
The data within the same row followed by different superscript letters differ significantly (p < 0.05). |
Serum
|
TC (mmol L−1) |
5.78 ± 0.18ab |
6.03 ± 0.10a |
5.63 ± 0.11b |
5.79 ± 0.26ab |
TG (mmol L−1) |
1.04 ± 0.18a |
1.17 ± 0.09a |
1.07 ± 0.14a |
1.12 ± 0.06a |
LDL-c (mmol L−1) |
0.40 ± 0.02a |
0.37 ± 0.07a |
0.36 ± 0.02a |
0.33 ± 0.06a |
HDL-c (mmol L−1) |
2.61 ± 0.30a |
1.63 ± 0.46b |
2.16 ± 0.40ab |
2.60 ± 0.28a |
|
Liver
|
TC (μmol gprot−1) |
503.53 ± 22.17a |
510.49 ± 17.89a |
469.62 ± 25.61b |
453.88 ± 20.15b |
TG (μmol gprot−1) |
238.26 ± 16.45b |
477.81 ± 33.82a |
371.64 ± 36.28ab |
224.17 ± 7.19b |
4. Conclusion
It is indicated that D-GLPs can be used as a potential prebiotic agent to reduce HFD-induced MS and inflammation accompanied by an improvement of the gut microbiota. D-GLPs reversed HFD-induced gut microbiota dysbiosis characterized by increased abundances of some Bacteroidales members that was positively correlated with decreased lipid abnormality, fat accumulation and inflammation. Compared with GLPs, D-GLPs had similar effects on the overall structure of the gut microbiota, but it better promoted some Bacteroidales member proliferation. It was different from the effect of AGOs on the gut microbiota, indicating that the polymer length affects the prioritization strategies to polysaccharides by gut microbes. It provides a theoretical basis for precise regulation of the gut microbiota and oligosaccharide preparation.
Conflicts of interest
There are no conflicts to declare.
Acknowledgements
This was supported by the National Key R&D Program of China (2018YFD0901106) and the National Natural Science Foundation of China (No. 31701601).
References
- S. M. Grundy, Metabolic syndrome: a multiplex cardiovascular risk factor, J. Clin. Endocrinol. Metab., 2007, 92, 399–404 CrossRef CAS.
- S. M. Grundy, Metabolic syndrome pandemic, Arterioscler. Thromb. Vasc. Biol., 2008, 28, 629–636 CrossRef CAS.
- C. M. Elks and J. Francis, Central adiposity, systemic inflammation, and the metabolic syndrome, Curr. Hypertens. Rep., 2010, 12, 99–104 CrossRef CAS.
- T. S. Han and M. E. Lean, A clinical perspective of obesity, metabolic syndrome and cardiovascular disease, JRSM Cardiovasc. Dis., 2016, 5, 1–13 Search PubMed.
- P. Perez-Martinez, D. P. Mikhailidis, V. G. Athyros, M. Bullo, P. Couture, M. I. Covas, L. de Koning, J. Delgado-Lista, A. Diaz-Lopez, C. A. Drevon, R. Estruch, K. Esposito, M. Fito, M. Garaulet, D. Giugliano, A. Garcia-Rios, N. Katsiki, G. Kolovou, B. Lamarche, M. I. Maiorino, G. Mena-Sanchez, A. Munoz-Garach, D. Nikolic, J. M. Ordovas, F. Perez-Jimenez, M. Rizzo, J. Salas-Salvado, H. Schroder, F. J. Tinahones, R. de la Torre, B. van Ommen, S. Wopereis, E. Ros and J. Lopez-Miranda, Lifestyle recommendations for the prevention and management of metabolic syndrome: an international panel recommendation, Nutr. Rev., 2017, 75, 307–326 CrossRef.
- P. J. Turnbaugh, R. E. Ley, M. A. Mahowald, V. Magrini, E. R. Mardis and J. I. Gordon, An obesity-associated gut microbiome with increased capacity for energy harvest, Nature, 2006, 444, 1027–1031 CrossRef PubMed.
- R. E. Ley, P. J. Turnbaugh, S. Klein and J. I. Gordon, Microbial ecology: human gut microbes associated with obesity, Nature, 2006, 444, 1022–1023 CrossRef CAS.
