DOI:
10.1039/D0NA00749H
(Paper)
Nanoscale Adv., 2020,
2, 5682-5687
Gas–solid two-phase flow (GSF) mechanochemical synthesis of dual-metal–organic frameworks and research on electrochemical properties†
Received
6th September 2020
, Accepted 19th October 2020
First published on 20th October 2020
Abstract
As an alternative approach for conventional mechanochemical synthesis, a novel gas–solid two-phase flow (GSF) synthetic technique for the mechanochemical synthesis of dual metal–organic frameworks (DMOFs) was reported for the first time. The prepared CoMn2(BTC)2 was characterized by FT-IR, DTA, TG/DTG, and XRD studies. The results indicated that CoMn2(BTC)2 (BTC = 1,3,5-benzenetricarboxylate) was successfully synthesized after 10 min at a rate of 60 kg h−1. CoMn2O4 microspheres were also prepared via the CoMn2(BTC)2 precursor method and characterized using FT-IR, XPS, XRD, SEM, EDS, and BET methods. The electrochemical properties of the as-prepared CoMn2O4 were investigated, and the GSF results showed that the microsphere electrodes of CoMn2O4 had a high specific capacitance (969 F g−1) at a current density of 1 A g−1 in 3 M aqueous KOH solution.
Introduction
Bimetallic oxides have been extensively studied and are considered a candidate for lithium-ion battery (LIB) anode materials because of their higher theoretical specific capacity than graphite, low cost, and environmental benignity. In particular, CoMn2O4 has the advantages of cobalt- and manganese-based metal oxides and superior application in the field of LIBs.1,2
The precursor method was one of the main methods to prepare CoMn2O4. Usually, sodium hydroxide or acid citrate is used to provide coordination sites, and cobalt and manganese are added to form a complex at high temperature and high pressure by the solvothermal method. Then, the obtained complex is burned to prepare the target product. However, this traditional method requires the extensive use of hostile organic solvents, thereby increasing environmental burden. Moreover, it is impossible to achieve the process conditions of batch preparation.
In this study, we used dual metal–organic frameworks (DMOFs) [CoMn2(BTC)2] as the precursor of CoMn2O4.3–12 Before mechanochemical synthesis,13–17 DMOFs were also prepared through the conventional solvohermal method. Nowadays, MOFs are realized by mechanical ball milling and twin-screw extrusion. However, these synthetic techniques suffer from many drawbacks, such as time and energy consumption.18–20 Thus, the development of a novel mechanochemical synthetic technique is highly desirable.
This work explored the use of a gas–solid two-phase flow (GSF) (Fig. 1) for the mechanochemical synthesis of DMOFs (Fig. S5† shows the reaction process flow chart of the GSF). The results indicated that CoMn2(BTC)2 (BTC = 1,3,5-benzenetricarboxylate) was successfully synthesized. CoMn2(BTC)2 was also used as a precursor to prepare CoMn2O4 microspheres, and their electrochemical properties were studied.
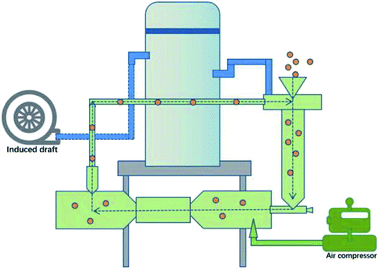 |
| Fig. 1 The reaction process flow chart of the GSF. | |
Experimental
Chemicals and materials
Cobaltous nitrate (Co(NO3)2·6H2O, AR, 98.0%) and manganous acetate (Mn(CH3COO)2, AR, 99%) were purchased from Kelong (Chengdu, China). 1,3,5-Trimesic acid (H3BTC), was purchased from Aladdin (Shanghai, China). Fourier transform infrared (FT-IR) spectra were recorded on a Nicolet-5700 FTIR spectrometer using pressed KBr pellets to test the chemical bonding of the samples from 4000 to 400 cm−1. X-ray photoelectron spectroscopy (XPS) experiments were carried out using an XPS-7000 spectrometer with Al Kα radiation. Thermogravimetric analysis (TGA) was carried out on a TGA 4000 (Perkin Elmer Co., Ltd) with the temperature range from room temperature to 600 °C in air. Differential thermal analysis (DTA) curves were recorded on a WCR-1B instrument in flowing air at a heating rate of 10 °C min−1. Field-emission scanning electron microscopy (FESEM) measurements were performed on an Ultra 55 microscope (ZEISS Company, The German) with an acceleration voltage of 15.0 kV with energy dispersive spectroscopy (EDS) detectors. XRD was performed on a Philips X'Pert Pro X-ray diffractometer (PANalytical, Holland). The N2 adsorption–desorption tests were performed on a Micromeritics ASAP 2460 instrument.
