DOI:
10.1039/D0NA00702A
(Paper)
Nanoscale Adv., 2020,
2, 5688-5698
Rhodium doping augments photocatalytic activity of barium titanate: effect of electronic structure engineering†
Received
23rd August 2020
, Accepted 4th November 2020
First published on 5th November 2020
Abstract
Environmentally friendly BaTiO3 is emerging as a potential photocatalyst due to its tunable electronic structure. Although originally believed to be a poor photocatalyst due to its wide band gap, several strategies have been implemented to reduce its band gap. One such approach is doping, but this often leads to the formation of mid gap recombination centers and diminishes the efficiency of the material. In the present work, we study for the first time the effect of site occupancy of Rh on the electronic structure of BaTiO3. As the theoretical results reveal that if Rh occupies both Ba and Ti sites simultaneously it leads to the formation of mid gap states, an experimental approach is implemented to reduce the band gap of BaTiO3 while simultaneously avoiding the formation of recombination centers. The facile one pot hydrothermal approach reported here directs the Rh towards Ba sites leading to a decrease in the band gap due to the appearance of donor Rh3+ states, suppressing the formation of Rh4+ states. A promising photocatalytic activity of 96% degradation of methylene blue dye in 120 minutes was observed for the 0.5 Rh sample indicating the high efficiency of the material.
Introduction
The current energy and environmental crises have led to immense interest in the field of photocatalysis, be it hydrogen production via water splitting or pollutant degradation by using visible light. Oxides have been the front runners in the field of photocatalysis due to their nontoxic, economical and stable nature.1,2 Since oxides possess a large band gap which prohibits absorption of the visible range of solar radiation, various strategies such as formation of composites with 2D materials, heterostructures and doping have been implemented to facilitate the process of photocatalysis.3–6 Among oxides, multi-cation oxides such as tungstates, titanates, and vanadates are a convenient choice as they can provide multiple sites for doping.7–10 It is well known that SrTiO3, a perovskite material, has been extensively studied for photocatalysis in degradation of methylene blue (MB) dye by tuning its band gap via doping, but only a few reports exist on BaTiO3.11–15
BaTiO3 is a versatile material with a highly tunable crystal and electronic structure and has the potential of being a multifunctional material.5,10,16,17 For a material to show enhanced photocatalytic properties it must have a band gap small enough to absorb visible radiation and generate electron–hole pairs, and the photogenerated charge carriers must have sufficient separation to prevent their recombination.18 So far, the piezoelectric effect of BaTiO3 has been used to enhance the separation of photogenerated charge carriers while it is also combined with some other plasmonic nanomaterials of Ag or Au to further meet the challenge of absorption of visible light radiation.10,19–21 Besides this, compositing with carbon-based materials has also been adopted to enhance the photocatalytic efficiency via promoting charge carrier separation.14,15,22,23 Various dopants such as Ce, Cr, Fe, In, Mn, Mo, Ni, N, Rh, W, and Zn have been used to reduce the band gap of BaTiO3 and bring its absorption from the UV to the visible region of the solar spectrum, thus improving its photocatalytic efficiency.4,6,13,17,24–29 Doped BaTiO3 has largely been used for the photocatalytic production of hydrogen rather than degradation of dyes.4,6,27,30 Doping is well known to largely affect the electronic structure and hence the properties associated with it depending on the site which it occupies.31,32 Although doping is a promising choice to reduce the band gap, in certain cases it may lead to the formation of acceptor levels leading to the recombination of charge carriers and hence poor efficiency.12,33 Therefore, we need to keep in mind that in addition to being stable, nontoxic and having an appropriate band gap, the recombination rate should be low with an increase in the lifetime of charge carriers for a material to be an ideal photocatalyst.18
Recently, we reported Rh to be an ideal dopant in SrTiO3 for photocatalytic degradation of MB and thermoelectric application and found that the site occupancy of the material plays a major role in the performance of the material.34,35 Although Rh has already been doped in BaTiO3, its efficiency as a photocatalyst has been tested only for hydrogen generation.36,37 Herein, we theoretically study the effect of Rh on the electronic structure of BaTiO3 and its effect on the band gap of the material. Based on the inputs from the first principles density functional theory (DFT) results, we devised a simple hydrothermal technique which directs Rh towards Ba sites which leads to the formation of donor levels and a decrease in the band gap. We carried out an in-depth analysis of the photocatalytic phenomenon taking place along with thorough characterization of the synthesized material. Since Rh is expensive we tried to make the final material considerably economical by trying to achieve higher efficiency for as low concentration as 0.5 Rh (mol%). 96% degradation was observed in 120 min and the high cycling stability reveals the high efficiency of the material as a photocatalyst in comparison to the 65.2% exhibited by 0.5 Rh doped in SrTiO3.34
Methods
Computational details
We simulated the electronic structure of pristine BaTiO3 and Rh-doped BaTiO3 using a 2 × 2 × 2 supercell of primitive BaTiO3. The first principles DFT calculations carried out using the Quantum ESPRESSO package considered the Perdew–Wang (PW) functional type of pseudopotential with local density approximation.38,39 The norm conserving pseudopotential considered 6s2, 3d24s2, 2s22p4, and 4d85s1 of Ba, Ti, O and Rh, respectively, as the valence electrons. The plane wave basis representing the wave functions was terminated with a high energy cutoff and charge density cutoffs of 120 Ry and 480 Ry, respectively. The Brillouin zone was sampled using k point meshes of 4 × 4 × 4 and 8 × 8 × 8 for self-consistent and non-self-consistent field calculations, respectively. The electronic structure was determined along the Γ − X − M − Γ − R − X high symmetry path.
Synthesis
All the chemicals were purchased from Sigma-Aldrich and were used as received without further purification. 0.35 g of titanium(IV) oxide, anatase (325 mesh powder), was added to 30 mL of 5 M KOH solution. To this, calculated amounts of rhodium(III) chloride and barium hydroxide (octahydrate) were added and stirred for one hour. The resultant mixture was sealed in an autoclave and kept in a hot air oven maintained at 180 °C for 20 hours. The as-obtained precipitates were washed thoroughly with acetic acid and water. The washed products were dried in an oven at 70 °C for 8 hours. The products obtained by using 0.1, 0.3, 0.5, 0.7 and 0.9 mol% of the Rh precursor were labeled 0.1 Rh, 0.3 Rh, 0.5 Rh, 0.7 Rh and 0.9 Rh, respectively.