- J. Qin, Y. Li, Z. Cai, S. Li, J. Zhu, F. Zhang, S. Liang, W. Zhang, Y. Guan, D. Shen, Y. Peng, D. Zhang, Z. Jie, W. Wu, Y. Qin, W. Xue, J. Li, L. Han, D. Lu, P. Wu, Y. Dai, X. Sun, Z. Li, A. Tang, S. Zhong, X. Li, W. Chen, R. Xu, M. Wang, Q. Feng, M. Gong, J. Yu, Y. Zhang, M. Zhang, T. Hansen, G. Sanchez, J. Raes, G. Falony, S. Okuda, M. Almeida, E. LeChatelier, P. Renault, N. Pons, J. M. Batto, Z. Zhang, H. Chen, R. Yang, W. Zheng, S. Li, H. Yang, J. Wang, S. D. Ehrlich, R. Nielsen, O. Pedersen, K. Kristiansen and J. Wang, A metagenome-wide association study of gut microbiota in type 2 diabetes, Nature, 2012, 490, 55–60 CrossRef CAS.
- A. Vrieze, E. Van Nood, F. Holleman, J. Salojarvi, R. S. Kootte, J. F. Bartelsman, G. M. Dallinga-Thie, M. T. Ackermans, M. J. Serlie, R. Oozeer, M. Derrien, A. Druesne, J. E. Van Hylckama Vlieg, V. W. Bloks, A. K. Groen, H. G. Heilig, E. G. Zoetendal, E. S. Stroes, W. M. de Vos, J. B. Hoekstra and M. Nieuwdorp, Transfer of intestinal microbiota from lean donors increases insulin sensitivity in individuals with metabolic syndrome, Gastroenterology, 2012, 143, 913–916 CrossRef CAS.
- X. Zhang, Y. Zhao, M. Zhang, X. Pang, J. Xu, C. Kang, M. Li, C. Zhang, Z. Zhang, Y. Zhang, X. Li, G. Ning and L. Zhao, Structural changes of gut microbiota during berberine-mediated prevention of obesity and insulin resistance in high-fat diet-fed rats, PLoS One, 2012, 7, e42529 CrossRef CAS.
- J. Henao-Mejia, E. Elinav, C. Jin, L. Hao, W. Z. Mehal, T. Strowig, C. A. Thaiss, A. L. Kau, S. C. Eisenbarth, M. J. Jurczak, J. P. Camporez, G. I. Shulman, J. I. Gordon, H. M. Hoffman and R. A. Flavell, Inflammasome-mediated dysbiosis regulates progression of NAFLD and obesity, Nature, 2012, 482, 179–185 CrossRef CAS.
- K. M. Keeney, S. Yurist-Doutsch, M. C. Arrieta and B. B. Finlay, Effects of antibiotics on human microbiota and subsequent disease, Annu. Rev. Microbiol., 2014, 68, 217–235 CrossRef CAS.
- A. Papathanasopoulos and M. Camilleri, Dietary fiber supplements: effects in obesity and metabolic syndrome and relationship to gastrointestinal functions, Gastroenterology, 2010, 138, 65–72 CrossRef CAS.
- C. J. Chang, C. S. Lin, C. C. Lu, J. Martel, Y. F. Ko, D. M. Ojcius, S. F. Tseng, T. R. Wu, Y. Y. Chen, J. D. Young and H. C. Lai, Ganoderma lucidum reduces obesity in mice by modulating the composition of the gut microbiota, Nat. Commun., 2015, 6, 7489 CrossRef CAS.
- X. Xu, P. Xu, C. Ma, J. Tang and X. Zhang, Gut microbiota, host health, and polysaccharides, Biotechnol. Adv., 2013, 31, 318–337 CrossRef CAS.
- L. Wen, Y. Zhang, D. Sunwaterhouse, L. You and X. Fu, Advantages of the polysaccharides from Gracilaria lemaneiformis over metformin in antidiabetic effects on streptozotocin-induced diabetic mice, RSC Adv., 2017, 7, 9141–9151 RSC.
- X. Liao, L. Yang, M. Chen, J. Yu, S. Zhang and Y. Ju, The hypoglycemic effect of a polysaccharide (GLP) from Gracilaria lemaneiformis and its degradation products in diabetic mice, Food Funct., 2015, 6, 2542–2549 RSC.
- X. Sun, M. Duan, Y. Liu, T. Luo, N. Ma, S. Song and C. Ai, The beneficial effects of Gracilaria lemaneiformis polysaccharides on obesity and the gut microbiota in high fat diet-fed mice, J. Funct. Foods, 2018, 46, 48–56 CrossRef CAS.