Synthesis of CoMn2(BTC)2
CoMn2(BTC)2 was prepared as follows. Co(NO3)2·6H2O (1.309 kg), Mn(CH3COO)2 (0.805 kg) and H3BTC (1.38 kg) were mixed, and the mixture was transferred into an impact chamber at the rate of 1 kg min−1 (60 kg h−1). Then, the materials were accelerated to supersonic velocity with compressed air (1.5 MPa).21 The products were collected after different reaction times. Here, 1.68 kg of the purple crystal were collected after 10 min. Yields: 91% based on H3BTC.
Precursor method for the synthesis of CoMn2O4 microspheres
CoMn2O4 was prepared as shown in Scheme 1. The as-prepared CoMn2(BTC)2 was placed in a tube furnace and heated to 600 °C at a temperature increase rate of 10 °C min−1 in air, and maintained at this temperature for 12 h, then cooled down to room temperature, and the CoMn2O4 microspheres were obtained.22–25
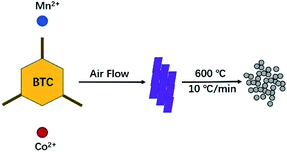 |
| Scheme 1 Preparation of CoMn2O4 with a controlled composition and size. | |
Results and discussion
Characterization
Fig. 2 shows the FT-IR spectrum of CoMn2O4. Similar to the results reported in the literature, the peaks at 3412 and 1628 cm−1 originated from a hydroxyl group in the form of physically adsorbed water.26–28 The two peaks at 611 and 495 cm−1 corresponded to the Mn–O and the Co–O bonds.
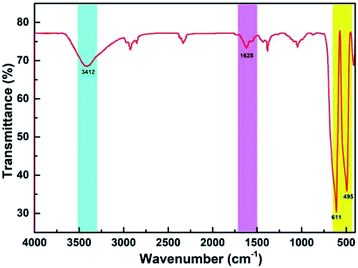 |
| Fig. 2 Fourier transform infrared (FT-IR) spectra of CoMn2O4. | |
Fig. 3a illustrates the XPS survey.29Fig. 3b shows that the O 1s peak was at 529.8 and 528.8 eV. A hybrid of the two peaks with Gaussian fitting was used to obtain the amount of points for calculation of the two peaks. The peaks at 529.8 and 528.8 eV represented the Mn–O–Mn and the Mn–OH bonds, respectively. Cobalt oxide was negligible because the quantity of the doped cobalt was low. The Mn–O–Mn and the Mn–OH bonds were related to MnO2 and Mn2O3, respectively. As such, the manganese oxide (Fig. 3c) in CoMn2O4 was mixed with MnO2 and Mn2O3 at 29.81% and 70.19%, respectively, on the basis of the peak separation results. The valence of manganese oxide was less than quadrivalent, and its oxidation state was 2.6. Fig. 3d shows the Co 2p1/2 and Co 2p3/2 peaks at 780.5 and 795.9 eV, which was consistent with the Co 2p orbital peak of Co3O4. Thus, Co, existing in the form of a Co3O4 spinel structure, can cause lattice distortion in the manganese oxide mixture and increase surface spacing, making it easier to conduct ions into the manganese oxide.30
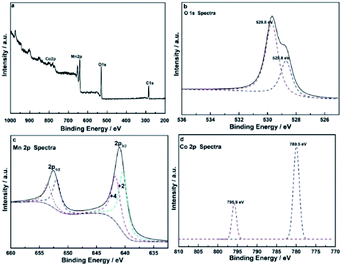 |
| Fig. 3 (a) High-resolution XPS spectra of (b) deconvoluted O 1s, (c) Co 2p, and (d) Mn 2p of CoMn2O4. | |
Fig. S6 and S7† show the XRD patterns of the product collected at different times and after TGA, respectively. As illustrated in Fig. 4, the XRD pattern can be readily assigned to the body-centered tetragonal CoMn2O4 (JCPDS card no. 77-0471), which showed good properties.31 The absence of the characterization peaks from residues or other contaminants indicated the high purity of the products.