Characterization
The crystallographic parameters of the synthesized materials were examined by using an X-ray diffractometer (XRD, Rigaku Miniflex 600) equipped with a monochromatic Cu Kα radiation (λ = 0.154 nm) at a scan rate of 2° min−1 in the range of 20–80°. The surface morphological features of the synthesized semiconductors were analyzed using transmission electron microscopy (TEM) and high-resolution transmission electron microscopy (HRTEM, JEOL/JEM 2100). A Kratos XSAM800 spectrometer equipped with an Al Kα source was used to record the X-ray photoelectron spectrum (XPS) to study the surface chemistry and valence states of the material. The specific surface area was determined using the Brunauer–Emmett–Teller (BET) method (BEL SORP II, JAPAN). The pore size distribution was determined using the Barrett–Joyner–Halenda (BJH) method. The diffuse reflectance (DR) spectra were recorded using a UV-visible spectrometer (DRS, DR SPECORD S600 Analytic Jena). The photoluminescence (PL) spectra were recorded at room temperature (LS-55, PerkinElmer).
Determination of photocatalytic activity
A photocatalytic reactor provided with a high-pressure 250 W Hg vapor lamp (used as a visible light source) was used for the photocatalytic degradation measurements. Before photocatalytic studies, the photocatalyst (50 mg) was dispersed in 50 mL of MB solution (10 mg L−1) with the help of a sonicator for 5 minutes. The resultant solution was loaded into the reactor and then the visible light source was turned on. 5 mL of the solution was withdrawn periodically, centrifuged in order to separate the catalyst and the absorbance of the supernatant dye solution was measured using a UV-visible spectrometer at 664 nm. The percentage degradation of dye was calculated as per eqn (1). | Degradation% = [(Co − C)/Co] × 100 | (1) |
where Co is the initial concentration of the solution and C is the concentration at different intervals of time.1,7
Results and discussion
Electronic structure analysis
BaTiO3, a perovskite material, has a cubic structure formed out of Ba atoms occupying the corner, a Ti atom occupying the center and O atoms occupying the 6 face centers.16 It is well known that in the electronic structure of the primitive BaTiO3 there exists a direct band gap at the Γ point while two indirect gaps appear from R → Γ and M → Γ. In the electronic structure of the 2 × 2 × 2 supercell of BaTiO3 we observe that a direct band gap of 1.929 eV appears at the Γ point (Fig. 1a). This is due to the folding of the R point of the primitive cell onto the Γ point of the 2 × 2 × 2 supercell.16 Hence, the indirect band gap between Γ and R points appears as a direct band gap at the Γ point. The original direct band gap at the Γ point of the primitive cell appears as the distance between the conduction band minimum and the second set of bands beneath the valence band maximum at the Γ point, measuring 2.304 eV.16 The band which exists between this and the valence band maximum is formed due to the overlapping of the M point of the primitive cell onto the Γ point of the supercell and measures 1.994 eV.16 The band gap is typically underestimated in DFT based calculations due to the presence of discontinuities in the derivative of energy.40 To understand the contributions from various atomic orbitals, we projected them onto the density of states (DOS). We see that the O ‘p’ orbitals form the valence band, while the Ti ‘d’ orbitals form the conduction band (Fig. 1b). Conduction bands also reveal the presence of contributions from O ‘p’ orbitals. As Ba atoms have ionic nature, we do not see their contributions in the pDOS plot.16
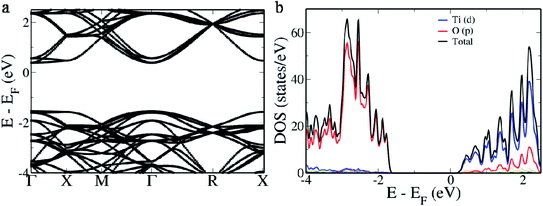 |
| Fig. 1 (a) Electronic structure and (b) pDOS of the 2 × 2 × 2 supercell of BaTiO3. | |
Since there is a possibility of Rh atoms occupying both the sites simultaneously (case A) or either Ti sites (case B) or Ba sites (case C) only, we simulated the electronic structure for all three conditions. When Rh atoms occupy one Ba site and one Ti site (case A), we see that the electronic structure reveals the occurrence of an acceptor level within the band gap with a width of 0.167 eV above the Fermi level, effectively decreasing the material band gap to 0.673 eV (Fig. 2a). The lifetime of the photogenerated electrons and holes is largely affected due to the presence of this acceptor level as it can act as a recombination center. On careful observation of pDOS, we find that the acceptor level is formed due to the hybridization of ‘d’ orbitals of Rh atoms in Ba sites and ‘p’ orbitals of O atoms (Fig. 2b). However, the valence band edge is formed out of contribution from ‘d’ orbitals of both the Rh atoms along with ‘p’ orbitals of the O atoms. We see a large gap of 1.032 eV between the edge of the valence band and the acceptor level which effectively prevents the replenishment of electrons leading to a larger lifetime of the recombination center.34
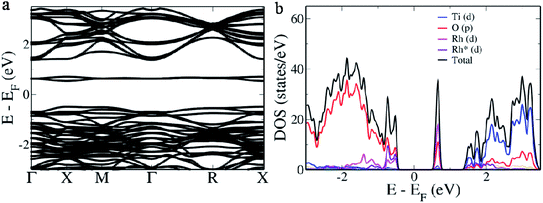 |
| Fig. 2 (a) Electronic structure and (b) pDOS of the 2 × 2 × 2 supercell of Rh-doped BaTiO3 (case A) with one Rh in the Ti site (lavender) indicated as Rh* and another Rh in the Ba site (pink). | |
We further visualized the wavefunctions at the Γ point at various energy levels marked in the pDOS plot given in Fig. S1.† Hyper deep defect states appear at region I due to the ‘d’ states of Rh atoms (Fig. 3a).41 While we observe the contributions of ‘p’ orbitals of O in the valence band regions II and III, contributions of ‘d’ orbitals of both the Rh atoms were also dominant at the valence band edge (Fig. 3b and c). In region IV we clearly see the contribution due to ‘d’ orbitals of Rh in the Ba site while minor contribution from ‘d’ orbitals of Rh in the Ti site is seen at the conduction band edge in region V along with major contributions from ‘d’ orbitals of Ti (Fig. 3d and e). Upper conduction bands in region VI show major contributions from ‘d’ orbitals of Ti and ‘p’ orbitals of O atoms (Fig. 3f).
