DOI:
10.1039/D0MA00698J
(Paper)
Mater. Adv., 2020,
1, 3460-3465
Green synthesis of Zn-doped Catharanthus Roseus nanoparticles for enhanced anti-diabetic activity
Received
12th September 2020
, Accepted 9th November 2020
First published on 10th November 2020
Abstract
Recently, many studies have been interested in the bio-production of zinc oxide nanoparticles (ZnO-NPs) for biomedical applications using plants. However, green synthesized zinc-based nanoparticles (Zn-NPs) have not been reported previously. In this research, for the first time, we have prepared eco-friendly Zn-doped Catharanthus Roseus (C. roseus) plants using a facile photon induced method (PIM) for green biomaterials. Most importantly, the presence of phytoconstituents in the leaf extract of C. roseus plays a vital role in the development of NPs, as the phytoconstituents serve as reducing agents, which can be efficiently used for enhanced anti-diabetic performance. X-ray diffraction (XRD) and energy dispersive spectroscopy (EDX) results confirmed the fabrication of the biosynthesized Zn-doped C. roseus plant. The prepared NPs revealed high crystallinity with a shifted band gap energy of 2.74 eV in the visible region. Fourier transform infrared spectroscopy (FTIR) showed Zn-doped C. roseus surfaces decorated with functional groups. The transmission electron microscopy (TEM) images showed that the NPs are uniformly dispersed in a spherical shape with sizes ranging from 10 to 20 nm. In addition, an alpha-amylase inhibitory assay was employed to assess the anti-diabetic nature of the prepared Zn-doped C. roseus extract, and this was compared with a standard drug (Acarbose). The results showed that the biosynthesized NPs had encouraging inhibitory effects on the alpha-amylase (α-1,4-glucan-4-glucanohydrolase) enzyme. Therefore, the significant results of this work are the generation of value-added drugs from the C. roseus plant for biotechnology and nanomaterial-based industries.
1. Introduction
In recent years, the use of green NPs has been addressed in playing a significant role in medicine, agriculture and solar cell fields. Therefore, obtaining new synthesis methods using biological systems for the fabrication of nanoparticles can pave a promising pathway for biomedical and nanotechnology-based industries.1–4 Researchers have carried out investigations to explore a low-environmental impact method for developing well-characterized NPs. Different low-cost and low-environmental impact approaches for preparing NPs have emerged as alternatives to traditional synthetic processes.5 One of the most considered techniques is the synthesis of NPs using organisms. Among all organisms, plants appear to be the best candidates for this and they are appropriate for the up-scaled bio-production of NPs.6 NPs synthesized by extracts are more stable and the rate of production is faster than in the case of microorganisms. In addition, extracts of medicinal plants are often utilized as stabilizing and reducing materials in the production of metallic NPs.7–9
The green preparation of NPs using extracts of medicinal plants has been employed to control the shapes of biosynthesized NPs, which determines their optical and biological features.10–14 In this regard, green NPs have been produced in recent years using diverse plants such as neem, alfalfa, Emblica Officinalis, lemongrass, tamarind, and Euphorbiatirucalli.15 Azeez and co-workers reported the biosynthesis of ZnO NPs using Punica granatum (pomegranate) juice extract as an efficient chelating and capping agent.16 In another study, ZnO NPs were prepared in a green method, employing Eucalyptus globulus Labill. leaf extract and zinc nitrate hexahydrate salt, and this showed good merits for possible medical applications.17 Himdad and co-workers reported the biosynthesis of ZnO NPs employing the Apium graveolens L. leaf plant and the prepared ZnO NPs revealed good properties for removing organic pollutants.18 Among plants, C. roseus is an important medicinal plant distributed throughout the world that can be utilized to synthesize NPs from metal solutions and can serve as an excellent nano-factory. The attention towards this extract is due to the fact that it possesses monomeric indole alkaloids: vindoline and catharanthine, as its most abundant constituents, and these have important medicinal values.19 Therefore, this material has considerable pharmacological merits such as antifungal,20 anticancer,21 antioxidant22 and antibacterial activities.23 Hence, it is highly suitable to produce effective eco-friendly NPs from the C. roseus plant without the addition of hazardous acid solvent, surface passivation chemicals or a difficult post-treatment process.
