DOI:
10.1039/C9FO02719J
(Paper)
Food Funct., 2020,
11, 846-859
Protective effect of Lachnum polysaccharide on dextran sulfate sodium-induced colitis in mice†
Received
19th November 2019
, Accepted 30th December 2019
First published on 10th January 2020
Abstract
Inflammatory bowel disease (IBD) has been gradually considered as a public health challenge worldwide. This study determined the protective effect of Lachnum polysaccharide (LEP) on dextran sulfate sodium (DSS)-induced experimental colitis in mice and explored the underlying mechanism. Results showed that dietary LEP reduced DSS-induced disease activity index (DAI), colon shortening and colonic tissue damage. LEP treatment restored intestinal barrier integrity by regulating the expression of tight junction proteins and mucus layer protecting proteins. Moreover, pro-inflammatory cytokine production was inhibited by LEP through regulating PPARγ/NF-κB and IL-6/STAT3 pathways and inhibiting inflammatory cell infiltration. In addition, LEP also inhibited (NOD)-like receptor family pyrin domain containing 3 (NLRP3) inflammasome activation, endoplasmic reticulum (ER) stress and oxidative/nitrosative stress induced by DSS. These results provided a scientific basis for LEP as a potential natural agent for protecting mice from DSS-induced IBD.
1. Introduction
Inflammatory bowel diseases (IBD), including ulcerative colitis (UC) and Crohn's disease (CD), are chronic relapsing inflammatory conditions associated with an increased risk of colorectal carcinoma. Increasing evidence revealed that IBD has become a global disease with accelerating incidence, the scope of which spreads across not only the Western world, but also many countries in South America, Asia and Africa. Even though the precise pathogenesis of IBD is still unknown, it is generally recognized that a variety of factors are related to the formation of the disease, including genetics and environmental factors.1 As a consequence, it is hard to find an effective way to prevent and alleviate this inflammatory disease. Current strategies for treating of IBD in the clinical aspect are mainly drug intervention, including corticosteroids (such as prednisone and prednisolone), immunosuppressive agents (such as azathioprine, 6-mercaptopurine and thioguanine), biological agents (such as infliximab) and antibiotics (such as metronidazole and methylprednisolone), and eventually surgical treatment.2 However, significant side effects or exorbitant price severely limited the application of these drugs, indicating that current therapeutic strategies are unsatisfactory. As a consequence, exploiting novel therapeutic agents or therapeutic options with high efficiency and low side effects are urgently needed.
In recent years, the application of certain foods or dietary compositions with strong biological activity and negligible side effects has been highly advocated, which provides an effective way to prevent and treat diseases, including IBD. Many research studies have been carried out to explore the feasibility of edible phytochemicals, such as peptides, amino acids, fatty alcohols, polyphenols and polysaccharides, as alternative therapeutic schedules for IBD. For example, Kobayashi et al. demonstrated that the anti-inflammatory effect of Trp-His (an anti-atherosclerotic dipeptide) was involved in the blockade of L-type Ca2+ channels in IBD.3 Dietary arginine was proved to decrease colonic impairment, inflammation and oxidative stress of DSS-induced colitis in mice.4 Guo et al. demonstrated that octacosanol, a kind of high-level saturated fatty alcohol, significantly improved the health status of pathological damage and alleviated inflammation in the colonic tissues of IBD mice.5 Resveratrol, a polyphenol, was proved to prevent barrier defects and inflammation in dextran sulfate sodium (DSS)-induced colitis mice by inhibiting inflammatory signaling and reducing neutrophil infiltration.6 In addition, as a large class of natural biopolymers, protective effect of polysaccharides against DSS-induced bowel disease was also documented. Jin et al. showed that a polysaccharide extracted from noni (Morinda citrifolia L.) promoted the expression of intestinal tight junction proteins and the secretion of mucus to improve the clinical diagnosis of acute colitis in IBD mice.7 Moreover, polysaccharide isolated from Phellinus linteus mycelia exerted anti-inflammatory function via obstructing MAPK and PPAR signaling pathways.8
Inflammation is one of the dominating clinical symptoms of IBD. In the presence of persistent inflammation, severe tissue inflammation was developed, accompanied with pro-inflammatory cytokines (IL-1β, TNF-α and IL-6) secretion, which could increase intestinal permeability and hamper mucosal barrier function.9 Nuclear factor-κB (NF-κB) cascades are considered as one the major pathways that promote inflammatory cytokines production. Growing evidence has suggested that NF-κB plays a key role in the pathogenesis of IBD, and targeted inhibition of NF-κB showed relieved colitis severity.10 Moreover, over-produced pro-inflammatory cytokines could in turn stimulate reactive oxygen species (ROS) production, which ultimately resulted in intestinal epithelial cell injury and contributed to the aggravated pathogenesis.11 The intestinal epithelial barrier is considered as the first line of defense against luminal pro-inflammatory substances such as bacterial endotoxins.6 Patients with IBDs exhibit dysfunctional intestinal barrier. Once the epithelial barrier integrity was impaired by DSS, activated immune cells would accumulated in inflamed tissues in response to pro-inflammatory substances, which could lead to the robust and chronic activation of immune cells.12 Maintaining and protecting intestinal barrier integrity and function could be an effective approach against intestinal inflammation.13
Lachnum sp. is a genus of higher fungi with extremely high bioactivity value, which grows throughout Asia, America, northern and Europe. During the past decades, it was found that under submerged fermentation condition, Lachnum sp. can produce various active metabolites such as polysaccharides, pigments and polyphenols.14 It has been demonstrated that Lachnum polysaccharide exerted anti-inflammatory and antioxidant effects to prevent liver damage by inhibiting NF-κB and enhancing antioxidant defense system.15 Peroxisome proliferator-activated receptors (PPARs) are crucial anti-inflammatory molecules in chronic inflammatory diseases, which are able to abrogate the NF-κB signaling pathway and restrain pro-inflammatory cytokines production.16 Furthermore, promoting PPAR-γ activation was evidenced to protect mice from DSS-induced IBD.17 Our previous studies have shown that polysaccharide from Lachnum sp. significantly up-regulated PPAR-γ in adipose tissue of type 2 diabetic mice.18 Collectively, these biological actions imply that Lachnum polysaccharide may play a role to ameliorate IBD, however relevant research has never been carried out.
The present study for the first time investigated the ameliorative effect of dietary Lachnum polysaccharide in a murine model of colitis induced by DSS, and explored the potential mechanism focusing on restoring intestinal barrier integrity, regulating intestinal immune and inflammatory response, and reducing oxido-nitrosative stress.
2. Materials and methods
2.1. Materials and reagents
Lachnum YM130 polysaccharide (LEP), with the molecular weight of 1.31 × 106 Da, sugar composition of mannose and galactose in a molar ratio of 3.8
:
1.0, and the yield of 6.28 g L−1 in the fermentation broth, was prepared according to the previous method19,20 and preserved in a sealed bag under −20 °C in Microbial Resources and Application Laboratory of Hefei University of Technology until use. DSS (molecular weight 36–50 kDa) was purchased from MP Biomedicals (Santa Ana, CA, USA). ELISA detection kits for lipopolysaccharide binding protein (LBP), lipopolysaccharide (LPS), myeloperoxidase (MPO), prostaglandin E2 (PGE2), tumor necrosis factor (TNF)-α, interleukin (IL)-1β and interleukin (IL)-6 were provided by Shanghai Yansheng industrial Co., Ltd (Shanghai, China). Test kits for bicinchoninic acid (BCA), catalase (CAT), malonic dialdehyde (MDA), nitric oxide (NO), superoxide dismutase (SOD) and total antioxidant capacity (T-AOC) were purchased from Nanjing Jiancheng Bioengineering Institute (Nanjing, China). The primary antibodies used in this study are listed in ESI Table S1.† All other chemicals were of reagent grade.