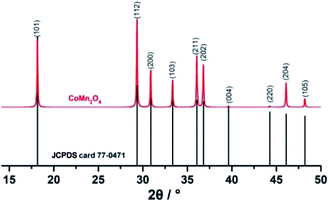 |
| Fig. 4 XRD pattern of CoMn2O4 and the standard diffraction pattern of CoMn2O4 (JCPDS card no. 77-0471). | |
The electrochemical performance of active materials is greatly related to the morphology and assembled structures. Consequently, the formation of nanosheet assembled microspheres is distinctly important to the electrochemical performance of CoMn2O4. The hybrids were investigated by field-emission scanning electron microscopy to obtain the microscopic structure of CoMn2O4, explore its application value, and further illustrate CoMn2O4 synthesis. Fig. 5a shows the typical SEM image of the obtained CoMn2O4 in the form of a regular microsphere. As shown in Fig. 5b, the microsphere showed plate-like particles when we further enlarged the surface of CoMn2O4. The surface of the CoMn2O4 microsphere was smooth and had many micropores. Meanwhile, the chemical analysis conducted via EDS revealed the compositional signals for Co, Mn, and O, as confirmed by the elemental mapping images (Fig. 5c–f).32
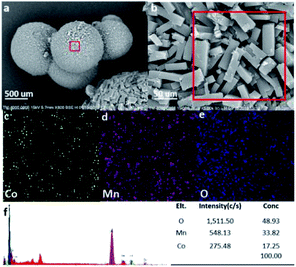 |
| Fig. 5 (a and b) SEM images of CoMn2O4, (c–e) elemental mapping images, and (f) corresponding elemental contents measured by energy dispersive spectroscopy. | |
Nitrogen adsorption/desorption isotherms were obtained at 77 K to study the textural properties of CoMn2O4. Because of its spherical structure, its specific surface area was large. Based on the nitrogen adsorption–desorption curves, the BET surface area of CoMn2O4 (ref. 33) (Fig. 6) was 63.3 m2 g−1 (Fig. S8† shows the BET surface area of CoMn2(BTC)2).
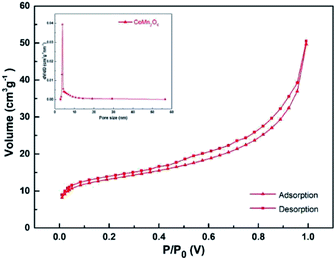 |
| Fig. 6 N2 adsorption/desorption isotherms of CoMn2O4. | |
Supercapacitive properties
The electrochemical properties of the as-carbonized materials were evaluated in the context of supercapacitors. For a three-electrode system in 3 M aqueous KOH solution, a Ag/AgCl electrode (0.22233 V) and Pt plates were used as reference and counter electrodes, respectively. A free-standing CoMn2O4 film with a thickness of about 0.2 mm served directly as the working electrode and was prepared by pressing the CoMn2O4 film (2.65 mg) onto a piece of copper foam (1 cm × 1 cm). Cyclic voltammetry (CV) and chronopotentiometry measurements were conducted at various scan rates (0.0–0.8 V) and current densities (1–20 A g−1), respectively. Benefiting from the porous microsphere structures, the CoMn2O4 electrodes were subjected to CV tests to evaluate their electrochemical behavior in 3 M aqueous KOH solution, as shown in Fig. 7.