Amongst the NPs, ZnO-NPs exhibit considerable semiconducting merits because of their high exciton binding energy and the fact that they possess a wide bandgap at 3.37 eV, and so can be used for optical, biological, gas sensing and medical applications. Furthermore, they are employed by industries, such as in wastewater treatment, and as pharmaceutical products for drug delivery, with anti-cancer, anti-diabetic, and antibacterial activities, as well as anti-fungal properties.24–29 However, the drawbacks of the toxicity of ZnO-NPs towards biological systems remains a controversial problem within recent reports.30 We believe that nanotechnology will dramatically improve the evolution of medicine.
Notably, no previous study has been reported in the literature on the biosynthesis of Zn-doped C. roseus. The present study was designed in a facile, eco-friendly manner, and it was cost-effective in the synthesis of Zn-doped C. roseus NPs using the C. roseus extract as a green precursor, and we have investigated the anti-diabetic activities of the NPs. The oxygen functional groups, size and crystallinity of Zn-doped C. roseus were recorded by FTIR, TEM, and XRD. Our findings showed that C. roseus-based Zn NPs have high anti-diabetic activity.
2. Experimental
2.1. Materials
A fresh part of C. roseus was collected from the Dharmapuri area of Agragaram, Dharmapuri District, Tamilnadu, India. Zinc sulfate monohydrate (99%, ZnSO4), α-amylase, acarbose, iodine, and hydrochloric acid (HCl) were ordered from Sigma-Aldrich. Distilled water (DW) was provided from Evergreen EG Laboratory, Chennai in India.
2.2. Preparation of green NPs
Zn-doped C. roseus NPs were biosynthesized using PIM. In brief, fresh C. roseus extracts were cleaned with DW and ground up. Then, 30 g of the C. roseus fine plant solution and 3 g of ZnSO4 were added to 1000 ml of DW. The mixed dispersion was stirred for 5 days under a 500 W Halogen light and then kept in an air atmosphere for 20 days under solar light. Finally, the collected powder was dried and calcinated at 100 °C for 1 h.
2.3. Characterization
The XRD pattern of the NPs was recorded on a Bruker D8 Advance powder diffractometer with Cu Kα (λ = 0.15406 nm) radiation. The shape, size, and elemental composition were visualized using field emission scanning electron microscopy (FESEM, Hitachi S-4800), TEM (JEM-2100F), and EDX, respectively. The FTIR pattern of the NPs was measured using a KBr pellet approach on a Bruker Tensor 27 spectrometer. Z-Average and zeta potential measurements were recorded using dynamic light scattering (DLS) analysis (Horiba). Ultraviolet-visible diffuse reflectance spectroscopy (UV-DRS) of the sample was determined with a PerkinElmer Lambda 25 spectrophotometer.
2.4. Alpha-amylase assay
The α-amylase inhibitory effect of the green NPs (Zn-doped C. roseus) was performed using the standard protocol with little modification.31 First, 100 μl of α-amylase material (0.1 mg ml−1) was mixed with various amounts (10, 20, 40, 80, 160, and 320 μg ml−1) of the green NPs, a standard (acarbose), and a control (without standard/test samples), and the samples were then pre-incubated at 37 °C for 20 min. 100 μl of starch solution was added to initiate the reaction and the samples were incubated at 37 °C for 60 min. Subsequently, 10 μl of 1 M HCl and 100 μl of iodine were added to the reaction tubes. The absorbance values of the dispersions were recorded at 580 nm and the α-amylase inhibitory effect was evaluated using the following equation:31
% of Inhibition = [(OD of test − OD of control)/OD of test] × 100 |
3. Results and discussion
The crystal nature of Zn-doped C. roseus NPs was determined by XRD patterns, as shown in Fig. 1a. The XRD was performed in the range of 10–70° and most of the diffraction peaks can be attributed to Zn-doped C. roseus NPs, according to previous reports.32 The broadening of the peaks implies the fabrication of Zn-doped C. roseus NPs within the nanometer range.33 XRD results corroborated well with those in previously reported literature.34 The highest intensity peak of the Zn-doped C. roseus NPs along with other smaller intensity peaks were observed. The average particle size of the Zn-doped C. roseus NPs was found to be 16 nm, using Debye Scherrer's formula.35 These XRD findings prove the purity of the synthesized Zn-doped C. roseus NPs. An FTIR spectroscopic study (Fig. 1b) was carried out to investigate the plausible mechanism behind the formation of Zn-doped C. roseus NPs. As can be seen, the FTIR shows the availability of functional groups at 3420 cm−1, corresponding to the O–H stretch of H–bonded amino acids, and at 2848 cm−1 for the O–H stretching of the –CH2 groups. The absorption bands were centered at wavenumbers of 2922 cm−1 and 1630 cm−1, corresponding to the vibrational stretches of C–H and the carboxyl groups (asymmetrical COO− stretching vibration), respectively, and at 1410 cm−1 due to the symmetrical COO− stretching vibration.36 Very weak bands centered at 1058 cm−1 correspond to C–O stretched aromatic amines and aliphatic amines.37 The plant extract serves as a reducing and stabilizing material in the conversion of the plant to green NPs. The strong absorption band at 612 cm−1 is due to the Zn-doped C. roseus NPs.38–41 The functional groups present in the plant extract contribute to the mechanism of bonding within the Zn NPs. Any shift or change in the location or intensity of the bands in the spectra of the NPs can be correlated with the interaction of the oxygen agents of the plant extract with the Zn NPs. It can therefore be deduced that oxygen agents in the plant donate electrons that can reduce Zn ions and finally obtain the Zn NPs. In addition, the negative oxygen groups present in the plant can have a stabilizing effect.