2.2. Ethics statement
Male ICR mice (6–8 weeks) were obtained from the Laboratory Animal Center of Anhui Medical University (certificate number: no. 1 license of the Medical Laboratory Animal of Anhui), Hefei, China. The mice were housed under an air-conditioned atmosphere with a 12 h light–dark cycle, controlled temperature of 24 ± 1 °C, and were provided water and food ad libitum. They were acclimatized for one week before being used in the experiment. All experimental protocols in this study were guided in accordance with the National Institutes of Health guide for the care and use of Laboratory animals (NIH publications no. 8023, revised 1978), and approved by the Institutional Animal Care and Use Committee of Hefei University of Technology, China.
2.3. Animals experimental design
Mice were randomly assigned into three groups (eight mice in each group), including control group (CON), DSS group (DSS) and DSS + LEP group (LEP). The experimental timelines of the animal model and treatment are described in Fig. 1A. Before DSS treatment, mice in LEP group were administered with LEP (200 mg kg−1) by gavage once per day for two weeks, and the same amount of saline was used to feed other mice. On day 15, mice in DSS and LEP groups were fed with DSS (2.5%) water for 1 week. On day 22, DSS was removed and mice were kept for another week. LEP and saline were administered as usual along with and after DSS treatment. During the whole experiment, body weights of the mice and disease severity were monitored daily using an ulcerative colitis disease activity index (DAI) as described previously.21 On day 29, mice were sacrificed, and the serum and colons were collected. The length of colon was measured from the ileocecal junction to the anal verge. Half of the colon specimens were fixed in 10% formalin and another half were snap frozen in liquid nitrogen and stored at −80 °C.
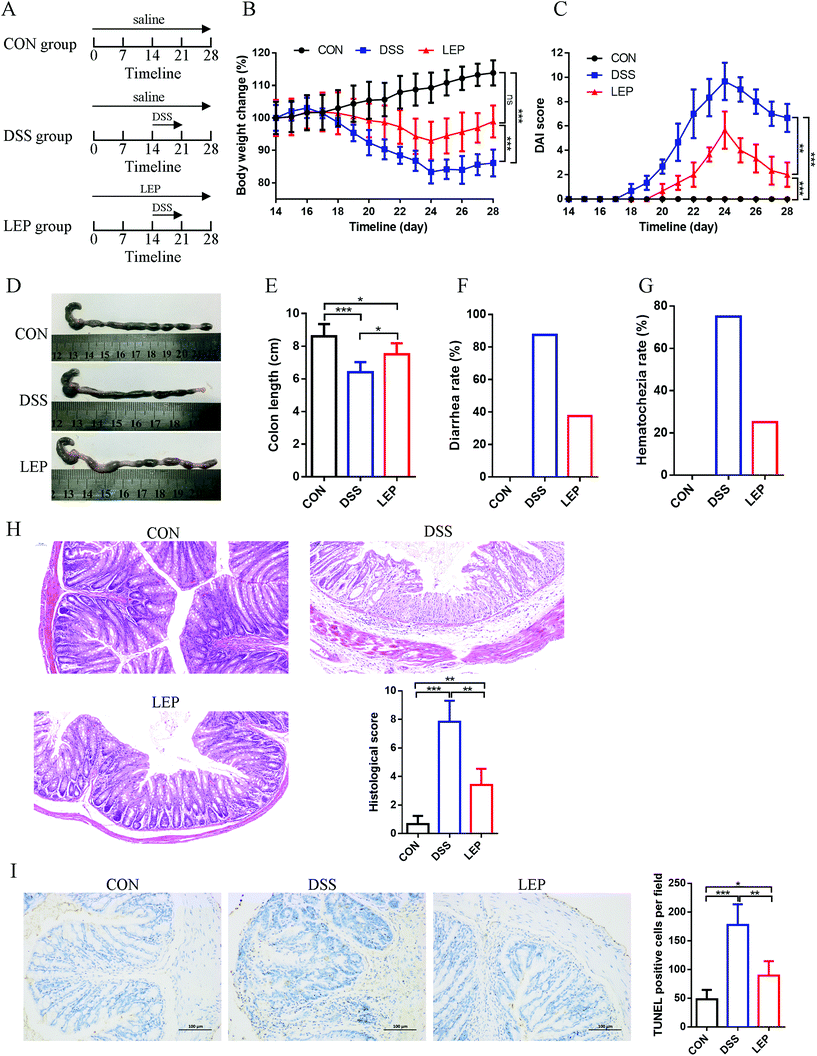 |
| Fig. 1 LEP ameliorated symptoms in DSS-induced colitic mouse model. (A) Experimental timelines of the animal model and treatment. (B) Percentage of initial body weight. (C) DAI score. (D) Representative photos of colon length. (E) Colon length, (F) diarrhea rate and (G) hematochezia rate were measured. (H) Colonic histologic and pathological scores. (I) Representative TUNEL staining and positive cells of colonic sections. Data are expressed as mean ± SD (n = 3). Ns, no significant. *p < 0.05, **p < 0.01 and ***p < 0.001. | |
2.4. Measurement of oxidative stress parameters and cytokines
Levels of LBP and LPS in serum, IL-1β, TNF-α and IL-6 in both serum and colonic tissues, and MPO activity and PGE2 content in colonic tissues were analyzed by ELISA kits according to the manufacturer's protocols. CAT, MDA, NO, SOD, T-AOC from colonic tissues were assessed using commercial kits following the manufacturer's instructions.
2.5. Measurement of serum nitrates and nitrites (NOx)
Serum NOx was estimated by the Griess reaction.22 In brief, 50 μL serum was mixed with 10 μL NADPH, 10 μL FAD, 10 μL nitrate reductase (2 U ml−1) and 20 μL potassium phosphate buffer, and incubated at 37 °C in the dark for one hour. Then, 5 μL zinc sulphate (1 M L−1) was added. The supernatant (50 μL) of the mixture was obtained by centrifugation, and then mixed with 100 μL Griess reagent. After stabilized for 10 min at room temperature, absorbance was measured at 540 nm.
2.6. Western blot analysis
Relative protein levels in colonic tissues were analyzed by western blotting as previously described.20 The primary antibodies used in the present study are listed in Table S1 in ESI.† The band density was normalized to β-actin when analyzed.
2.7. Histopathological examination
After fixed in 10% formalin for 24 h, colonic tissues were embedded in paraffin, and cut into slices (5 μm). The slices were stained with hematoxylin–eosin (H&E) and alcian-blue/periodic acid Schiff reagent (AB-PAS), and then observed using a light microscope. The histological scores were marked as described elsewhere.23
2.8. Immunohistochemical and immunofluorescence analysis
Immunohistochemistry was performed according to the manufacturer's instructions (LSAB kit; Dako, Carpinteria, CA, USA). Antigen retrieval was performed by boiling the slide in 10 mM sodium citrate (pH 6.0) for 30 min. Then, slides were incubated overnight at 4 °C with primary antibodies (Table S1†). Afterwards, the slides were incubated with horseradish peroxidase (HRP)-conjugated secondary antibodies, developed in 3,3′-diaminobenzidine (DAB), counterstained with hematoxylin, and mounted in resin. Five-grade system was applied to determine the score of nitrotyrosine staining.24
For immunofluorescence assessment, slides were incubated with primary antibodies (Table S1†) at 4 °C overnight and incubated with FITC-labeled secondary antibody for 1 h at room temperature. Mounting medium containing 4,6-diamidino-2-phenylindole (DAPI) was used for nuclear counterstaining. The slices were observed using an inverted fluorescence microscope (NIKON ECLIPSE TI-SR, Japan). Positive cells were quantified using Image-Pro Plus 6.0 software (Media Cybernetics, Inc., Rockville, MD, USA).