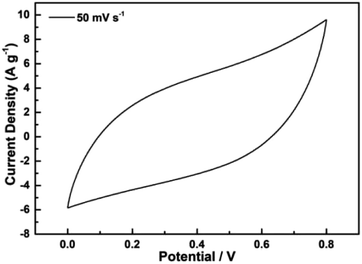 |
| Fig. 7 Cyclic voltammetry curve of CoMn2O4 electrodes obtained using the three-electrode method at a voltage scan rate of 50 mV s−1. | |
The CV curves were measured at scan rates between 5 and 100 mV s−1 within a potential window of 0.0–0.8 V (vs. Ag/AgCl), as shown in Fig. 8. Typical cathodic and anodic peaks were observed during the redox reaction between CoMn2O4 and MnO–CoO. The well-developed CV curve of CoMn2O4 exhibited the largest integral area, demonstrating that CoMn2O4 possessed the best supercapacitive behavior among the three electrodes. Meanwhile, two pairs of redox peaks were associated with the Faraday effect at 0.38 and 0.45 V and 0.08 and 0.58 V of the CV curve. The quasiregular rectangle shapes also confirmed the excellent supercapacitive nature of CoMn2O4.
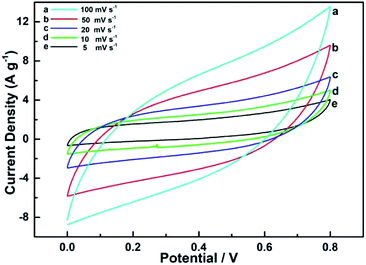 |
| Fig. 8 Cyclic voltammetry curve of supercapacitors with CoMn2O4 as the electrode at scan rates of (a) 100, (b) 50, (c) 20, (d) 10, and (e) 5 mV s−1. | |
The supercapacitive properties34 of CoMn2O4 were used for the subsequent method. Specific capacitance was calculated from various current densities by using the following equation:
|  | (1) |
where
I (A) represents the discharge current, Δ
t (s) refers to the discharge time within the potential change Δ
V (V), and
m is the active material weight in the working electrode. All electrochemical measurements were carried out using a CHI660C (Shanghai, China) electrochemical working station.
Fig. 9 shows the results of the constant current charge and discharge test of the CoMn2O4 supercapacitor in a voltage window of 1 V. The charge and discharge currents were 20, 5, 4, 2, and 1 A g−1. The supercapacitors with CoMn2O4 electrodes can be used as charge storage devices. When the current decreased, the specific capacity of CoMn2O4 ultracapacitors increased because the electrode process was limited by the diffusion and transfer rates of the charge and ion. The electrode material cannot be fully utilized, and its capacity was reduced when the diffusion and transfer rates of the charge and ion were not as fast as the rate of the charge flow. For comparison with different methods reported before, the results are listed in Table 1. A maximum specific capacitance of 969 F g−1 was obtained after charging and discharging at a current density of 1 A g−1.