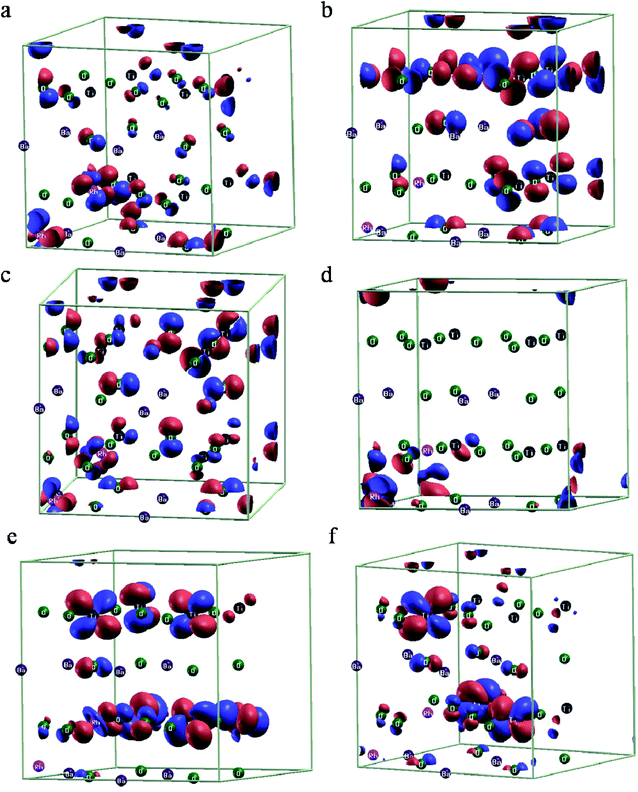 |
| Fig. 3 Wavefunction visualization at the Γ point (a–f) corresponding to various energy levels as indicated by Roman numerals I–VI in the pDOS in Fig. S1,† respectively, for case A. Red and blue colors indicate the positive and negative lobes of the orbitals. | |
In case B, we estimate a direct band gap of 2.028 eV at the Γ point and an indirect band gap of 1.796 eV from X → G. The indirect band gap is a consequence of overlapping of the defect band with the valence band edge (Fig. 4a). pDOS reveals this area of overlap as hybridization of ‘p’ orbitals of O with ‘d’ orbitals of Rh while rest of the contributions remain the same as that of the pristine BaTiO3 (Fig. 4b). In case C, we observe in the electronic structure, a separate donor level having a width of 0.363 eV formed 0.148 eV above the valence band edge (Fig. 5a). This effectively reduces the band gap to 1.525 eV making it the lowest value in all three cases. The pDOS reveals that while the valence band is formed out of major contribution of ‘p’ orbitals of O, it also hybridizes with ‘d’ orbitals of Rh to form the donor level and shows minor contributions in the conduction band which has major contributions from ‘d’ orbitals of the Ti atom (Fig. 5b). For easy migration of photogenerated charge carriers, continuous nature of the band is essential which is seen in the case of single occupancy.34
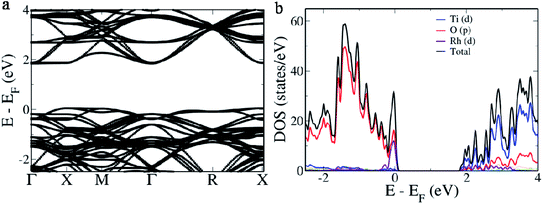 |
| Fig. 4 (a) Electronic structure and (b) pDOS of the 2 × 2 × 2 supercell of Rh-doped BaTiO3 (case B) with Rh in the Ti site. | |
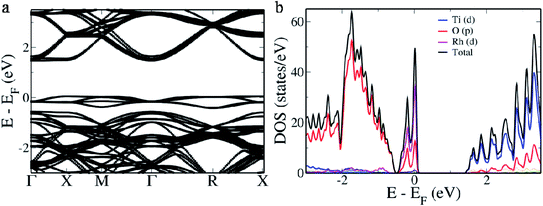 |
| Fig. 5 (a) Electronic structure and (b) of the 2 × 2 × 2 supercell of Rh-doped BaTiO3 (case C) with Rh in the Ba site. | |
Further, we increased the concentration of Rh in BaTiO3 by substituting two Rh in Ba sites. In the electronic structure, we see that with the increase in the concentration of Rh the width of the donor level increases to 0.472 eV. Also, it revealed a further decrease in the band gap to 1.461 eV predicting better absorption of visible light and hence higher photocatalytic efficiency consistent with the experiments (Fig. S2†). It is seen that when Rh occupies the Ba site, the band gap decreases to a maximum extent indicating the extension of light absorption to the visible region. Also, since the donor level is only slightly above the valence band within the Fermi level, rapid replenishment of electrons would occur avoiding the formation of trap centers, thus increasing the carrier life time.34,42 The results from the DFT calculations reveal that a synthetic method to direct Rh towards the Ba site would help in developing a better photocatalyst than Rh in the Ti site. Furthermore, if Rh occupies both the sites then the material would not be an ideal photocatalyst. Hence, we adopted a hydrothermal approach to direct Rh towards the Ba site during the synthesis so that a photocatalyst with reduced band gap and donor levels is formed without creation of the recombination centers.
XRD analysis
We observe that the XRD pattern of the synthesized samples matched well with the cubic phase of BaTiO3 (JCPDS card no. 00-031-0174) indicating that both doped and undoped samples had the same crystal structure (Fig. 6). We were not able to see any impurity peaks, which indicated the high purity of the synthesized samples. The ionic radii of Rh3+, Ba2+ and Ti4+ are 0.067 nm, 0.161 nm and 0.061 nm, respectively, which means that Rh should preferably be doped in the Ti site. Substitution in the Ti site would have caused a slight increase in the lattice parameter which was not the case here. The synthetic approach we adopted directs the Rh towards the Ba site which leads to a reduction in the lattice parameter to 4.02 Å. This manifests as shifting of peaks towards higher θ values in the doped samples with respect to the undoped BaTiO3 (Fig. S3†).