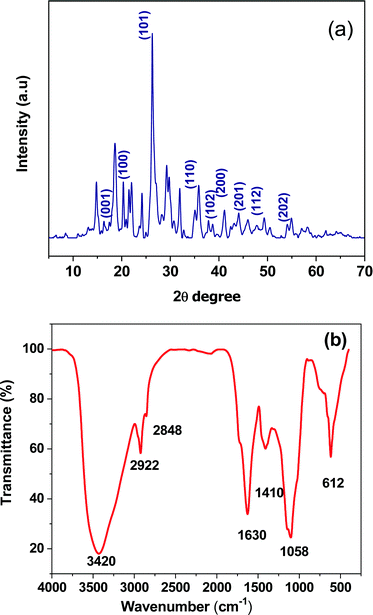 |
| Fig. 1 (a) XRD spectrum of the green synthesized Zn-doped C. roseus NPs. (b) FTIR spectrum of the Zn-doped C. roseus NPs. | |
The zeta potential value was determined to be −0.8 for the Zn-doped C. roseus NPs, as shown in Fig. 2. The zeta potential value provides us with evidence regarding the surface charge of the NPs, and this appeared to be more negative than previously reported.42–44 Additionally, it gives us an idea about the stability of the NPs and the zeta potential value obtained for the Zn-doped C. roseus NPs lies within the low stable range, implying that the green NPs are unstable in aqueous solution. Therefore, there may be possible aggregations due to residual surfactants.
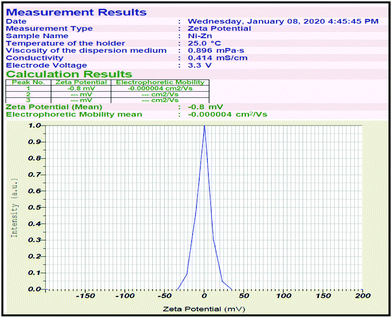 |
| Fig. 2 Zeta potential analysis of the Zn-doped C. roseus NPs. | |
The UV-DRS reflectance spectrum of Zn-doped C. roseus is shown in Fig. 3a. The bandgap energy of the prepared NPs was obtained using a Tauc plot (Fig. 3b).45 This demonstrated that the measured bandgap energy of the NPs is an indirect type with a value of 2.74 eV. The narrow bandgap in the Zn-doped C. roseus NPs, obtained by PIM, may be assigned to the upward shifting of the valence band. Therefore, it can be concluded that a smaller bandgap is more important for visible light absorption.