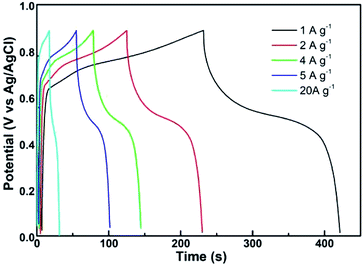 |
| Fig. 9 Constant current charge/discharge curves of supercapacitors with CoMn2O4 as the electrode at different current densities. | |
Table 1 Specific capacitance of CoMn2O4 for different methods
Sample |
Current density |
Specific capacitance |
Ref. |
CoMn2O4 microspheres |
1 A g−1 |
969 F g−1 |
This work |
CoMn2O4 spinel material |
1 A g−1 |
188 F g−1 |
Ren L. et al.35 |
CoMn2O4 electrode |
5 V s−1 |
700 F g−1 |
Vigneshwaran P. et al.36 |
Conclusion
The GSF method provided a new route for the mechanochemical synthesis of DMOFs, and is the successor and developed model of traditional solid phase synthesis methods. The GSF method overcame the defects in traditional solid-phase synthesis and achieved more efficient preparation. Thus, the GSF can be a novel and effective technique for the mechanochemical synthesis of DMOFs under solvent-free conditions. CoMn2(BTC)2 was successfully synthesized to confirm the feasibility of this method.35–40 CoMn2O4 microspheres were successfully prepared via the CoMn2(BTC)2 precursor method, and further exploration was carried out in the application. The results showed that CoMn2O4 had good capacitive behavior when used as a supercapacitor electrode. A specific capacitance of 969 F g−1 was obtained after charging and discharging at a current density of 1 A g−1.41–45
Conflicts of interest
The authors declare no conflicts of interest.
Acknowledgements
We are grateful for financial support from the National Natural Science Foundation of China (project no. 51972278), Major Frontier Innovation Project of Science and Technology Department of Sichuan Province (project no. 2019YJ0447), and Project of State Key Laboratory of Environment-friendly Energy Materials, Southwest University of Science and Technology (19 fksy04).
References
- M. X. Li, Y. X. Yin, C. J. Li, F. Z. Zhang, L. J. Wan, S. L. Xu and D. G. Evansa, Well-dispersed bi-component-active CoO/CoFe2O4 nanocomposites with tunable performances as anode materials for lithium-ion batteries, Chem. Commun., 2012, 48(3), 410–412 RSC.
- X. J. Hou, X. F. Wang, B. Liu, Q. F. Wang, T. Luo, D. Chen and G. Z. Shen, Hierarchical MnCo2O4 nanosheet arrays/carbon cloths as integrated anodes for lithium-ion batteries with improved performance, Nanoscale, 2014, 6(15), 8858–8864 RSC.
- J. Y. Zheng, X. L. Cui, Q. W. Yang, Q. L. Ren, Y. W. Yang and H. B. Xing, Shaping of ultrahigh-loading MOF pellet with a strongly anti-tearing binder for gas separation and storage, Chem. Eng. J., 2018, 354, 1075–1082 CrossRef CAS.
- J. R. Li, R. J. Kuppler and H. C. Zhou, Selective gas adsorption and separation in metal–organic frameworks, Chem. Soc. Rev., 2009, 38(5), 1477–1504 RSC.
- T. Rodenas, I. Luz, G. Prieto, B. Seoane, H. Miro, A. Corma, F. Kapteijn, X. Francesc, L. Xamena and J. Gascon, Metal–organic framework nanosheets in polymer composite materials for gas separation, Nat. Mater., 2015, 14(1), 48 CrossRef CAS.
- H. L. Wang, Q. L. Zhu, R. Q. Zou and Q. Xu, Metal-organic frameworks for energy applications, Chem, 2017, 2(1), 52–80 CAS.
- H. L. Li, M. Eddaoudi, M. O'Keeffe and O. M. Yaghi, Design and synthesis of an exceptionally stable and highly porous metal-organic framework, Nature, 1999, 402(6759), 276 CrossRef CAS.
- G. Y. Xu, P. Nie, H. Dou, B. Ding, L. Y. Li and X. G. Zhang, Exploring metal organic frameworks for energy storage in batteries and supercapacitors, Mater. Today, 2017, 20(4), 191–209 CrossRef CAS.
- L. Wang, Y. Z. Han, X. Feng, J. W. Zhou, P. F. Qi and B. Wang, Metal–organic frameworks for energy storage: Batteries and supercapacitors, Coord. Chem. Rev., 2016, 307, 361–381 CrossRef CAS.