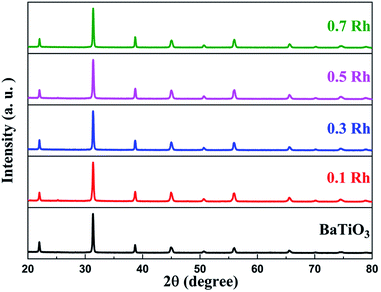 |
| Fig. 6 XRD patterns of BaTiO3 and Rh-doped BaTiO3 with varying concentrations of Rh. | |
XPS analysis
The XPS survey spectrum indicated the presence of Ba, Ti, O and Rh species in the sample (Fig. S4†). We observe that Ba 3d has two spin states Ba 3d5/2 and Ba 3d3/2 which appear at binding energies of 778.74 eV and 794.03 eV, respectively, as peaks (Fig. 7a). The binding energy separation of 15.29 eV between the two spin states of Ba confirmed the oxidation state of Ba in BaTiO3 as +2.7,43 The high-resolution spectrum of Ti indicates that it is in the +4 oxidation state as peaks emerge at binding energies of 458.42 eV (for Ti 2p3/2) and 464.19 eV (for Ti 2p1/2), respectively (Fig. 7b).3 Further, the peaks at binding energies 529.61 eV and 531.45 eV can be attributed to the lattice oxygen (OL) and surface hydroxyl groups (OOH), respectively (Fig. 7c).44,45 The deconvoluted peaks of Rh at binding energies 310.10 eV and 314.58 eV can be allotted to the Rh 3d5/2 and Rh 3d3/2 spin states, respectively (Fig. 7d). These binding energy values indicated the successful incorporation of Rh3+ ions into the lattice of BaTiO3 and not Rh4+.34,36
 |
| Fig. 7 High-resolution XPS plot of (a) Ba 3d, (b) Ti 3p, (c) O 1s and (d) Rh 3d. | |
Morphology and surface area analysis
We studied the morphology of the samples using TEM. We observe that undoped BaTiO3 has a cubooctahedral shape, while doping it with Rh makes it cubic in structure (Fig. 8a and b). We observe the 110 planes in the HRTEM image of the 0.5 Rh sample with a spacing of 0.28 nm (Fig. 8c). The single crystalline nature of the synthesized 0.5 Rh sample indicates the success of the employed synthetic technique. The elemental composition studied using EDX reveals Ba, Ti, O and Rh as the elements present, confirming the results from XPS (Fig. 8d). The spot EDX confirms the uniform distribution of the atoms in the sample (Fig. S5†).
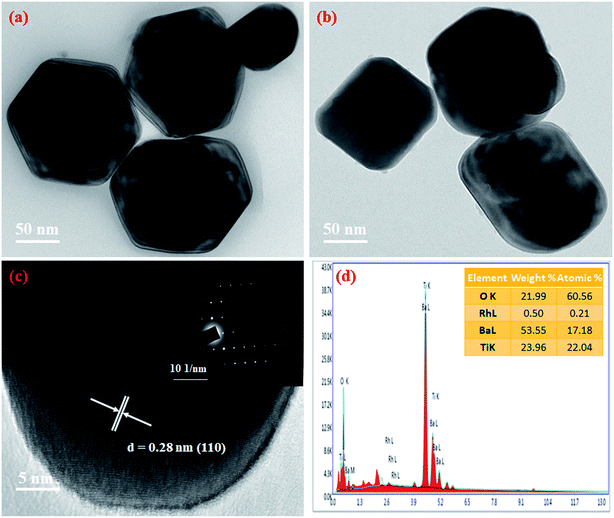 |
| Fig. 8 (a) TEM image of BaTiO3, (b) TEM image of 0.5 Rh, (c) HRTEM of 0.5 Rh (inset shows the SAED pattern of the 0.5 Rh sample) and (d) EDX spectrum of 0.5 Rh samples. | |
Since the surface area of the sample plays a huge role in the catalysis process, we studied the surface area of both doped and undoped BaTiO3. In the nitrogen adsorption–desorption isotherms of BaTiO3 and 0.5 Rh samples we observe a type IV pattern with hysteresis loops being type H3, indicating the presence of slit like pores (Fig. S6 and S9†).46 The BET surface area was found to be 7 m2 g−1 for the 0.5 Rh sample, which is higher than that of pure BaTiO3 (4.5 m2 g−1). This higher surface area of the 0.5 Rh sample will be highly beneficial for the effective adsorption and degradation of the pollutants. A broad BJH pore size distribution in the range of 2–100 nm indicated the presence of mesopores and macropores (inset of Fig. 9).47 The pore volume of the 0.5 Rh sample was found to be 0.0419 cm3 g−1 which is almost twice that of the pristine sample (0.0229 cm3 g−1), which helps in the efficient photo-absorption and diffusion of molecules during the photocatalytic reaction.
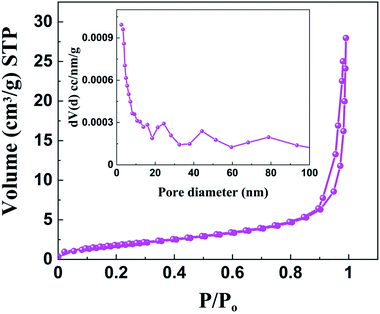 |
| Fig. 9 BET surface area analysis of the 0.5 Rh sample (inset shows the pore size distribution). | |
Optical absorbance analysis
In the DR spectra of pristine BaTiO3 we observe an absorption edge at around 400 nm due to the electronic transitions from the O ‘p’ orbitals to the Ti ‘d’ orbitals (Fig. 10a). However, the absorption edges of Rh-doped samples are red shifted to the visible region (400–550 nm) due to the decrease in the band gap. These wavelengths can be attributed to the electronic transitions from the donor level formed by the Rh3+ species to the conduction band of BaTiO3. The characteristic absorption peak at 580 nm, caused by Rh4+, was absent indicating that Rh was doped in the +3 oxidation state further confirming the results from XPS.34,48
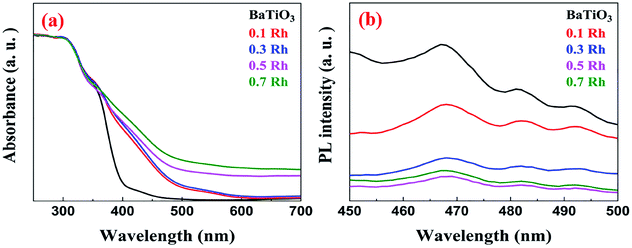 |
| Fig. 10 (a) DR and (b) PL spectra of BaTiO3 and Rh-doped BaTiO3 samples. | |
The direct band gap of the samples was calculated using the Tauc relation using eqn (2).