2.9. qRT-PCR analysis
Total RNA of colonic sample was isolated using PureLink™ RNA Mini Kit (Invitrogen, CA, US). Complementary cDNA was synthesized using a Revert Aid First Strand cDNA Synthesis Kit (Thermo Scientific) and quantified with SYBR-Green assays on Roche LightCycler96 (Roche, Germany). The relative expression levels were determined via the ΔΔCt method. The mRNA expression from each sample was calculated by normalizing with β-actin as an endogenous control. The primers used in this study were provided in Table S2.†
2.10. Terminal deoxynucleotidyl transferase-mediated dUTP nick end labeling (TUNEL) assay
Apoptotic cells in the colonic tissues were detected by TUNEL staining (Millipore, USA) according to the manufacturer's instruction. The cells observed under the microscope with brown color nuclei were considered to be TUNEL-positive cells. Fields were taken at 200× magnification, and integrated optical density (IOD) values or stained cells were counted using Image-Pro Plus 6.0 software (Media Cybernetics, Inc., Rockville, MD, USA).
2.11. Statistical analysis
Data were expressed as mean ± SD. Statistical analysis was performed with SPSS 22.0 (SPSS Inc., Chicago, IL, USA). A paired-samples t-test was performed to analyze the relationships between two groups. Multiple comparisons were evaluated using one-way ANOVA followed by Tukey's multiple-comparison test. P < 0.05 was considered statistically significant.
3. Results
3.1. LEP Treatment ameliorated DSS-induced colitis
In the present study, the protective therapeutic effect of LEP on DSS-induced colitis model was investigated. Experimental timelines of the animal model and treatment were displayed in Fig. 1A. As shown in Fig. 1B and C, compared to DSS group, body weight and DAI score were considerably reduced after LEP treatment. Macroscopically, other clinical parameters such as colon length, stool consistency and blood were also measured. Shortened colon, high diarrhea and hematochezia rate were observed in DSS treatment group. However LEP improved these symptoms (Fig. 1D–G). In addition, LEP ameliorated DSS-induced histopathologic damage, including crypts lost, mucosa ulceration, inflammatory infiltration and necrosis (Fig. 1H). Moreover, as evidenced by TUNEL positive cells, DSS administration led to abundant cell apoptosis, which was attenuated by LEP (Fig. 1I). These data indicated that LEP treatment showed mitigating effect on the clinical symptoms of the damage induced by DSS.
3.2. LEP treatment restored intestinal barrier in mice with colitis
Intestinal barrier integrity plays an important role in maintaining stable internal environment in the intestine and reducing inflammation levels. As addressed above, the overall histopathological changes were improved by LEP, thus we speculated that LEP might act on tight junction (TJ) proteins and mucus layer protecting proteins to restore the barrier function and regulate intestinal permeability in colons. As shown in Fig. 2A and B, LPS and LBP contents, biomarkers of intestinal barrier impairment, in serum were significantly increased in DSS group, however LEP reversed this change. TJ protein expressions were further examined by immunoblot analyses. Compared to CON group, DSS-induced colitis resulted in a significant reduction in the expression of TJ proteins, whereas a restoration was observed in LEP group (Fig. 2C), indicating that LEP alleviated intestinal barrier injury induced by DSS. Moreover, mRNA levels of mucus layer protecting proteins, such as mucin 1 (MUC1), mucin 2 (MUC2), trefoil factor 3 (TFF3), resistin-like molecule β (Relmβ), and the quantity of goblet cells were also evaluated after LEP administration (Fig. 2D and E). Antibacterial proteins play a crucial part in keeping bacteria at a distance from the intestinal epithelium. In the present study, LEP treatment led to a sharp increase of regenerating islet-derived 3β (Reg3β) and regenerating islet-derived 3γ (Reg3γ) in colon tissue (Fig. 2D), which indicated that LEP enhanced the intestinal barrier function. The major isoforms of the claudin family, claudin-3 and claudin-7, were visualized by immunofluorescence microscopy. As shown in Fig. 2F, in CON group, claudin-3 and -7 were expressed in the junctional and basolateral regions. DSS administration seriously impaired the expression and localization of the two TJ proteins, whereas LEP supplementation clearly attenuated these impairments. Collectively, these data demonstrated that LEP restored intestinal barrier function and alleviated the impairments induced by DSS.
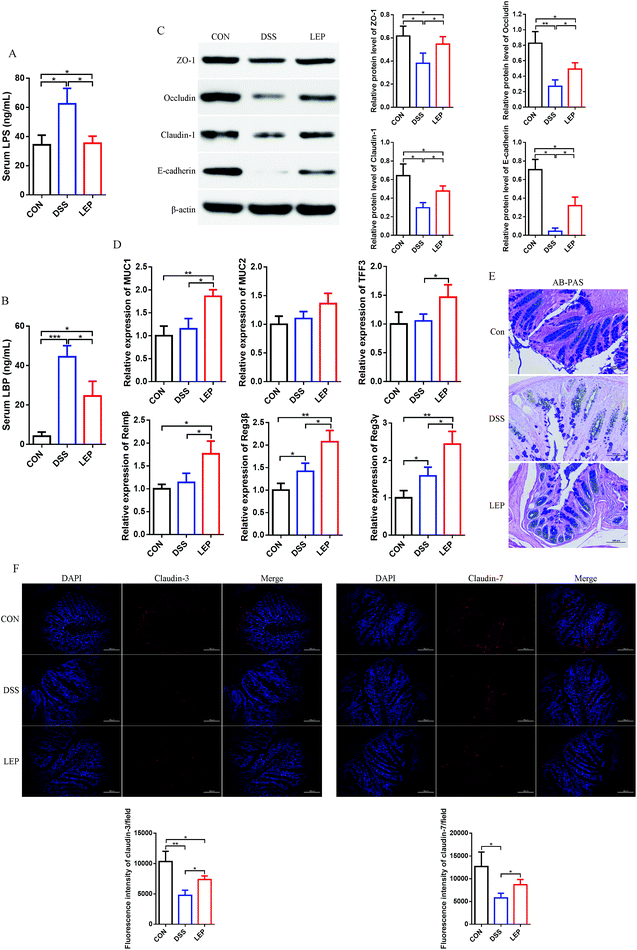 |
| Fig. 2 LEP relieved intestinal barrier defects induced by DSS. Levels of LPS (A) and LBP (B) in serum. (C) Changes of tight junction proteins (ZO-1, occluding, claudin-1 and E-cadherin) in colon tissues. (D) Relative mRNA expression of mucus layer protecting proteins (MUC1 MUC2, TFF3 and Relmβ) and antimicrobial proteins (Reg3b and Reg3g). (E) AB-PAS staining of the colon tissues. (F) Representative images of claudin-3 and claudin-7 immunostaining and DAPI in colonic sections. Data are expressed as mean ± SD (n = 3). *p < 0.05, **p < 0.01 and ***p < 0.001. | |
3.3. LEP suppressed DSS-induced inflammatory mediators production and NF-κB signaling pathway activation
Aberrant up-regulation of pro-inflammatory cytokines is a widely accepted marker of DSS-induced inflammation. Next, the effect of LEP on DSS-induced production of inflammatory cytokines was investigated. As shown in Fig. 3A–C, levels of TNF-α, IL-1β and IL-6 were significantly evaluated after DSS treatment, whereas the ascending behavior of these cytokines was mitigated by LEP supplementation. Our previous results showed that Lachunm polysaccharide un-regulated protein level of PPARγ in type 2 diabetic mice.18 As shown in Fig. 3D, the down-regulated protein level of PPARγ induced by DSS was inhibited after LEP treatment. Moreover, the protein level of key pro-inflammatory proteins, iNOS and COX-2, were obviously declined in LEP group compared to DSS group. In parallel, downstream molecules of iNOS and COX-2, NO (Fig. 3E) and PGE2 (Fig. 3F), were also decreased after LEP administration. PPARγ is one of the upstream molecules of NF-κB pathway. NF-κB activation will lead to abundant pro-inflammatory proteins expression, including iNOS and COX-2. Therefore, we speculated that NF-κB might play a key role in the anti-inflammatory action of LEP. Results showed that dietary LEP effectively suppressed the activation of NF-κB signaling induced by DSS (Fig. 3G). Together, these results suggested that LEP might exert anti-inflammatory capacity by evaluating PPARγ and suppressing NF-κB activation.