- D. Yang and B. C. Gates, Catalysis by metal organic frameworks: perspective and suggestions for future research, ACS Catal., 2019, 9(3), 1779–1798 CrossRef CAS.
- J. Y. Lee, O. K. Farha, J. Roberts, K. A. Scheidt, S. T. Nguyena and J. T. Hupp, Metal–organic framework materials as catalysts, Chem. Soc. Rev., 2009, 38(5), 1450–1459 RSC.
- Y. Hua, X. X. Li, C. Y. Chen and H. Pang, Cobalt based metal-organic frameworks and their derivatives for electrochemical energy conversion and storage, Chem. Eng. J., 2019, 370, 37–59 CrossRef CAS.
- L. Takacs, The historical development of mechanochemistry, Chem. Soc. Rev., 2013, 42(18), 7649–7659 RSC.
- P. Baláž, M. Achimovičová, M. Baláž, P. Billik, Z. C. Zheleva, J. M. Criado, F. Delogu, E. Dutková, E. Gaffet, F. J. Gotor, R. Kumar, I. Mitov, T. Rojac, M. Senna, A. Streletskiikl and K. W. Ciurowam, Hallmarks of mechanochemistry: from nanoparticles to technology, Chem. Soc. Rev., 2013, 42(18), 7571–7637 RSC.
- S. L. James, C. J. Adams, C. Bolm, D. Braga, P. Collier, T. Friščić, F. Grepioni, K. D. M. Harris, G. Hyett, W. Jones, A. Krebs, J. Mack, L. Maini, A. G. Orpen, I. P. Parkin, W. C. Shearouse, J. W. Steed and D. C. Waddell, Mechanochemistry: opportunities for new and cleaner synthesis, Chem. Soc. Rev., 2012, 41(1), 413–447 RSC.
- D. E. Crawford, J. Casaban, R. Haydon, N. Giri, T. McNally and S. L. James, Synthesis by extrusion: continuous, large-scale preparation of MOFs using little or no solvent, Chem. Sci., 2015, 6(3), 1645–1649 RSC.
- G. W. Wang, Mechanochemical organic synthesis, Chem. Soc. Rev., 2013, 42(18), 7668–7700 RSC.
- T. Panda, S. Horike, K. Hagi, N. Ogiwara, K. Kadota, T. Itakura, M. Tsujimoto and S. Kitagawa, Mechanical Alloying of Metal-Organic Frameworks, Angew. Chem., 2017, 129(9), 2453–2457 CrossRef.
- T. D. Bennett, P. J. Saines, D. A. Keen, J. C. Tan and A. K. Cheetham, Ball-Milling-Induced Amorphization of Zeolitic Imidazolate Frameworks (ZIFs) for the Irreversible Trapping of Iodine, Chem. - Eur. J., 2013, 19(22), 7049–7055 CrossRef CAS.
- D. E. Crawford and J. Casaban, Recent developments in mechanochemical materials synthesis by extrusion, Adv. Mater., 2016, 28(27), 5747–5754 CrossRef CAS.
- B. Sun, Y. He, R. Peng, S. J. Chu and J. Zuo, Air-flow impacting for continuous, highly efficient, large-scale mechanochemical synthesis: a proof-of-concept study, ACS Sustainable Chem. Eng., 2016, 4(4), 2122–2128 CrossRef CAS.
- L. Zhou, D. Zhao and X. W. Lou, Double-shelled CoMn2O4 hollow microcubes as high-capacity anodes for lithium-ion batteries, Adv. Mater., 2012, 24(6), 745–748 CrossRef CAS.
- L. Wang, X. Zhao, Y. H. Lu, M. W. Xu, D. W. Zhang, R. S. Ruoff, K. J. Stevenson and J. B. Goodenough, CoMn2O4 spinel nanoparticles grown on graphene as bifunctional catalyst for lithium-air batteries, J. Electrochem. Soc., 2011, 158(12), A1379–A1382 CrossRef CAS.