Where
α is the absorption coefficient,
Eg is the band gap energy of the semiconductor,
K is a constant, and
hν is the photon energy. The band gap energies of BaTiO
3, 0.1 Rh, 0.3 Rh, 0.5 Rh and 0.7 Rh samples estimated from their respective absorption edge values were found to be 3.04 eV, 2.37 eV, 2.34 eV, 2.26 eV and 2.17 eV, respectively. We observe a red shift in the absorption peak accompanied by a decrease in the band gap with an increase in the concentration of Rh doping due to the increase in the width of the donor level which further gets closer to the conduction band.
In the PL spectra of BaTiO3 and Rh-doped BaTiO3, we can observe that there is a noticeable drop in the fluorescence intensity for the doped samples compared to that of the pristine sample, which indicates the lower opportunity for the recombination of photoinduced charges in the case of doped samples (Fig. 10b).49 The electrons are rapidly replenished to the vacant site by the valence band as discussed in the DFT results which prevents their recombination with the holes generated. Thus, more photoinduced holes and electrons can participate in the photocatalytic reactions. It can be noted that the PL intensity decreases till 0.5 Rh and beyond this concentration the PL intensity increases which can be attributed to the formation of recombination centers probably due to mixed occupancies as revealed by DFT studies.
Photocatalytic activity
The photocatalytic efficiency of the synthesized materials was evaluated for the decomposition of MB dye under irradiation of visible light. Fig. 11a shows the photocatalytic degradation curves of MB in the presence of BaTiO3 and Rh-doped BaTiO3 samples. The percentage degradation of MB by BaTiO3, 0.1 Rh, 0.3 Rh, 0.5 Rh and 0.7 Rh samples was found to be 45, 55, 69, 96 and 73, respectively. We observed that the photocatalytic activity of Rh-doped samples was higher than that of the pristine sample, which could be attributed to the formation of Rh3+ donor levels above the valence band which efficiently decreased the band gap enabling harvesting of the visible light. The 0.5 Rh sample exhibited the highest photocatalytic activity beyond which the activity decreased. This is due to the fact that even though the doping of Rh decreased the band gap, at higher concentration there is larger scope for mixed occupancy leading to the formation of recombination centers as revealed by the DFT calculations. This fact is also supported by the PL studies above. To further confirm this fact, we synthesized the 0.9 Rh sample and tested its photocatalytic activity. The characterization results and the photocatalytic activity are provided in Fig. S7.† We observe that the 0.9 Rh sample shows the least activity of 57% with a rate constant of 0.0068 min−1.
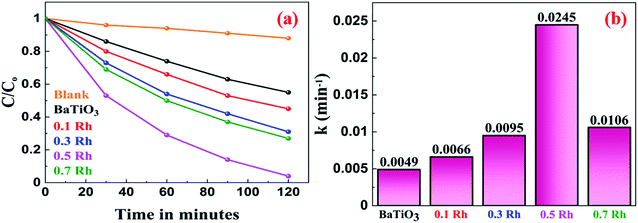 |
| Fig. 11 (a) The photocatalytic degradation of MB and (b) the rate constants of the photocatalytic degradation of MB by the synthesized BaTiO3 and Rh-doped BaTiO3. | |
The kinetics of the photocatalytic degradation of MB by BaTiO3 and Rh-doped BaTiO3 samples are well in agreement with the pseudo first-order rate equation given by eqn (3).
where
Co is the initial concentration of the dye,
C is the concentration of the dye at time ‘
t’ and
k is the first order rate constant, which was calculated from the slope of the straight line obtained by plotting −ln(
C/
Co)
versus ‘
t’.
49 The rate constant of the 0.5 Rh sample was found to be higher than that of all the synthesized samples (
Fig. 11b). Further, the 0.5 Rh sample showed only a marginal drop in the photocatalytic efficiency even after five consecutive cycles, suggesting its high stability and reusability towards photocatalytic degradation reactions (Fig. S8
†). Comparison of the photocatalytic activity of this material with that of the previously reported ones shows that the 0.5 Rh sample is highly efficient (Table S1
†).
The thermodynamic parameters such as energy of activation (Ea), free energy of activation (ΔG#), enthalpy of activation (ΔH#) and entropy of activation (ΔS#) were computed by employing activation complex theory (ACT) and Eyring equation as described in the previous reports.12 We see from Table 1 that the photodegradation of MB without a catalyst requires higher energy of activation while by using BaTiO3 and Rh-doped BaTiO3 catalysts, relatively lower energy of activation is needed, with the 0.5 Rh sample having the least value. This indicates that addition of catalysts alters the reaction pathway, which confirms that the catalyst alters the path of the reaction by lowering the activation energy. Positive enthalpy and free energy change indicate that the reaction is endothermic and not spontaneous.