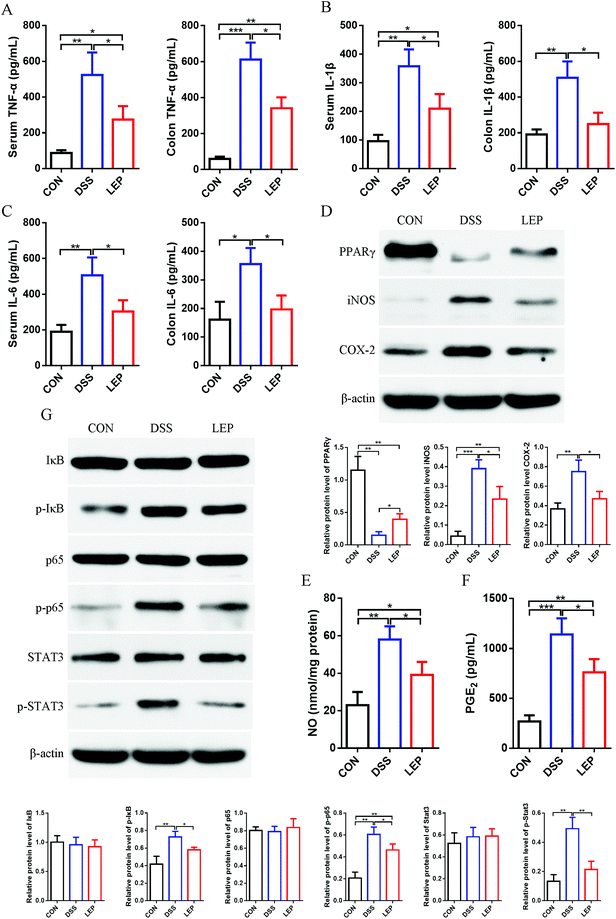 |
| Fig. 3 LEP inhibited inflammatory cytokines expression in DSS-induced colitis. Levels of TNF-α (A), IL-1β (B) and IL-6 (C) in serum and colon tissues were measured. (D) Protein levels of PPARγ, iNOS and COX-2 in colonic tissue were analyzed by western blotting. Levels of NO (E) and PGE2 (F) in colon. (G) Protein expression of key molecules in NF-κB pathway. Data are expressed as mean ± SD (n = 3). *p < 0.05, **p < 0.01 and ***p < 0.001. | |
3.4. LEP inhibited the infiltration of inflammatory cells into colonic tissue
Inflammatory cells recruitment from blood into inflamed colonic tissues is both inducement and indication of intestinal inflammation. In this study, it was found that LEP decreased pro-inflammatory cytokines in both colon and serum; thereafter, we speculated that LEP might also play a role in inflammatory cells recruitment. Compared to DSS group, MPO activity, a biomarker of neutrophil infiltration, was significantly decreased after LEP administration (Fig. 4A). Consistently, IHC staining of Ly6G, one of neutrophil biomarkers, was evidently increased after DSS treatment; however, LEP inhibited this change (Fig. 4B). In addition, another dominant pro-inflammatory immune cell, macrophages, were also reduced after LEP treatment (Fig. 4C). DSS treatment also induced inflammation through T helper (Th) cells; therefore, the effect of LEP on Th cells infiltration was examined. As shown in Fig. 4D, DSS administered mice showed a higher population of CD4+IL-10+ Th2 cells in colonic tissues, whereas the increase was remarkably diminished by LEP supplementation. These data demonstrated that LEP inhibited inflammatory cells infiltration induced by DSS.
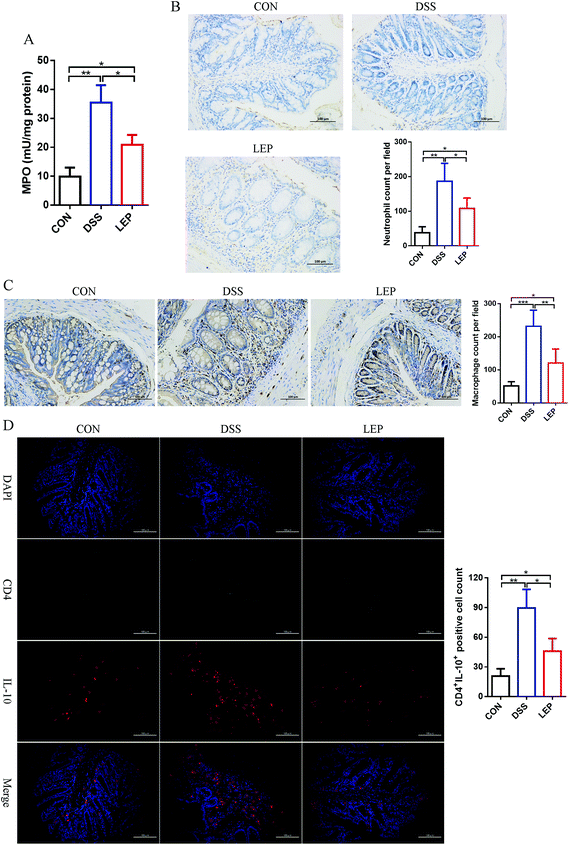 |
| Fig. 4 LEP reduced inflammatory cells infiltration in DSS-induced colitic mouse colons. (A) MPO activity in colon tissues. (B) Representative images and quantification numbers of Ly6G neutrophil marker staining. (C) Representative images and quantification numbers of F4/80 macrophage marker staining. (D) Representative images and quantification numbers of CD4+IL-4+ Th2 cells marker staining. Data are expressed as mean ± SD (n = 3). *p < 0.05, **p < 0.01 and ***p < 0.001. | |
3.5. LEP suppressed NLRP3 inflammasome activation in colonic tissue
Bioactive IL-1β is a unique cytokine, whose secretion relies on inflammasome activation.25 In this study, we have demonstrated that LEP reduced the level of IL-1β in serum and colon, which enlightened a possibility to suppressed NLRP3 inflammasome activation. Western blotting results showed that LEP treatment considerably inhibited the overexpression of NLRP3, apoptosis-associated speck-like protein containing a CARD (ASC) and caspase-1 induced by DSS, and resulted in a reduction of IL-1β secretion (Fig. 5). Together, these results showed that LEP might have anti-inflammatory capacity by inhibiting NLRP3 inflammasome activation.