- J. Zheng, G. Cheng, Q. Li, S. Jiao, C. Feng, X. Zhao, H. Yin, Y. Du and H. Liu, Chitin Oligosaccharide Modulates Gut Microbiota and Attenuates High-Fat-Diet-Induced Metabolic Syndrome in Mice, Mar. Drugs, 2018, 16, 66 CrossRef.
- B. Scavuzzi, F. Henrique, L. Miglioranza, A. Simão and I. Dichi, Impact of prebiotics, probiotics and synbiotics on components of the metabolic syndrome, Ann. Nutr. Disord. Ther., 2014, 1, 1009 Search PubMed.
- Y. Higashimura, Y. Naito, T. Takagi, K. Uchiyama, K. Mizushima, C. Ushiroda, H. Ohnogi, Y. Kudo, M. Yasui, S. Inui, T. Hisada, A. Honda, Y. Matsuzaki and T. Yoshikawa, Protective effect of agaro-oligosaccharides on gut dysbiosis and colon tumorigenesis in high-fat diet-fed mice, Am. J. Physiol.: Gastrointest. Liver Physiol., 2016, 310, G367–G375 CrossRef PubMed.
- Y. E. Tuncil, Y. Xiao, N. T. Porter, B. L. Reuhs, E. C. Martens and B. R. Hamaker, Reciprocal Prioritization to Dietary Glycans by Gut Bacteria in a Competitive Environment Promotes Stable Coexistence, mBio, 2017, 8, e01068–e01017 CrossRef CAS.
- E. Vilkas and F. Radjabi-Nassab, The glucomannan system from Aloe vahombe (liliaceae). III. Comparative studies on the glucomannan components isolated from the leaves, Biochimie, 1986, 68, 1123–1127 CrossRef CAS.
- X.-Q. Xu, B.-M. Su, J.-S. Xie, R.-K. Li, J. Yang, J. Lin and X.-Y. Ye, Preparation of bioactive neoagaroligosaccharides through hydrolysis of Gracilaria lemaneiformis agar: A comparative study, Food Chem., 2018, 240, 330–337 CrossRef CAS.
- T. J. Borody, S. Paramsothy and G. Agrawal, Fecal microbiota transplantation: indications, methods, evidence, and future directions, Curr. Gastroenterol. Rep., 2013, 15, 337 CrossRef.
- S. Rakoff-Nahoum, M. J. Coyne and L. E. Comstock, An ecological network of polysaccharide utilization among human intestinal symbionts, Curr. Biol., 2014, 24, 40–49 CrossRef CAS.
- D. L. Topping, J. M. Gooden, I. L. Brown, D. A. Biebrick, L. McGrath, R. P. Trimble, M. Choct and R. J. Illman, A high amylose (amylomaize) starch raises proximal large bowel starch and increases colon length in pigs, J. Nutr., 1997, 127, 615–622 CrossRef CAS.
- M. Gulhane, L. Murray, R. Lourie, H. Tong, Y. H. Sheng, R. Wang, A. Kang, V. Schreiber, K. Y. Wong, G. Magor, S. Denman, J. Begun, T. H. Florin, A. Perkins, P. O. Cuiv, M. A. McGuckin and S. Z. Hasnain, High Fat Diets Induce Colonic Epithelial Cell Stress and Inflammation that is Reversed by IL-22, Sci. Rep., 2016, 6, 28990 CrossRef CAS.
- J. M. Wong, R. de Souza, C. W. Kendall, A. Emam and D. J. Jenkins, Colonic health: fermentation and short chain fatty acids, J. Clin. Gastroenterol., 2006, 40, 235–243 CrossRef CAS.
- E. Lim, J. Y. Lim, J.-H. Shin, P. R. Seok, S. Jung, S.-H. Yoo and Y. Kim, D-Xylose suppresses adipogenesis and regulates lipid metabolism genes in high-fat diet-induced obese mice, Nutr. Res., 2015, 35, 626–636 CrossRef CAS.
- S. J. Hong, J. H. Lee, E. J. Kim, H. J. Yang, J. S. Park and S. K. Hong, Anti-Obesity and Anti-Diabetic Effect of Neoagarooligosaccharides on High-Fat Diet-Induced Obesity in Mice, Mar. Drugs, 2017, 15, 90 CrossRef.
- S. Li, J. Li, G. Mao, Y. Hu, X. Ye, D. Tian, R. J. Linhardt and S. Chen, Fucosylated chondroitin sulfate oligosaccharides from Isostichopus badionotus regulates lipid disorder in C57BL/6 mice fed a high-fat diet, Carbohydr. Polym., 2018, 201, 634–642 CrossRef CAS PubMed.