Table 1 Thermodynamic parameters of BaTiO3 and Rh-doped BaTiO3 samples
Sample |
E
a (kJ mol−1) |
ΔH# (kJ mol−1) |
ΔS# (kJ mol−1) |
ΔG# (kJ mol−1) |
Without catalyst |
17.7 |
15.2 |
−0.25 |
91.2 |
BaTiO3 |
13.2 |
10.7 |
−0.25 |
86.6 |
0.1 Rh |
12.5 |
10.0 |
−0.25 |
85.9 |
0.3 Rh |
11.6 |
9.1 |
−0.25 |
85.0 |
0.5 Rh |
9.3 |
6.8 |
−0.25 |
82.7 |
0.7 Rh |
11.3 |
8.8 |
−0.25 |
84.7 |
0.9 Rh |
12.4 |
9.9 |
−0.25 |
85.8 |
We also carried out trapping experiments in order to assess the active species involved in the photocatalytic degradation by following the same procedure as that of photocatalytic activity evaluation but with the aid of different radical scavenging agents such as benzoquinone (1 mM) as a superoxide anion radical
scavenger, potassium iodide (10 mM) as a hole (h+) scavenger and isopropyl alcohol (10 mM) as a hydroxyl radical (OH˙) scavenger. In Fig. 12 we observe that superoxide radical anions are not the major active species as the corresponding scavenger benzoquinone did not deteriorate the photocatalytic activity to a larger extent. However, the addition of potassium iodide and isopropyl alcohol reduced the photocatalytic activity significantly. As a result, holes followed by hydroxyl radicals are considered to be the active species for the effectual degradation of the dye.
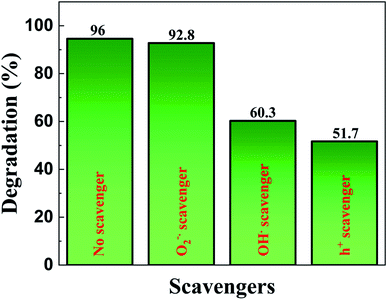 |
| Fig. 12 Effect of radical scavengers on the photocatalytic degradation of MB by the 0.5 Rh sample. | |
Based on the above study we propose a mechanism for the photocatalytic degradation of MB dye as follows: when the 0.5 Rh sample gets irradiated with an energy greater than or equal to its band gap, the electrons from the donor levels formed by Rh3+ species are transferred to the conduction band. Later, these electrons react with oxygen to produce superoxide anion radicals. The formed holes either directly react with MB to produce degradation products or react with surface hydroxyl groups to produce hydroxyl radicals. The formed superoxide and hydroxyl radicals react with dye molecules to produce harmless degradation products (Fig. 13).
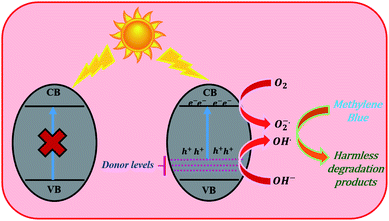 |
| Fig. 13 The photocatalytic mechanism of Rh-doped BaTiO3 for the degradation of MB under visible light irradiation. | |
Conclusions
Herein, we report a facile synthesis of Rh-doped BaTiO3 and its study as a photocatalyst in the degradation of MB. The experimental approach was designed by making use of first principles DFT calculations. The theoretical study revealed that by directing Rh towards either the Ba site or Ti site, only donor levels would be formed leading to a decreased band gap while simultaneous occupation of Ba and Ti sites would lead to the formation of acceptor states detrimental to the photocatalytic activity. Based on the above, we adopted a hydrothermal approach to direct Rh towards the Ba site. This led to the decrease in the band gap and extension of absorption to the visible region of the spectrum. The absence of recombination centers in 0.5 Rh samples led to increased photocatalytic activity with 96% MB degradation in 2 h revealing the approach to be successful even at low concentrations of Rh. This approach can very well be extended to other perovskite oxides to improve their efficiency in photocatalytic degradation of dyes.
Conflicts of interest
There are no conflicts to declare.
References
- M. M. J. Sadiq, U. S. Shenoy and D. K. Bhat, NiWO4-ZnO-NRGO Ternary Nanocomposite as an Efficient Photocatalyst for Degradation of Methylene Blue and Reduction of 4-Nitro Phenol, J. Phys. Chem. Solids, 2017, 109, 124–133 CrossRef CAS.
- S. Kampouri and K. C. Stylianou, Dual-Functional Photocatalysis for Simultaneous Hydrogen Production and Oxidation of Organic Substances, ACS Catal., 2019, 9, 4247–4270 CrossRef CAS.
- H. Bantawal, M. Sethi, U. S. Shenoy and D. K. Bhat, Porous Graphene Wrapped SrTiO3 Nanocomposite: Sr–C Bond as an Effective Coadjutant for High Performance Photocatalytic Degradation of Methylene Blue, ACS Appl. Nano Mater., 2019, 2, 6629–6636 CrossRef CAS.
- D. Zhong, W. Liu, P. Tan, A. Zhu, L. Qiao, Y. Bian and J. Pan, Efficient Hydrogen Generation of Indium Doped BaTiO3 Decorated with CdSe Quantum Dots: Novel Understanding of the Effect of Doping Strategy, Int. J. Hydrogen Energy, 2019, 44, 1627–1639 CrossRef CAS.
- A. Ali, Y. Liang, S. Ahmed, B. Yang, B. Guo and Y. Yang, Mutual Contaminants Relational Realization and Photocatalytic Treatment Using Cu2MgSnS4 Decorated BaTiO3, Applied Materials Today, 2020, 18, 100534 CrossRef.
- J. P. Zou, L. Z. Zhang, S. L. Luo, L. H. Leng, X. B. Luo, M. J. Zhang, Y. Luo and G. C. Guo, Preparation and Photocatalytic Activities of Two New Zn-Doped SrTiO3 and BaTiO3 Photocatalysts for Hydrogen Production from Water without Cocatalysts Loading, Int. J. Hydrogen Energy, 2012, 37, 17068–17077 CrossRef CAS.
- M. M. J. Sadiq, U. S. Shenoy and D. K. Bhat, Synthesis of BaWO4/NRGO–gC3N4 Nanocomposites with Excellent Multifunctional Catalytic Performance via Microwave Approach, Frontiers of Materials Science, 2018, 12, 247–263 CrossRef.
- M. M. J. Sadiq, U. S. Shenoy and D. K. Bhat, Enhanced Photocatalytic Performance of N-Doped RGO-FeWO4/Fe3O4 Ternary Nanocomposite in Environmental Applications. Mater, Today Chem., 2017, 4, 133–141 Search PubMed.
- X. Huang, G. Zhao, G. Wang and J. T. Irvine, Synthesis and Applications of Nanoporous Perovskite Metal Oxides, Chem. Sci., 2018, 9, 3623–3637 RSC.