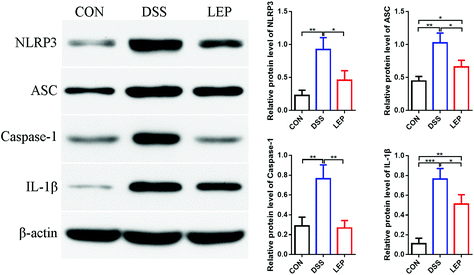 |
| Fig. 5 LEP reduced NLRP3 inflammasome activation in colonic tissues. Protein levels of IL-1β, NLRP3, ASC and caspase-1 were determined by western blotting and normalized to β-actin. Data are expressed as mean ± SD (n = 3). *p < 0.05, **p < 0.01 and ***p < 0.001. | |
3.6. LEP reduced endoplasmic reticulum (ER) stress in mice with DSS-induced colitis
ER stress is usually accompanied by the development of colitis and tumorigenesis, which ultimately leads to cell apoptosis.26 Our data in this study demonstrated that LEP reduced colitis and TUNEL positive cells in colon. Next, whether anti-inflammatory and anti-apoptosis efficacy of LEP was related to ER stress pathway has been investigated. Under ER stress, misfolded proteins interact with binding immunoglobulin protein (BiP/GRP78), resulting in its release form the stable complexes with protein kinase RNA-like ER kinase (PERK), activating transcription factor 6 (ATF6) and inositol-requiring enzyme 1 (IRE1α), unfolded protein response (UPR) is subsequently activated.27 As shown in Fig. 6, protein level of PERK was evidently decreased after LEP treatment; the change of ATF6 was not obvious compared to DSS group. However, IRE1α expression was significant up-regulated in LEP group compared to DSS group. Next, levels of XBP1 and CHOP, downstream key molecules of IRE1α and PTEN, respectively, were examined. Results showed that compared to DSS group, protein expression of CHOP declined in LEP group, whereas XBP1 showed the opposite trend, indicating that LEP might attenuate ER stress through regulating IRE1α-XBP1 and PTEN-CHOP branches of the UPR signaling pathway. Moreover, expression of cleaved-caspase 3 was reduced strongly after LEP administration, suggesting that apoptosis induced by DSS exposure was inhibited.
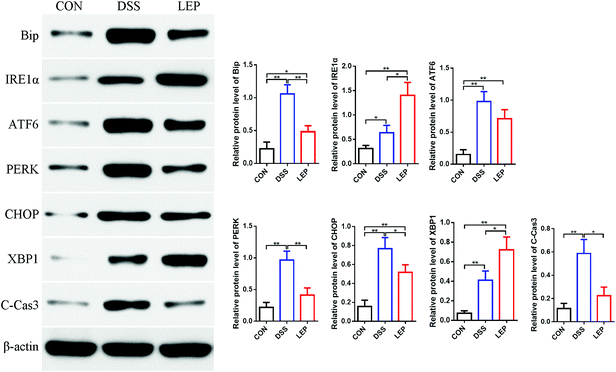 |
| Fig. 6 LEP decreased ER stress in mice with DSS-induced colitis. Data are expressed as mean ± SD (n = 3). *p < 0.05 and **p < 0.01. | |
3.7. LEP relieved intestine oxidative stress and nitrosative stress in mice with DSS-induced colitis
In addition to abnormal inflammatory response, oxidative stress as well as nitrosative stress is also considered to be a critical event during intestinal inflammatory progression. As shown in Fig. 7A, DSS treatment significantly promoted the MDA level, on the contrary, down-regulated the enzyme activity of SOD and CAT, and the ability of T-AOC. However, these obvious changes were abrogated by LEP treatment. Apart from oxidative stress, nitrogen species (RNS) also play an important role in causing tissue dysfunction. Our data in this study demonstrated that LEP inhibited excessive protein expression of iNOS and NO level, suggesting a potential of LEP to inhibit nitrosative stress. The immunoreactivity of nitrotyrosine, one of the proposed indexes of nitrosative stress, was then detected. As shown in Fig. 7B, stronger positive nitrotyrosine immunoreactivity was observed in DSS group compared to CON group; however, this change was alleviated by LEP administration. In parallel, serum NOx, stable end-products of NO, was also decreased by LEP (Fig. 7C). Together, these evidences indicated that LEP mitigated oxido-nitrosative stress induced by DSS in the intestinal tissue.
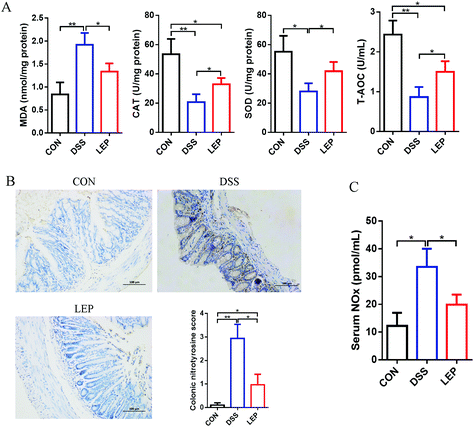 |
| Fig. 7 Effects of LEP administration on oxidative stress and nitrosative stress parameters in DSS-induced colitic mice. (A) Levels of colonic oxidative stress parameters MDA, CAT, SOD and T-AOC were measured. (B) Representative images of nitrotyrosine immunostaining in colonic sections. (C) Serum NOx was determined by Griess reaction. Data are expressed as mean ± SD (n = 3). *p < 0.05 and **p < 0.01. | |
4. Discussion
IBD is a kind of chronically recurrent gastrointestinal inflammatory disease. With the incidence rapidly increasing in the world, IBD has been regarded as a public health issue.28 Current therapeutic strategies for IBD are not satisfactory, the restrictions of which including side effects, high recurrence, high price, etc. As a natural product, polysaccharides are endowed with a multitude of bioactivities as well as invisible toxicity, making them one of the optimal candidates for rehabilitative intervention of disease. Previous literatures demonstrated that Lachnum polysaccharides showed anti-tumor, immunomodulatory, antioxidant and anti-inflammatory effects.14,15,29 However, the mitigative effect of Lachnum polysaccharide on IBD is still unknown. In this study, for the first time, we assessed the ameliorative effect of LEP at the pharmaceutical dose of 200 mg kg−1 on the symptoms of DSS-induced colitis mice, and investigated the potential underlying mechanism.
Accumulating evidences indicated that intestinal barrier plays a crucial role in protecting intestinal health. The impaired intestinal barrier function has been considered as the first event occurring in inflamed intestinal tissues of patients with IBD, which results in increased permeability of intestinal mucosa to endotoxins and antigens, and ultimately leading to strong and chronic inflammatory response.30 In this study, LEP treatment not only alleviated histopathologic damage induced by DSS, but also decreased the serum levels of LPS and LBP, indicators of colonic barrier integrity,13 indicating that LEP treatment reduced the abnormal barrier impairment. Afterwards, the underlying mechanism was investigated. Colonic TJ proteins are essential mechanical barrier, which have a key role in regulating intestinal integrity and permeability.31 In IBD patients, decreased and structural abnormity of TJ proteins were observed, which are currently emerged as a novel target for IBD therapy.32 As expected, expression of TJ proteins was abolished by DSS. However, this change was restored after LEP treatment. In addition to TJ proteins levels, the localization and expression of claudin-3 and claudin-7, known as the major isoforms of the claudin family, were relocated in junctional and basolateral regions of the epithelial cells. Moreover, mucus layer protecting proteins, antimicrobial proteins as well as the number and size of goblet cells were significantly increased after LEP treatment, indicating that goblet cell function and inner mucus layer production were promoted by LEP.33 These results suggested that LEP restored intestinal barrier integrity and relieving the damage to the colon.
It is well known that abnormal inflammatory response is one of the most striking features of IBD. In this study, intestinal function and intestinal barrier integrity were restored by LEP, which could abrogate the infiltration of foreign substances such as LPS and pernicious bacteria, and therefore repress inflammatory response.32 Regulation of pro-inflammatory mediators release is a pivotal therapeutic strategy to mitigate the inflammatory process. In line with LPS and LBP, the enhanced levels of pro-inflammatory factors, such as TNF-α, IL-1β and IL-6, in serum and colon tissues were inhibited by LEP administration. Next, the mechanism by which LEP inhibited pro-inflammatory cytokines production was investigated.