- J. S. Lee, P. Y. Chang, Y. Zhang, J. R. Kizer, L. G. Best and B. V. Howard, Triglyceride and HDL-C Dyslipidemia and Risks of Coronary Heart Disease and Ischemic Stroke by Glycemic Dysregulation Status: The Strong Heart Study, Diabetes Care, 2017, 40, 529–537 CrossRef.
- R. M. Krauss, Lipoprotein subfractions and cardiovascular disease risk, Curr. Opin. Lipidol., 2010, 21, 305–311 CrossRef CAS.
- V. Greenfield, O. Cheung and A. J. Sanyal, Recent advances in nonalcholic fatty liver disease, Curr. Opin. Gastroenterol., 2008, 24, 320–327 CrossRef.
- Y. Z. Fang, S. Yang and G. Wu, Free radicals, antioxidants, and nutrition, Nutrition, 2002, 18, 872–879 CrossRef CAS.
- M. Ma, G. Liu, Z. Yu, G. Chen and X. Zhang, Effect of the Lycium barbarum polysaccharides administration on blood lipid metabolism and oxidative stress of mice fed high-fat diet in vivo, Food Chem., 2009, 113, 872–877 CrossRef CAS.
- N. Wu, L. K. Sarna, S. Y. Hwang, Q. Zhu, P. Wang, Y. L. Siow and K. O, Activation of 3-hydroxy-3-methylglutaryl coenzyme A (HMG-CoA) reductase during high fat diet feeding, BBA, Mol. Basis Dis., 2013, 1832, 1560–1568 CrossRef CAS.
- Y. D. Wang, W. D. Chen, D. D. Moore and W. Huang, FXR: a metabolic regulator and cell protector, Cell Res., 2008, 18, 1087–1095 CrossRef CAS.
- Z. Chen, D. Chu, J. M. Castro-Perez, W. Ni, A. Zhang, M. L. Krsmanovic, D. Xie, V. Shah, S. J. Stout, D. G. McLaren, A. C. Stefanni, S. H. Lee, T. P. Roddy, A. S. Plump, B. K. Hubbard, T. F. Vogt and H. H. Zhou, AAV8-mediated long-term expression of human LCAT significantly improves lipid profiles in hCETP;Ldlr(+/-) mice, J. Cardiovasc. Transl., 2011, 4, 801–810 CrossRef.
- Y. Cao, Y. Sun, S. Zou, M. Li and X. Xu, Orally Administered Baker's Yeast beta-Glucan Promotes Glucose and Lipid Homeostasis in the Livers of Obesity and Diabetes Model Mice, J. Agric. Food Chem., 2017, 65, 9665–9674 CrossRef CAS.
- A. Chawla, K. D. Nguyen and Y. P. Goh, Macrophage-mediated inflammation in metabolic disease, Nat. Rev. Immunol., 2011, 11, 738–749 CrossRef CAS.
- A. Jaja-Chimedza, L. Zhang, K. Wolff, B. L. Graf, P. Kuhn, K. Moskal, R. Carmouche, S. Newman, J. M. Salbaum and I. Raskin, A dietary isothiocyanate-enriched moringa (Moringa oleifera) seed extract improves glucose tolerance in a high-fat-diet mouse model and modulates the gut microbiome, J. Funct. Foods, 2018, 47, 376–385 CrossRef CAS.
- M. Feuerer, L. Herrero, D. Cipolletta, A. Naaz, J. Wong, A. Nayer, J. Lee, A. B. Goldfine, C. Benoist, S. Shoelson and D. Mathis, Lean, but not obese, fat is enriched for a unique population of regulatory T cells that affect metabolic parameters, Nat. Med., 2009, 15, 930–939 CrossRef CAS PubMed.
- M. Million, J. C. Lagier, D. Yahav and M. Paul, Gut bacterial microbiota and obesity, Clin. Microbiol. Infect., 2013, 19, 305–313 CrossRef CAS.
- C. De Filippo, D. Cavalieri, M. Di Paola, M. Ramazzotti, J. B. Poullet, S. Massart, S. Collini, G. Pieraccini and P. Lionetti, Impact of diet in shaping gut microbiota revealed by a comparative study in children from Europe and rural Africa, Proc. Natl. Acad. Sci. U. S. A., 2010, 107, 14691–14696 CrossRef.
- M. K. Bjursell, E. C. Martens and J. I. Gordon, Functional genomic and metabolic studies of the adaptations of a prominent adult human gut symbiont, Bacteroides thetaiotaomicron, to the suckling period, J. Biol. Chem., 2006, 281, 36269–36279 CrossRef CAS PubMed.