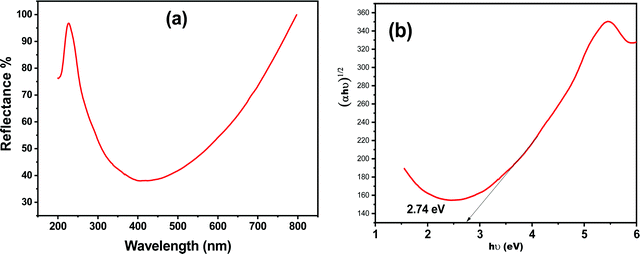 |
| Fig. 3 UV-DRS measurements of (a) reflectance and (b) the indirect bandgap of the Zn-doped C. roseus NPs. | |
The morphology of the Zn-doped C. roseus NPs was studied using FESEM and the results are shown in Fig. 4a. It can be seen that the surface of the sample appears uneven due to the domination of the co-precipitate of the metal with the plant. The particles are random in size and are irregular in shape. The FESEM image of the Zn-CR NPs exhibited sizes ranging between 15 and 20 nm, proving the existence of spherical Zn-doped C. roseus NPs (red circular area). The TEM image of the Zn-doped C. roseus NPs is presented in Fig. 4b. As observed, the TEM image shows agglomerated globular NPs. In addition, the results demonstrated that the biosynthesized NPs in this work are multi-dispersed with a particle size range of 10–20 nm. The extremely small particle size can obtain great specific surface area and surface energy. No mono-dispersion of NPs was observed. Due to the high surface energy, C. roseus NPs in an aqueous medium tend to form agglomerates.
 |
| Fig. 4 (a) FESEM and (b) TEM images of the Zn-doped C. roseus NPs. | |
Fig. 5 shows the elemental analysis of the Zn-doped C. roseus NPs using EDX mapping. The weight percentages of C, O, S, K, Ca, and Zn were 45%, 21%, 13%, 6%, 5% and 10%, respectively. These results confirm that a very small amount of Zn is present.
 |
| Fig. 5 EDS spectrum and inset of the mapping images, and the Smart Quant Results of the Zn-doped C. roseus NPs. | |
The α-1,4-glucan-4-glucanohydrolase is one of the most prominent secretory products of the pancreas and salivary gland. This is responsible for the hydrolysis of complex carbohydrates into oligosaccharides and disaccharides in the intestinal mucosa. These sugars are further digested to monosaccharides by the action of alpha-glucosidase. The α-amylase inhibitory studies performed in the Zn-doped C. roseus extract showed moderate inhibitory potential, as shown in Fig. 6a and b. The half maximal inhibitory concentration (IC50) value of the Zn-doped C. roseus extract is shown to have good α-amylase inhibitory activity. The IC50 value of the given sample was found to be 35.64165 μg ml−1, whilst the value of the standard drug (Acarbose) was 13.085434 μg ml−1. Herein, Zn serves as an actuator for blood sugar regulation, which is significantly affected in the presence of the Zn-doped C. roseus extract. The prepared Zn-doped C. roseus extract reveals an improved inhibitory effect for the treatment of diabetic issues and suppresses the fasting blood glucose level efficiently, due to the presence of amino acids in the Zn-doped C. roseus NPs.46 Thus, biosynthesized Zn-doped C. roseus NPs can form an effective starch blocker drug to treat this dysfunction. Many synthetic medicines have certain side effects such as abdominal bloating and diarrhea. According to the above findings, stable and biocompatible Zn NPs associated with insulin metabolism could be efficiently employed as a vital anti-diabetic drug.
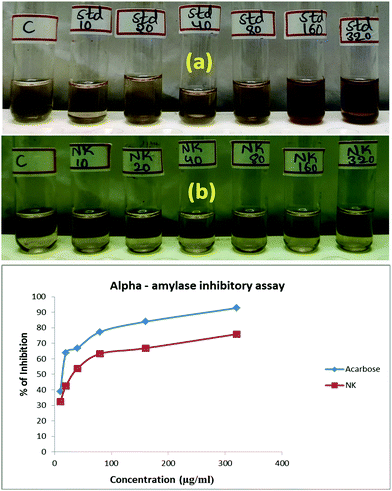 |
| Fig. 6 (a) Anti-diabetic activity of the standard and of (b) the Zn-doped C. roseus NPs. | |
4. Conclusion
Herein, we report a simple, green, and reproducible process for the environmentally-friendly preparation of a Zn-doped C. roseus plant for next-generation green biomedicine. As shown from the analytical results, the prepared Zn-doped C. roseus NPs had spherical morphology with a size of 10–20 nm, albeit in an aggregated form. In addition, the XRD results confirmed the production of crystalline NPs with a crystallite size of 16 nm. Herein, the biosynthesized NPs were tested for possible medical applications as anti-diabetic drugs. The Zn-doped C. roseus NPs showed good α-amylase inhibitory activity compared to the standard drug. Overall, it was clearly evident from our studies that Zn-doped C. roseus NPs can elicit potent anti-diabetic activity. Furthermore, these green NPs had no side effects compared with the standard sample. From the above results, we recommend that the biogenically synthesized green NPs can be used for different biological purposes, particularly in diabetes research.