Like other nuclear receptor superfamilies, PPARγ is a transcription regulator, which acts as a vital anti-inflammatory mediator in alleviating the pathogenic condition of many diseases.34 Literatures have indicated that overexpression of PPARγ is capable to attenuate colitis, whereas suppression of PPARγ increases susceptibility to colitis.35 Our previous studies have shown that Lachnum polysaccharide significantly up-regulated PPARγ in adipose tissue of type 2 diabetic mice.18 Consistently, in this study it was found that PPARγ was strikingly up-regulated by LEP. Numerous studies have indicated that nitric oxide (NO) and prostaglandin E2 (PGE2), two important pro-inflammatory mediators which are generated by nitric oxide synthase (iNOS) and Cyclooxygenase-2 (COX-2), respectively, are closely related to inflammation.12 In this study, the inhibitory impact of LEP on NO and PGE2 production as well as their synthesized enzymes (iNOS and COX-2) were observed. Our previous results have indicated that Lachnum polysaccharide exerted anti-inflammatory effect to prevent liver damage by inhibiting NF-κB activation in carbon tetrachloride-induced acute liver injury mice model.15 It is well known that NF-κB is an important regulatory transcription factor, which regulates a series of pro-inflammatory enzymes and cytokines production, including iNOS, COX-2, IL-1β, IL-6 and TNF-α.5 Aberrant activation of NF-κB in intestinal epithelial cells has a close connection with IBD in both humans and animals. It has been reported that PPARγ, as an upstream target of NF-κB, alleviates intestinal inflammation by negatively regulating NF-κB activation and inhibiting inflammatory cytokines secretion.36 Above evidences inspired us that NF-κB might play a key role in anti-inflammatory effect of LEP. As expected, LEP treatment decreased the protein levels of the phosphorylation of NF-κB p65 and IκB, indicating that NF-κB signal pathway was inhibited. As a key downstream molecular of IL-6 signal pathway, STAT3 was demonstrated to promote the development of colitis, the state of which was strongly activated in DSS-induced colitis like NF-κB.37 Consistent with IL-6, increased expression of p-STAT3 was observed in DSS-induced colitis; however, this change was abolished after LEP administration. These results suggested the anti-inflammatory effect of LEP was achieved by regulating PPARγ/NF-κB and IL-6/STAT3 pathways.
Clinical studies have demonstrated that in response to DSS, the infiltration of various immune cells in colon tissues was regarded as one of the principal factors contributing to colitis severity.30 The activity of MPO was deemed as a marker of inflammatory response in damaged tissues the extent neutrophil infiltration.38 In the present study, colon MPO activity was decreased after LEP treatment, so did neutrophil accumulation in colon tissues. In addition to neutrophil, recruitment of macrophages provides a vital source of pro-inflammatory mediators and thereby aggravates the pathophysiology of IBD.39 In line with neutrophil, a sharp decrease of F4/80 positive macrophages was observed in LEP group. DSS-induced colitis model has been used to explore the abnormal immunological response in intestinal inflammation and simulate human UC which is exaggerated Th2 immune response based.40 The present results showed that Th2 cells (CD4+IL-10+) infiltration was considerably inhibited by LEP. These results indicated that the anti-inflammatory effect of LEP might also be attributed to reduced infiltration of inflammatory cells.
Unlike other pro-inflammatory cytokines, the production of bioactive IL-1β relies on inflammasome activation.31 Previous study has demonstrated that NLRP3/ASC/caspase-1 cascade is essential for bioactive IL-1β secretion and colitis development.41 Therefore, we suspected LEP might have an anti-IBD role by regulating NLRP3 inflammasome. In the present study, NLRP3 inflammasome was found significantly activated in DSS-induced IBD mice, which was consistent with previous results.31 However, LEP inhibited inflammasome activation as evidenced by decreased protein levels of NLRP3, ASC, caspase-1 and IL-1β. These data indicated that the beneficial role of LEP might be due to suppression of NLRP3 inflammasome activation.
Endoplasmic reticulum (ER) stress is considered as one of the pathogeneses of intestinal inflammation and tumorigenesis. Inflammation-driven ER stress responses are known to aggravate the condition of IBD pathology.42 Mice deficient IRE1 and XBP1 gene were more susceptive to DSS-induced colitis.43 Moreover, some studies have suggested that suppression NF-κB activation is not sufficient to inhibit colitis, ER stress pathway might be one of the pivotal factors in IBD.42 The downstream signaling pathway activated by ER stress has been studied for a long time. Protein misfolding is recognized by chaperone BiP/GRP78, which plays a central role in regulating the sensitivity and duration of the UPR.44 Consequently, BiP/GRP78 released from the stable complexes (also known as sensors) with PERK, ATF6 and IRE1α, and subsequently initiated UPR signaling cascades.27 Our results showed that protein level of PERK as well as the downstream key molecule (CHOP) was strikingly decreased after LEP administration. However, expressions of IRE1α and XBP1 were considerably up-regulated after LEP administration compared to DSS group, indicating that IRE1α might be activated by LEP to maintain the intestinal epithelial homeostasis and defend against IBD.45 Apoptosis induced by ER stress is achieved by promoting pro-apoptotic protein expression. Consistent with previous results,46 decreased protein level of cleaved-caspase 3 was observed in the colon tissues after LEP treatment. These data showed that LEP might reduce DSS-induced cell apoptosis and inflammatory response by attenuating ER stress through activating IRE1α-XBP1 and inhibiting PTEN-CHOP branch.
In addition to aberrant inflammatory response, oxidative stress of the intestinal tract has been considered as a pivotal event during IBD pathological progression. Excessive inflammation augments oxidative stress and nitrosative stress by stimulating reactive oxygen species (ROS) and reactive nitrogen species (RNS) generating systems, thereby causing tissue damage and exacerbation of patient's condition.32 Moreover, increased oxidative/nitrosative stress has been associated with extensive infiltration of immune cells, and activation of NF-κB signaling and ER stress.11,27 Previously, we have demonstrated that LEP alleviated oxidative damage caused by cyclophosphamide,14 however the protection role of LEP on oxidative stress injury in IBD is still unknown. Subsequently, key biomarkers of oxidative stress were determined to verify whether LEP could alleviate oxidative stress injury in DSS-induced colitis. Consistent with previous results, it was found that DSS exposure evidently induced oxidative stress, indicated by the elevated MDA level, and decreased CAT, SOD and T-AOC activity.11,32 On the contrary, these changes were inhibited after LEP treatment. Furthermore, abnormally elevated nitrosative stress markers, colon nitrotyrosine level and serum NOx concentration, were abolished by LEP. These results indicated that the protective effect of LEP on intestinal oxidative stress damage might be attributed to enhanced antioxidant defense system.
5. Conclusion
For the first time, these results demonstrated the protective effect of dietary LEP against the development of colonic inflammation in DSS-induced colitis mice. Multiple factors are involved in the protection role of LEP. LEP treatment alleviated the intestinal barrier defects induced by DSS through regulating tight junction proteins, mucus layer protecting proteins and antimicrobial proteins. Moreover, aberrant inflammatory response, inflammatory cells infiltration as well as NLRP3 inflammasome activation were abrogated by LEP treatment. Excessive ER stress and oxidative/nitrosative stress caused by DSS exposure were mitigated after LEP administration. Overall, these findings provided a solid scientific basis for utilization of LEP as an effective auxiliary adjuvant for maintaining intestinal homeostasis.
Conflicts of interest
The authors declare no conflict of interest.
Acknowledgements
This work was supported by the National Natural Science Foundation of China [No. 31470146].