- M. Prabu, P. Ramakrishnan and S. Shanmugam, CoMn2O4 nanoparticles anchored on nitrogen-doped graphene nanosheets as bifunctional electrocatalyst for rechargeable zinc-air battery, Electrochem. Commun., 2014, 41, 59–63 CrossRef CAS.
- J. Du, C. C. Chen, F. Y. Cheng and J. Chen, Rapid synthesis and efficient electrocatalytic oxygen reduction/evolution reaction of CoMn2O4 nanodots supported on graphene, Inorg. Chem., 2015, 54(11), 5467–5474 CrossRef CAS.
- C. X. Li, C. B. Chen, J. Y. Lu, S. Cui, J. Li, H. Q. Liu, W. W. Li and F. Zhang, Metal organic framework-derived CoMn2O4 catalyst for heterogeneous activation of peroxymonosulfate and sulfanilamide degradation, Chem. Eng. J., 2018, 337, 101–109 CrossRef CAS.
- L. Zhang, G. He, S. Lei, G. S. Qi, H. F. Jiu and J. Wang, Hierarchical hollow microflowers constructed from mesoporous single crystalline CoMn2O4 nanosheets for high performance anode of lithium ion battery, J. Power Sources, 2016, 326, 505–513 CrossRef CAS.
- J. L. Digol, M. F. M. Labata, M. F. Divinagracia and J. D. Ocon, CoMn2O4 Anchored on N-Doped High-Dimensional Hierarchical Porous Carbon Derived from Biomass for Bifunctional Oxygen Electrocatalysis, ECS Trans., 2017, 77(11), 525–531 CrossRef CAS.
- S. Alkhalaf, C. K. Ranaweera, P. K. Kahol, H. Adhikari, S. R. Mishra, F. Perez, B. K. Gupta, K. Ramasamy and R. K. Gupta, Electrochemical energy storage performance of electrospun CoMn2O4 nanofibers, J. Alloys Compd., 2017, 692, 59–66 CrossRef CAS.
- H. Zhu, D. Yu, S. G. Zhang, J. W. Chen, W. B. Wu, M. Wan, L. Wang, M. Zhang and M. L. Du, Morphology and structure engineering in nanofiber reactor: tubular hierarchical integrated networks composed of dual phase octahedral CoMn2O4/carbon nanofibers for water oxidation, Small, 2017, 13(26), 1700468 CrossRef.
- P. Mahata, D. Sarma, C. Madhu, A. Sundaresen and S. Natarajan, CoMn2O4 spinel from a MOF: synthesis, structure and magnetic studies, Dalton Trans., 2011, 40(9), 1952–1960 RSC.
- Y. Y. Fu, X. Z. Lu, K. Wan, Y. X. Zhang, Y. H. Yang, H. L. Quan, X. T. Xu and F. Wang, Spinel CoMn2O4 nanosheet arrays grown on nickel foam for high-performance supercapacitor electrode, Appl. Surf. Sci., 2015, 357, 2013–2021 CrossRef CAS.
- J. Cheng, X. Liu, Y. Lu, X. Y. Hou, H. Lei, H. L. Yan, J. Y. Xu and Y. S. Luo, Porous CoMn2O4 microspheres as advanced pseudocapacitive materials, Mater. Lett., 2016, 165, 231–234 CrossRef CAS.
- Q. Li, J. N. Guo, D. Xu, J. Q. Guo, X. Ou, Y. Hu, H. J. Qi and F. Yan, Electrospun N-Doped Porous Carbon Nanofibers Incorporated with NiO Nanoparticles as Free-Standing Film Electrodes for High-Performance Supercapacitors and CO2 Capture, Small, 2018, 14(15), 1704203 CrossRef.