Conflicts of interest
The authors declare no competing financial interest.
Acknowledgements
The authors thank Director Dr P. Mohana Sundram, PG Extension Centre, Periyar University, Dharmapuri-636107, Tamil Nadu, India.
References
- P. Zibin, L. Yuman, S. Binoy, O. Gary and C. Zuliang, J. Colloid Interface Sci., 2020, 558, 106–114 CrossRef.
- B. N. Rashmia, F. H. Sujatha, B. Avinashc, C. R. Ravikumarc, H. P. Nagaswarupad, M. R. A. Kumarc, K. Gurushanthae and M. S. Santoshf, Inorg. Chem. Commun., 2020, 111, 107580 CrossRef.
- D. S. Ahmed, M. K. A. Mohammed and S. M. Majeed, ACS Appl. Energy Mater., 2020 DOI:10.1021/acsaem.0c01896.
- M. Shakibaie, A.-E.-A. Fatemeh, A.-S. Mahboubeh, A. Atefeh, D. Mohsen, F. Hamid and A. Alieh, Nanomed. J., 2019, 6, 223–231 CAS.
- K. S. Virender, A. Y. Ria and L. Yekaterina, Adv. Colloid Interface Sci., 2009, 145, 83–96 CrossRef.
- P. Horky, S. Sylvie, U. Lenka, B. Daria, K. Silvia, B. Zuzana and K. Eliska, J. Anim. Sci. Biotechnol., 2019, 10(1), 17 CrossRef.
- B. Aderibigbe, Molecules, 2017, 22, 1370 CrossRef.
- M. A. Albrecht, C. W. Evans and C. L. Raston, Green Chem., 2006, 8, 417 RSC.
- M. Anbuvannan, M. Ramesh, G. Viruthagiri, N. Shanmugam and N. Kannadasan, Mater. Sci. Semicond. Process., 2015, 39, 621–628 CrossRef CAS.
- N. Bala, S. Saha, M. Chakraborty, M. Maiti, S. Das, R. Basu and P. Nandy, RSC Adv., 2014, 5, 4993–5003 RSC.
- G. Bhumi and N. Savithramma, Int. J. Drug Dev. Res., 2014, 6, 208–214 Search PubMed.
- T. Bhuyan, K. Mishra, M. Khanuja and R. Prasad, Mater. Sci. Semicond. Process., 2015, 32, 55–61 CrossRef CAS.
- M. Gupta, R. S. Tomar, S. Kaushik and R. K. Mishra, Front. Microbiol., 2018, 9, 1–13 CrossRef.
- S. K. Chaudhuri and L. Malodia, Appl. Nanosci., 2017, 7(8), 501–512 CrossRef CAS.
- H. R. Naika, K. Lingaraju, K. Manjunath, D. Kumar, G. Nagaraju, D. Suresh and H. Nagabhushana, J. Taibah Univ. Sci., 2014, 4–6 Search PubMed.
- A. B. Azeez, M. H. Samir, M. E. Mahera, K. A. Semih and H. S. H. Faiq, Micro Nano Lett., 2020, 15, 415–420 CrossRef.
- A. B. Azeez and H. A. Himdad, SN Appl. Sci., 2020, 2, 991 CrossRef.
- H. A. Himdad and A. B. Azeez, Desalin. Water Treat., 2020, 190, 179–192 CrossRef.
- M. T. Ansari, S. Farheen, A. K. Fatin, Z. A. Mohammad, A. S. Tengku, M. bin Tengku, M. Shahnaz and A. Sadath, Curr. Nanomed., 2018, 8, 225–233 CrossRef CAS.
- S. Roy and P. Chatterjee, Ind. Crops Prod., 2010, 32, 375–380 CrossRef CAS.
- S. Gajalakshmi, S. Vijayalakshmi and V. Devi Rajeswari, Int. J. Pharma Biol. Sci., 2013, 4, 821–830 Search PubMed.
- D. M. Pereira, J. Faria, L. Gaspar, F. Ferreres, P. Valentão, M. Sottomayor and P. B. Andrade, Food Chem., 2010, 121, 56–61 CrossRef CAS.