References
- Y. Wang, N. Zhang, J. Kan, X. Zhang, X. Wu, R. Sun, S. Tang, J. Liu, C. Qian and C. Jin, Structural characterization of water-soluble polysaccharide from Arctium lappa and its effects on colitis mice, Carbohydr. Polym., 2019, 213, 89–99 CrossRef CAS.
- Y. S. Chiou, J. L. Ma, S. Sang, C. T. Ho, Y. J. Wang and M. H. Pan, Peracetylated (-)-Epigallocatechin-3-gallate (AcEGCG) Potently Suppresses Dextran Sulfate Sodium-Induced Colitis and Colon Tumorigenesis in Mice, J. Agric. Food Chem., 2012, 60, 3441–3451 CrossRef CAS.
- Y. Kobayashi, J. Kovacs-Nolan, T. Matsui and Y. Mine, The Anti-atherosclerotic Dipeptide, Trp-His, Reduces Intestinal Inflammation through the Blockade of L-Type Ca2+ Channels, J. Agric. Food Chem., 2015, 63, 6041–6050 CrossRef CAS.
- M. E. R. Andrade, P. A. V. d. Barros, P. L. d. R. Menta, G. M. F. Costa, S. E. M. Miranda, P. C. L. Leocádio, C. M. d. Almeida-Leite, S. d. V. Generoso, J. I. A. Leite and V. N. Cardoso, Arginine supplementation reduces colonic injury, inflammation and oxidative stress of DSS-induced colitis in mice, J. Funct. Foods, 2019, 52, 360–369 CrossRef CAS.
- T. Guo, Q. Lin, X. Li, Y. Nie, L. Wang, L. Shi, W. Xu, T. Hu, T. Guo and F. Luo, Octacosanol Attenuates Inflammation in Both RAW264.7 Macrophages and a Mouse Model of Colitis, J. Agric. Food Chem., 2017, 65, 3647–3658 CrossRef CAS.
- Y. Mayangsari and T. Suzuki, Resveratrol Ameliorates Intestinal Barrier Defects and Inflammation in Colitic Mice and Intestinal Cells, J. Agric. Food Chem., 2018, 66, 12666–12674 CrossRef CAS.
- M. Jin, Y. Wang, X. Yang, H. Yin, S. Nie and X. Wu, Structure characterization of a polysaccharide extracted from noni (Morinda citrifolia L.) and its protective effect against DSS-induced bowel disease in mice, Food Hydrocolloids, 2019, 90, 189–197 CrossRef CAS.
- T. Hu, Q. Lin, T. Guo, T. Yang, W. Zhou, X. Deng, J. K. Yan, Y. Luo, M. Ju and F. Luo, Polysaccharide isolated from Phellinus linteus mycelia exerts anti-inflammatory effects via MAPK and PPAR signaling pathways, Carbohydr. Polym., 2018, 200, 487–497 CrossRef CAS.
- M. T. Pacheco, T. Vezza, P. Diez-Echave, P. Utrilla, M. Villamiel and F. J. Moreno, Anti-inflammatory bowel effect of industrial orange by-products in DSS-treated mice, Food Funct., 2018, 9, 4888–4896 RSC.
- J. Wei and J. Feng, Signaling pathways associated with inflammatory bowel disease, Recent Pat. Inflammation Allergy Drug Discovery, 2010, 4, 105–117 CrossRef CAS.
- Y. Ji, Z. Dai, S. Sun, X. Ma, Y. Yang, P. Tso, G. Wu and Z. Wu, Hydroxyproline Attenuates Dextran Sulfate Sodium-Induced Colitis in Mice: Involvment of the NF-κB Signaling and Oxidative Stress, Mol. Nutr. Food Res., 2018, 62, 1800494 CrossRef.
- M. Marin, C. Gimeno, R. M. Giner, J. L. Rios, S. Manez and M. A. C. Recio, Influence of Dimerization of Apocynin on Its Effects in Experimental Colitis, J. Agric. Food Chem., 2017, 65, 4083–4091 CrossRef CAS.
- A. Kawabata, T. Van Hung, Y. Nagata, N. Fukuda and T. Suzuki, Citrus kawachiensis Peel Powder Reduces Intestinal Barrier Defects and Inflammation in Colitic Mice, J. Agric. Food Chem., 2018, 66, 10991–10999 CrossRef CAS.
- S. Zong, J. Li, L. Yang, Q. Huang, Z. Ye, G. Hou and M. Ye, Synergistic antitumor effect of polysaccharide from Lachnum sp. in combination with cyclophosphamide in hepatocellular carcinoma, Carbohydr. Polym., 2018, 196, 33–46 CrossRef CAS.
- L. Jing, S. Zong, J. Li, M. Ye, M. Surahio and L. Yang, Potential mechanism of protection effect of exopolysaccharide from Lachnum YM406 and its derivatives on carbon tetrachloride-induced acute liver injury in mice, J. Funct. Foods, 2017, 36, 203–214 CrossRef CAS.
- L. Dubuquoy, S. Dharancy, S. Nutten, S. Pettersson, J. Auwerx and P. Desreumaux, Role of peroxisome proliferator-activated receptor γ and retinoid X receptor heterodimer in hepatogastroenterological diseases, Lancet, 2002, 360, 1410–1418 CrossRef.
- M. Sánchez-Hidalgo, A. R. Martín, I. Villegas and C. Alarcón De La Lastra, Rosiglitazone, an agonist of peroxisome proliferator-activated receptor gamma, reduces chronic colonic inflammation in rats, Biochem. Pharmacol., 2005, 69, 1733–1744 CrossRef PubMed.
- Y. Wang, N. Su, G. Hou, J. Li and M. Ye, Hypoglycemic and hypolipidemic effects of a polysaccharide from Lachnum YM240 and its derivatives in mice induced by high fat diet and low dose STZ, MedChemComm, 2017, 8, 680–689 RSC.
- P. Xu, R. Yuan, G. Hou, J. Li and M. Ye, Structural Characterization and In Vitro Antitumor Activity of a Novel Exopolysaccharide from Lachnum YM130, Appl. Biochem. Biotechnol., 2018, 185, 541–554 CrossRef CAS PubMed.
- S. Zong, J. Li, L. Yang, Q. Huang, G. Hou, Z. Ye and M. Ye, Mechanism of bioactive polysaccharide from Lachnum sp. acts synergistically with 5-fluorouracil against human hepatocellular carcinoma, J. Cell. Physiol., 2019, 234, 15548–15562 CrossRef CAS PubMed.
- H. Cao, J. Liu, P. Shen, J. Cai and Y. Cao, Protective Effect of Naringin on DSS-Induced Ulcerative Colitis in Mice, J. Agric. Food Chem., 2018, 66, 13133–13140 CrossRef CAS PubMed.
- G. Varga, M. Ugocsai, P. Hartmann, N. Lajkó, R. Molnár, S. Szűcs, D. K. Jász, D. Érces, M. Ghyczy and G. Tóth, Acetylsalicylic acid-tris-hydroxymethyl-aminomethane reduces colon mucosal damage without causing gastric side effects in a rat model of colitis, Inflammopharmacology, 2018, 26, 261–267 CrossRef CAS PubMed.
- Y. Sun, X. Shi, X. Zheng, S. Nie and X. Xu, Inhibition of dextran sodium sulfate-induced colitis in mice by baker's yeast polysaccharides, Carbohydr. Polym., 2019, 207, 371–381 CrossRef CAS PubMed.
- S. Ni, S. K. Clinton, L. Zhihua, W. Yongquan, K. M. Riedl, S. J. Schwartz, Z. Xiaoli, P. Zui and C. Tong, Strawberry phytochemicals inhibit azoxymethane/dextran sodium sulfate-induced colorectal carcinogenesis in Crj: CD-1 mice, Nutrients, 2015, 7, 1696–1715 CrossRef PubMed.