- L. Ren, J. Chen, X. Q. Wang, M. J. Zhi, J. W. Wu and X. H. Zhang, Facile synthesis of flower-like CoMn2O4 microspheres for electrochemical supercapacitors, RSC Adv., 2015, 5(39), 30963–30969 RSC.
- P. Vigneshwaran, M. Kandiban, N. S. Kumar, V. Venkatachalam, R. Jayavel and I. Vetha Potheher, A study on the synthesis and characterization of CoMn2O4 electrode material for supercapacitor applications, J. Mater. Sci.: Mater. Electron., 2016, 27(5), 4653–4658 CrossRef CAS.
- S. Xu, S. Gong, H. Jiang, P. H. Shi, Q. J. Xu and Y. L. Min, Z-scheme heterojunction through interface engineering for broad spectrum photocatalytic water splitting, Appl. Catal., B, 2020, 267, 118661 CrossRef CAS.
- S. W. Luo, R. Gu, P. H. Shi, Q. J. Xu and Y. L. Min, Π-Π interaction boosts catalytic oxygen evolution by self-supporting metal-organic frameworks, J. Power Sources, 2020, 448, 227406 CrossRef CAS.
- S. Q. Gong, Z. J. Jiang, P. H. Shi, J. C. Fan and Y. L. Min, Noble-metal-free heterostructure for efficient hydrogen evolution in visible region: Molybdenum nitride/ultrathin graphitic carbon nitride, Appl. Catal., B, 2018, 238, 318–327 CrossRef CAS.
- T. Wu, Y. Ma, Z. B. Qu, J. C. Fan, Q. X. Li, P. H. Shi, J. C. Fan and Y. L. Min, Black phosphorus-graphene heterostructure-supported Pd nanoparticles with superior activity and stability for ethanol electro-oxidation, ACS Appl. Mater. Interfaces, 2019, 11(5), 5136–5145 CrossRef CAS.
- K. Lu, Z. J. Min, J. X. Qin, P. H. Shi, J. F. Wu, J. C. Fan, Y. L. Min and Q. J. Xu, Preparation of nitrogen self-doped hierarchical porous carbon with rapid-freezing support for cooperative pollutant adsorption and catalytic oxidation of persulfate, Sci. Total Environ., 2020, 142282 Search PubMed.
- X. Wang, X. F. Zhang, L. Dai, H. Guo, P. H. Shi, Y. L. Min and Q. J. Xu, Recycling the Cathode Scrap of Spent Lithium-Ion Batteries as an Easily Recoverable Peroxymonosulfate Catalyst with Enhanced Catalytic Performance, ACS Sustainable Chem. Eng., 2020, 8(30), 11337–11347 CrossRef CAS.
- J. Z. Zhang, S. Zhu, Y. L. Min and Q. J. Xu, Mn-doped perovskite-type oxide LaFeO3 as highly active and durable bifunctional electrocatalysts for oxygen electrode reactions, Front. Mater. Sci., 2020, 1–10 Search PubMed.
- J. Zhao and R. F. Peng, Gas-Solid Two-Phase Flow Synthesis Equipment: A New Method for Continuous, Large-Scale Preparation of Metal-Organic Frameworks with No Solvent, Ind. Eng. Chem. Res., 2020, 59(35), 15791–15795 CrossRef CAS.
- J. Zhao, B. Jin and R. F. Peng, New Core-Shell Hybrid Material IR-MOF3@COF-LZU1 for highly efficient-visible-light photocatalyst-degrading nitro-aromatic explosives, Langmuir, 2020, 36, 5665–5670 CrossRef.
Footnote |
† Electronic supplementary information (ESI) available: The prepared CoMn2(BTC)2 was characterized by FT-IR, DTA, TG/DTG, and XRD studies (Fig. S1–S4). See DOI: 10.1039/d0na00749h |
|
This journal is © The Royal Society of Chemistry 2020 |
Click here to see how this site uses Cookies. View our privacy policy here.