- A. B. Azeez, M. H. Samir and J. I. Haidar, Eurasian J. Sci. Eng., 2019, 4, 74–83 Search PubMed.
- H. Pavel, S. Sylvie, U. Lenka, B. Daria, K. Silvia, B. Zuzana, K. Eliska, L. Zuzana, C. Natalia, G. Milica, M. Vedran, S. Vendula, V. Eva, N. Pavel, K. Pavel, K. Olga, H. David, K. Pavel, S. Jiri, A. Vojtech and S. Kristyna, J. Anim. Sci. Biotechnol., 2019, 10, 17 CrossRef.
- S. Divyapriya, C. Sowmia and S. Sasikala, World J. Pharm. Pharm. Sci., 2014, 3, 1635–1645 Search PubMed.
- R. Dobrucka and J. Długaszewska, Saudi J. Biol. Sci., 2016, 23, 517–523 CrossRef CAS.
- R. Farzana, P. Iqra, F. Shafaq, S. Sumaira, K. Zakia, T. Hunaiza and M. Husna, Arch. Clin. Microbiol., 2017, 8, 57 Search PubMed.
- K. A. M. Mustafa, Optik, 2020, 217, 164867 CrossRef.
- K. A. M. Mustafa, S. A. Duha and R. M. Mohammad, Mater. Res. Express, 2019, 6, 055404 CrossRef.
- J. Jinhuan, P. Jiang and C. Jiye, Bioinorg. Chem. Appl., 2018, 2018, 1–18 Search PubMed.
- J. O. Unuofin, G. A. Otunola and A. J. Afolayan, Heliyon, 2018, 4(9), e00810 CrossRef.
- M. Gupta, R. S. Tomar, S. Kaushik, R. K. Mishra and D. Sharma, Front. Microbiol., 2018, 9 Search PubMed.
- S. P. Rajendran and K. Sengodan, J. Nanosci., 2017, 2017, 1–7 CrossRef.
- H. N. Fal and F. Farzaneh, J. Sci., Islamic Repub. Iran, 2006, 17(3), 231–234 Search PubMed.
- K. A. M. Mustafa, Plasmonics, 2020 DOI:10.1007/s11468-020-01224-5.
- S. A. Duha and K. A. M. Mustafa, Chem. Pap., 2020, 74, 4033–4046 CrossRef.
- R. M. Mohammad, D. S. Ahmed and K. A. M. Mustafa, J. Sol-Gel Sci. Technol., 2019, 90, 498–509 CrossRef.
- G. Nagaraj, D. Brundha, V. Kowsalya, C. Chandraleka, S. Sangavi, R. Jayalakshmi, M. Arulpriya, N. Sathya, M. Prasath and S. Tamilarasu, Mater. Today Proc., 2020 DOI:10.1016/j.matpr.2020.05.318.
- P. Jithendra, A. M. Rajam, T. Kalaivani, A. B. Mandal and C. Rose, ACS Appl. Mater. Interfaces, 2013, 5(15), 7291–7298 CrossRef CAS.
- F. Nejatzadeh-Barandozi and S. Enferadi, Org. Med. Chem. Lett., 2012, 2(1), 33 CrossRef.
- O. Saibuatong and M. Phisalaphong, Carbohydr. Polym., 2010, 79(2), 455–460 CrossRef CAS.
- M. Singh Rajput, V. Nair and A. Chauhan, Middle East J. Sci. Res., 2011, 7(5), 784–788 Search PubMed.
- P. P. Pillay, C. P. M. Nair and T. N. Santi Kumari, Bull. Res. Inst., Univ. Kerala, Trivandrum, Ser. A, 1959, 1, 51–54 Search PubMed.
- S. Chakraborty, N. Kar, L. Kumari, A. K. De and T. Bera, Int. J. Nanomed., 2017, 12, 4849–4868 CrossRef CAS.
- S. A. Duha, R. M. Mohammad and K. A. M. Mustafa, Nanosci. Nanotechnol., 2020, 10, 127–133 Search PubMed.
- D. J. Manasa, K. R. Chandrashekar, A. P. K. Masineni, S. Doddavenkatanna, P. Ashwini, P. D. Rekha and S. Sana, Appl. Nanosci., 2020, 10, 3057–3074 CrossRef.
|
This journal is © The Royal Society of Chemistry 2020 |
Click here to see how this site uses Cookies. View our privacy policy here.