- L. Eicke, X. T. Sam and S. Andrea, Activation and regulation of the inflammasomes, Nat. Rev. Immunol., 2013, 13, 397–411 CrossRef PubMed.
- Z. Kezhong and R. J. Kaufman, From endoplasmic-reticulum stress to the inflammatory response, Nature, 2008, 454, 455–462 CrossRef PubMed.
- J. Gong, X. Z. Wang, T. Wang, J. J. Chen, X. Y. Xie, H. Hu, F. Yu, H. L. Liu, X. Y. Jiang and H. D. Fan, Molecular signal networks and regulating mechanisms of the unfolded protein response, J. Zhejiang Univ., Sci., B, 2017, 18, 1–14 CrossRef CAS.
- G. G. Kaplan and S. C. Ng, Understanding and Preventing the Global Increase of Inflammatory Bowel Disease, Gastroenterology, 2017, 152, 313–321 CrossRef PubMed.
- T. Chen, Y. Wang, J. Li, N. Su, M. M. Surhio, Y. Liu and Y. Ming, Phthaloyl modification of a polysaccharide from Lachnum YM262 and immunomodulatory activity, Process Biochem., 2016, 51, 1599–1609 CrossRef CAS.
- Y. Mayangsari and T. Suzuki, Resveratrol Ameliorates Intestinal Barrier Defects and Inflammation in Colitic Mice and Intestinal Cells, J. Agric. Food Chem., 2018, 66, 12666–12674 CrossRef CAS PubMed.
- H. Cao, J. Liu, P. Shen, J. Cai, Y. Han, K. Zhu, Y. Fu, N. Zhang, Z. Zhang and Y. Cao, Protective Effect of Naringin on DSS-Induced Ulcerative Colitis in Mice, J. Agric. Food Chem., 2018, 66, 13133–13140 CrossRef CAS PubMed.
- Z. Zhang, S. Li, H. Cao, P. Shen, J. Liu, Y. Fu, Y. Cao and N. Zhang, The protective role of phloretin against dextran sulfate sodium-induced ulcerative colitis in mice, Food Funct., 2019, 10, 422–431 RSC.
- M. Wlodarska, B. Willing, K. M. Keeney, A. Menendez, K. S. Bergstrom, N. Gill, S. L. Russell, B. A. Vallance and B. B. Finlay, Antibiotic treatment alters the colonic mucus layer and predisposes the host to exacerbated Citrobacter rodentium-induced colitis, Infect. Immun., 2011, 79, 1536–1545 CrossRef CAS PubMed.
- Y. Gaofeng, C. Xiaoe and L. Duo, Modulation of peroxisome proliferator-activated receptor gamma (PPAR γ) by conjugated fatty acid in obesity and inflammatory bowel disease, J. Agric. Food Chem., 2015, 63, 1883–1895 CrossRef PubMed.
- M. Adachi, R. Kurotani, K. Morimura, Y. Shah, M. Sanford, B. B. Madison, D. L. Gumucio, H. E. Marin, J. M. Peters, H. A. Young and F. J. Gonzalez, Peroxisome proliferator activated receptor gamma in colonic epithelial cells protects against experimental inflammatory bowel disease, Gut, 2006, 55, 1104–1113 CrossRef CAS PubMed.
- Y. Fu, J. Ma, X. Shi, X.-Y. Song, Y. Yang, S. Xiao, J. Li, W.-J. Gu, Z. Huang, J. Zhang and J. Chen, A novel pyrazole-containing indolizine derivative suppresses NF-κB activation and protects against TNBS-induced colitis via a PPAR-γ-dependent pathway, Biochem. Pharmacol., 2017, 135, 126–138 CrossRef CAS PubMed.
- Y. Ji, Z. Dai, S. Sun, X. Ma, Y. Yang, P. Tso, G. Wu and Z. Wu, Hydroxyproline Attenuates Dextran Sulfate Sodium-Induced Colitis in Mice: Involvment of the NF-κB Signaling and Oxidative Stress, Mol. Nutr. Food Res., 2018, 62, 1800494 CrossRef PubMed.
- M. Marin, C. Gimeno, R. M. Giner, J. L. Rios, S. Mañez and M. C. Recio, Influence of dimerization of apocynin on its effects in experimental colitis, J. Agric. Food Chem., 2017, 65, 4083–4091 CrossRef CAS PubMed.
- H. S. P. De Souza and F. Claudio, Immunopathogenesis of IBD: current state of the art, Nat. Rev. Gastroenterol. Hepatol., 2016, 13, 13–27 CrossRef CAS PubMed.
- T. Vezza, F. Algieri, J. Garrido-Mesa, M. P. Utrilla, M. E. Rodríguez-Cabezas, A. Baños, E. Guillamón, F. García, A. Rodríguez-Nogales and J. Gálvez, The Immunomodulatory Properties of Propyl-Propane Thiosulfonate Contribute to its Intestinal Anti-Inflammatory Effect in Experimental Colitis, Mol. Nutr. Food Res., 2019, 63, 1800653 CrossRef PubMed.
- C. Bauer, P. C. Duewell, H. A. Lehr, K. A. Fitzgerald, M. Dauer, J. Tschopp, S. Endres, E. Latz and M. Schnurr, Colitis induced in mice with dextran sulfate sodium (DSS) is mediated by the NLRP3 inflammasome, Gut, 2010, 59, 1192–1199 CrossRef CAS PubMed.
- D. H. Kim, S. Kim, J. H. Lee, J. H. Kim, X. Che, H. W. Ma, D. H. Seo, T. I. Kim, W. H. Kim, S. W. Kim and J. H. Cheon, Lactobacillus acidophilus suppresses intestinal inflammation by inhibiting endoplasmic reticulum stress, J. Gastroenterol. Hepatol., 2019, 34, 178–185 CrossRef CAS PubMed.
- A. D. Garg, A. Kaczmarek, O. Krysko, P. Vandenabeele, D. V. Krysko and P. Agostinis, ER stress-induced inflammation: does it aid or impede disease progression?, Trends Mol. Med., 2012, 18, 589–598 CrossRef CAS PubMed.
- B. Zou, G. Xiao, Y. Xu, J. Wu, Y. Yu and M. Fu, Persimmon vinegar polyphenols protect against hydrogen peroxide-induced cellular oxidative stress via Nrf2 signalling pathway, Food Chem., 2018, 255, 23–30 CrossRef CAS PubMed.
- H. S. Zhang, Y. Chen, L. Fan, Q. L. Xi, G. H. Wu, X. X. Li, T. L. Yuan, S. Q. He, Y. Yu, M. L. Shao, Y. Liu, C. G. Bai, Z. Q. Ling, M. Li, Y. Liu and J. Fang, The Endoplasmic Reticulum Stress Sensor IRE1alpha in Intestinal Epithelial Cells Is Essential for Protecting against Colitis, J. Biol. Chem., 2015, 290, 15327–15336 CrossRef CAS PubMed.
- F. Shen, J. Feng, X. Wang, Z. Qi, X. Shi, Y. An, Q. Zhang, C. Wang, M. Liu, B. Liu and L. Yu, Vinegar Treatment Prevents the Development of Murine Experimental Colitis via Inhibition of Inflammation and Apoptosis, J. Agric. Food Chem., 2016, 64, 1111–1121 CrossRef CAS PubMed.
Footnote |
† Electronic supplementary information (ESI) available. See DOI: 10.1039/c9fo02719j |
|
This journal is © The Royal Society of Chemistry 2020 |
Click here to see how this site uses Cookies. View our privacy policy here.