- S. Xu, L. Guo, Q. Sun and Z. L. Wang, Piezotronic Effect Enhanced Plasmonic Photocatalysis by AuNPs/BaTiO3 Heterostructures, Adv. Funct. Mater., 2019, 29, 1808737 CrossRef.
- H. Bantawal, U. S. Shenoy and D. K. Bhat, Tuning the Photocatalytic Activity of SrTiO3 by Varying the Sr/Ti Ratio: Unusual Effect of Viscosity of the Synthesis Medium, J. Phys. Chem. C, 2018, 122, 20027–20033 CrossRef CAS.
- H. Bantawal, U. S. Shenoy and D. K. Bhat, Vanadium-Doped SrTiO3 Nanocubes: Insight into Role of Vanadium in Improving the Photocatalytic Activity, Appl. Surf. Sci., 2020, 513, 145858 CrossRef CAS.
- M. Nageri and V. Kumar, Manganese-Doped BaTiO3 Nanotube Arrays for Enhanced Visible Light Photocatalytic Applications, Mater. Chem. Phys., 2018, 213, 400–405 CrossRef CAS.
- Z. Mengting, T. A. Kurniawan, S. Fei, T. Ouyang, M. H. D. Othman, M. Rezakazemi and S. Shirazian, Applicability of BaTiO3/Graphene Oxide (GO) Composite for Enhanced Photodegradation of Methylene Blue (MB) in Synthetic Wastewater under UV–Vis Irradiation, Environ. Pollut., 2019, 255, 113182 CrossRef.
- R. X. Wang, Q. Zhu, W. S. Wang, C. M. Fan and A. W. Xu, BaTiO3–Graphene Nanocomposites: Synthesis and Visible Light Photocatalytic Activity, New J. Chem., 2015, 39, 4407–4413 RSC.
- U. S. Shenoy and D. K. Bhat, Vanadium Doped BaTiO3 as High Performance Thermoelectric Material: Role of Electronic Structure Engineering, Mater. Today Chem., 2020, 18, 100384 CrossRef.
- H. Xiao, C. Luo, G. Huangfu and Y. Guo, Boosting the Photocatalytic Ability of Bandgap Engineered (Na0.5Bi0.5)TiO3-BaTiO3 by N-Ni Codoping, J. Phys. Chem. C, 2020, 124, 11810–11818 CrossRef CAS.
- M.
J. S. Mohamed, S. Shenoy and D. K. Bhat, Novel NRGO-CoWO4-Fe2O3 Nanocomposite as an Efficient Catalyst for Dye Degradation and Reduction of 4-Nitrophenol, Mater. Chem. Phys., 2018, 208, 112–122 CrossRef CAS.
- D. Liu, C. Jin, F. Shan, J. He and F. Wang, Synthesizing BaTiO3 Nanostructures to Explore Morphological Influence, Kinetics, and Mechanism of Piezocatalytic Dye Degradation, ACS Appl. Mater. Interfaces, 2020, 12, 17443–17451 CrossRef CAS.
- X. Liu, L. Xiao, Y. Zhang and H. Sun, Significantly Enhanced Piezo-Photocatalytic Capability in BaTiO3 Nanowires for Degrading Organic Dye, Journal of Materiomics, 2020, 6, 256–262 CrossRef.
- S. Xu, Z. Liu, M. Zhang and L. Guo, Piezotronics Enhanced Photocatalytic Activities of Ag-BaTiO3 Plasmonic Photocatalysts, J. Alloys Compd., 2019, 801, 483–488 CrossRef CAS.
- T. Xian, H. Yang, L. J. Di and J. F. Dai, Enhanced Photocatalytic Activity of BaTiO3@ g-C3N4 for the Degradation of Methyl Orange under Simulated Sunlight Irradiation, J. Alloys Compd., 2015, 622, 1098–1104 CrossRef CAS.
- P. Demircivi, B. Gulen, E. B. Simsek and D. Berek, Enhanced Photocatalytic Degradation of Tetracycline using Hydrothermally Synthesized Carbon Fiber Decorated BaTiO3, Mater. Chem. Phys., 2020, 241, 122236 CrossRef CAS.
- P. Senthilkumar, D. A. Jency, T. Kavinkumar, D. Dhayanithi, S. Dhanuskodi, M. Umadevi, S. Manivannan, N. V. Giridharan, V. Thiagarajan, M. Sriramkumar and K. Jothivenkatachalam, Built-in Electric Field Assisted Photocatalytic Dye Degradation and Photoelectrochemical Water Splitting of Ferroelectric Ce Doped BaTiO3 Nanoassemblies, ACS Sustainable Chem. Eng., 2019, 7, 12032–12043 CAS.
- I. C. Amaechi, G. Kolhatkar, A. H. Youssef, D. Rawach, S. Sun and A. Ruediger, B-Site Modified Photoferroic Cr3+-Doped Barium Titanate Nanoparticles: Microwave-Assisted Hydrothermal Synthesis, Photocatalytic and Electrochemical Properties, RSC Adv., 2019, 9, 20806–20817 RSC.
- I. C. Amaechi, A. H. Youssef, G. Kolhatkar, D. Rawach, C. Gomez-Yañez, J. P. Claverie, S. Sun and A. Ruediger, Ultrafast Microwave-Assisted Hydrothermal Synthesis and Photocatalytic Behaviour of Ferroelectric Fe3+-Doped BaTiO3 Nanoparticles under Simulated Sunlight, Catal. Today, 2019 DOI:10.1016/j.cattod.2019.07.021.
- P. Xie, F. Yang, R. Li, C. Ai, C. Lin and S. Lin, Improving Hydrogen Evolution Activity of Perovskite BaTiO3 with Mo Doping: Experiments and First-Principles Analysis, Int. J. Hydrogen Energy, 2019, 44, 11695–11704 CrossRef CAS.
- J. Cao, Y. Ji, C. Tian and Z. Yi, Synthesis and Enhancement of Visible Light Activities of Nitrogen-Doped BaTiO3, J. Alloys Compd., 2014, 615, 243–248 CrossRef CAS.
- P. Demircivi and E. B. Simsek, Visible-Light-Enhanced Photoactivity of Perovskite-Type W-Doped BaTiO3 Photocatalyst for Photodegradation of Tetracycline, J. Alloys Compd., 2019, 774, 795–802 CrossRef CAS.