- X. Song, L. Zhong, N. Lyu, F. Liu, B. Li, Y. Hao, Y. Xue, J. Li, Y. Feng and Y. Ma, Inulin can alleviate metabolism disorders in ob/ob mice by partially restoring leptin-related pathways mediated by gut microbiota, Genomics, Proteomics Bioinf., 2019, 17, 64–75 CrossRef PubMed.
- T. J. Khan, Y. M. Ahmed, M. A. Zamzami, S. A. Mohamed, I. Khan, O. A. S. Baothman, M. G. Mehanna and M. Yasir, Effect of atorvastatin on the gut microbiota of high fat diet-induced hypercholesterolemic rats, Sci. Rep., 2018, 8, 662 CrossRef PubMed.
- R. Liu, J. Hong, X. Xu, Q. Feng, D. Zhang, Y. Gu, J. Shi, S. Zhao, W. Liu, X. Wang, H. Xia, Z. Liu, B. Cui, P. Liang, L. Xi, J. Jin, X. Ying, X. Wang, X. Zhao, W. Li, H. Jia, Z. Lan, F. Li, R. Wang, Y. Sun, M. Yang, Y. Shen, Z. Jie, J. Li, X. Chen, H. Zhong, H. Xie, Y. Zhang, W. Gu, X. Deng, B. Shen, X. Xu, H. Yang, G. Xu, Y. Bi, S. Lai, J. Wang, L. Qi, L. Madsen, J. Wang, G. Ning, K. Kristiansen and W. Wang, Gut microbiome and serum metabolome alterations in obesity and after weight-loss intervention, Nat. Med., 2017, 23, 859–868 CrossRef CAS.
- H. Lin, Y. An, F. Hao, Y. Wang and H. Tang, Correlations of Fecal Metabonomic and Microbiomic Changes Induced by High-fat Diet in the Pre-Obesity State, Sci. Rep., 2016, 6, 21618 CrossRef CAS.
- G. den Besten, K. van Eunen, A. K. Groen, K. Venema, D.-J. Reijngoud and B. M. Bakker, The role of short-chain fatty acids in the interplay between diet, gut microbiota, and host energy metabolism, J. Lipid Res., 2013, 54, 2325–2340 CrossRef CAS PubMed.
- G. Jakobsdottir, J. Xu, G. Molin, S. Ahrne and M. Nyman, High-Fat Diet Reduces the Formation of Butyrate, but Increases Succinate, Inflammation, Liver Fat and Cholesterol in Rats, while Dietary Fibre Counteracts These Effects, PLoS One, 2013, 8, e80476 CrossRef PubMed.
- J. Slavin, Fiber and prebiotics: mechanisms and health benefits, Nutrients, 2013, 5, 1417–1435 CrossRef CAS PubMed.
- D. L. Topping and P. M. Clifton, Short-chain fatty acids and human colonic function: roles of resistant starch and nonstarch polysaccharides, Physiol. Rev., 2001, 81, 1031–1064 CrossRef CAS.
- P. Louis and H. J. Flint, Formation of propionate and butyrate by the human colonic microbiota, Environ. Microbiol., 2017, 19, 29–41 CrossRef CAS.
- Y. Lu, C. Fan, P. Li, Y. Lu, X. Chang and K. Qi, Short Chain Fatty Acids Prevent High-fat-diet-induced Obesity in Mice by Regulating G Protein-coupled Receptors and Gut Microbiota, Sci. Rep., 2016, 6, 37589 CrossRef CAS.
- V. K. Ridaura, J. J. Faith, F. E. Rey, J. Cheng, A. E. Duncan, A. L. Kau, N. W. Griffin, V. Lombard, B. Henrissat, J. R. Bain, M. J. Muehlbauer, O. Ilkayeva, C. F. Semenkovich, K. Funai, D. K. Hayashi, B. J. Lyle, M. C. Martini, L. K. Ursell, J. C. Clemente, W. Van Treuren, W. A. Walters, R. Knight, C. B. Newgard, A. C. Heath and J. I. Gordon, Gut microbiota from twins discordant for obesity modulate metabolism in mice, Science, 2013, 341, 1241214 CrossRef.
Footnote |
† Electronic supplementary information (ESI) available: Fig. S1 and Table S1. See DOI: 10.1039/c9fo01996k |
|
This journal is © The Royal Society of Chemistry 2020 |
Click here to see how this site uses Cookies. View our privacy policy here.