- H. C. Huang, C. L. Yang, M. S. Wang and X. G. Ma, Chalcogens Doped BaTiO3 for Visible Light Photocatalytic Hydrogen Production from Water Splitting, Spectrochim. Acta, Part A, 2019, 208, 65–72 CrossRef CAS.
- S. Shenoy and D. K. Bhat, Enhanced Bulk Thermoelectric Performance of Pb0.6Sn0.4Te: Effect of Magnesium Doping, J. Phys. Chem. C, 2017, 121, 20696–20703 CrossRef CAS.
- U. S. Shenoy and D. K. Bhat, Enhanced Thermoelectric Properties of Vanadium Doped SrTiO3: A Resonant Dopant Approach, J. Alloys Compd., 2020, 832, 154958 CrossRef CAS.
- T. Hisatomi and K. Domen, Reaction Systems for Solar Hydrogen Production via Water Splitting with Particulate Semiconductor Photocatalysts, Nat. Catal., 2019, 2, 387–399 CrossRef CAS.
- U. S. Shenoy, H. Bantawal and D. K. Bhat, Band Engineering of SrTiO3: Effect of Synthetic Technique and Site Occupancy of Doped Rhodium, J. Phys. Chem. C, 2018, 122, 27567–27574 CrossRef CAS.
- U. S. Shenoy and D. K. Bhat, Electronic Structure Engineering of SrTiO3 via Rhodium doping: A DFT Study, J. Phys. Chem. Solids, 2021, 148, 109708 CrossRef CAS.
- K. Maeda, Rhodium-Doped Barium Titanate Perovskite as a Stable p-Type Semiconductor Photocatalyst for Hydrogen Evolution under Visible Light, ACS Appl. Mater. Interfaces, 2014, 6, 2167–2173 CrossRef CAS.
- S. Nishioka and K. Maeda, Hydrothermal Synthesis of Rhodium-Doped Barium Titanate Nanocrystals for Enhanced Photocatalytic Hydrogen Evolution under Visible Light, RSC Adv., 2015, 5, 100123–100128 RSC.
- P. Giannozzi, S. Baroni, N. Bonini, M. Calandra, R. Car, C. Cavazzoni, D. Ceresoli, G. L. Chiarotti, M. Cococcioni, I. Dabo, A. L. Corso, S. de Gironcoli, S. Fabris, G. Fratesi, R. Gebauer, U. Gerstmann, C. Gougoussis, A. Kokalj, M. Lazzeri, L. Martin-Samos, N. Marzari, F. Mauri, R. Mazzarello, S. Paolini, A. Pasquarello, L. Paulatto, C. Sbraccia, S. Scandolo, G. Sclauzero, A. P. Seitsonen, A. Smogunov, P. Umari and R. M. Wentzcovitch, Quantum ESPRESSO: A Modular and Open-Source Software Project for Quantum Simulations of Materials, J. Phys.: Condens. Matter, 2009, 21, 395502 CrossRef.
- J. P. Perdew, K. Burke and M. Ernzerhof, Generalized Gradient Approximation Made Simple, Phys. Rev. Lett., 1996, 77, 3865–3868 CrossRef CAS.
- D. K. Bhat and U. S. Shenoy, Zn: A Versatile Resonant Dopant for SnTe Thermoelectrics, Materials Today Physics, 2019, 11, 100158 CrossRef.
- D. K. Bhat and S. U. Shenoy, Resonance Levels in GeTe Thermoelectrics: Zinc as a New Multifaceted Dopant, New J. Chem., 2020, 44, 17664–17670 RSC.
- H. C. Chen, C. W. Huang, J. C. Wu and S. T. Lin, Theoretical Investigation of the Metal-Doped SrTiO3 Photocatalysts for Water Splitting, J. Phys. Chem. C, 2012, 116, 7897–7903 CrossRef CAS.
- M. J. S. Mohamed and D. K. Bhat, A Facile Microwave Approach to Synthesize RGO-BaWO4 Composites for High Performance Visible Light Induced Photocatalytic Degradation of Dyes, AIMS Mater. Sci., 2017, 4, 487–502 Search PubMed.
- M. Sethi, S. U. Shenoy and D. K. Bhat, Porous Graphene-NiCo2O4 Nanorod Hybrid Composite as High Performance Supercapacitor Electrode Material, New J. Chem., 2020, 44, 4033–4041 RSC.
- I. C. Amaechi, A. Hadj Youssef, D. Rawach, J. P. Claverie, S. Sun and A. Ruediger, Ferroelectric Fe–Cr Codoped BaTiO3 Nanoparticles for the Photocatalytic Oxidation of Azo Dyes, ACS Appl. Nano Mater., 2019, 2, 2890–2901 CrossRef CAS.
- M. Sethi, S. U. Shenoy and D. K. Bhat, Porous graphene - NiFe2O4 Nanocomposite with High Electrochemical Performance and High Cyclic Stability for Energy Storage Application, Nanoscale Adv., 2020, 2, 4229–4241 RSC.
- M. Sethi, H. Bantawal, S. U. Shenoy and D. K. Bhat, Eco-friendly Synthesis of Porous Graphene and Its Utilization as High Performance Supercapacitor Electrode Material, J. Alloys Compd., 2019, 799, 256–266 CrossRef CAS.
- S. Kawasaki, K. Akagi, K. Nakatsuji, S. Yamamoto, I. Matsuda, Y. Harada, J. Yoshinobu, F. Komori, R. Takahashi, M. Lippmaa and C. Sakai, Elucidation of Rh-Induced In-Gap States of Rh: SrTiO3 Visible-Light-Driven Photocatalyst by Soft X-ray Spectroscopy and First-Principles Calculations, J. Phys. Chem. C, 2012, 116, 24445–24448 CrossRef CAS.
- M. M. J. Sadiq, U. S. Shenoy and D. K. Bhat, Novel RGO–ZnWO4–Fe3O4 Nanocomposite as High Performance Visible Light Photocatalyst, RSC Adv., 2016, 6, 61821–61829 RSC.
Footnote |
† Electronic supplementary information (ESI) available. See DOI: 10.1039/d0na00702a |
|
This journal is © The Royal Society of Chemistry 2020 |
Click here to see how this site uses Cookies. View our privacy policy here.