DOI:
10.1039/D0NA00746C
(Paper)
Nanoscale Adv., 2020,
2, 5271-5279
Prediction of two-dimensional CP3 as a promising electrode material with a record-high capacity for Na ions†
Received
5th September 2020
, Accepted 23rd September 2020
First published on 23rd September 2020
Abstract
Borophene with a maximum Li/Na capacity of 1984 mA h g−1 (nanoscale 2016, 8, 15
340–15
347) has shown the highest capacity among two-dimensional (2-D) anode materials identified so far. Herein, we report the record break for Na-ion using a newly proposed 2-D material, namely, CP3. We fully investigated Li- and Na-ion adsorption and diffusion processes on a CP3 monolayer. We found that the material can enable stable Li/Na adsorption considering charge accumulation on CP3 surfaces. The ion diffusion barriers for Li and Na were identified to be 98 meV and 356 meV, respectively. These values were comparable or smaller than those of the typical high-capacity electrode materials such as borophene. Most remarkably, the maximum Na capacity in CP3 monolayer can reach up to 2298.9 mA h g−1, which breaks the value recorded using borophene (1984 mA h g−1). Our work highly promises that the 2-D CP3 material could serve as an outstanding electrode material for Na-ion batteries with an extremely high storage capacity.
1. Introduction
Li-ion battery (LIB) technology has been one of the most successful breakthroughs in recent decades. LIBs are currently used in various fields because of their high performances on energy storage and electrical operation.1–5 Meanwhile, they are still suffering from bottlenecks for application in large-scale electrical devices because of intrinsic issues such as safety and costs.6–10 To relieve these issues, continuous efforts have been made on two aspects. One aspect focuses on developing high-performance and cost-effective LIB electrode materials. For this consideration, two-dimensional (2-D) electrodes fall into the spotlight, because their unique geometric configurations can favor the ion diffusion and storage processes.11–19 As accompanied, it has seen an explosion of 2-D electrode development in recent years, nearly covering all layered materials.20–40 For the other aspect, great efforts were made on developing other types of ion batteries.41–47 Among various potential ion batteries, Na-ion batteries (NIBs) have attracted much attention because of excellent security, low costs, and similar operating mechanisms with LIBs.21–23,32 There has already been a rapid progress in NIB development; however, further development of LIBs face crucial challenges. One of the challenges is the lack of excellent LIB anode material, in particular those that can enable high capacity. Currently, the storage capacity of 2-D anode materials for NIBs is mostly in the range of 500–800 mA h g−1; hence, there is an urgently need to explore excellent LIB anode materials that can enable higher capacities.
In 1970s, two typical layered materials, namely, GeP3 and SnP3, have been synthesized.48–50 Soon after, the great feasibility of exfoliating GeP3 and SnP3 monolayers from their bulk materials has been theoretically reported.51–54 Besides, it was found that these freestanding monolayers possess good thermodynamic and mechanical stabilities, which can exist steadily. As it is known, carbon, germanium and tin (C, Ge and Sn) belong to the same group in the periodic table of elements. Then, one may wonder whether the material of CP3 can stably exist? Whether it is a layered material like GeP3 and SnP3? Further, is it possible to peel off monolayer from the bulk? These questions have been solved by the following studies. Ramzan et al. reported for the first time the excellent dynamic stability of CP3 layered materials, which have a similar structure to GeP3 and SnP3 counterparts.55 Immediately after, Sarkar et al. reported that the monolayer form of CP3 has a relatively low cleavage energy, which can be readily cleaved from its bulk phases like other layered materials.56
In this work, we report for the first time that 2-D CP3 is a very promising electrode material for LIBs and NIBs. As realized by first-principles calculations, we have thoroughly simulated the Li/Na atom adsorption and diffusion processes on CP3 monolayers. We realize that the CP3 monolayer can enable stable Li/Na adsorption and considerable charge transfers are observed during the period. The CP3 electrode hosts good conductivity, considering the definite metallic electronic structures both before and after adsorptions. The ion diffusion path and the minimum migration barriers for Li/Na atoms on CP3 monolayer are obtained, which are comparable or lower than those of 2-D electrodes with high storage capacity. Besides, the storage capacity and the average open circuit voltage have been systematically investigated. The results indicated that the CP3 monolayer has a low average open circuit voltage for Li/Na, which is suitable to be used as an anode material. Most excitingly, the maximum capacity of the CP3 monolayer for Na-ion has reached a new record for 2-D anode materials, which is 2298.9 mA h g−1. In particular, 2-D CP3 can provide higher capacity (2298.9 mA h g−1versus 1984 mA h g−1) than the famous anode material borophene23 with a comparable diffusion barrier (356 meV versus 340 meV).
2. Computational details
In this work, we performed the first-principles calculations using the Vienna ab initio simulation package (VASP),57 based on density functional theory (DFT).58 For the exchange-correlation potential, we applied the generalized gradient approximation (GGA) of the Perdew–Burke–Ernzerhof (PBE) functional.59,60 The cutoff energy was set as 500 eV during the calculations. A vacuum space with a thickness of 30 Å was built in the bare CP3 monolayer to avoid artificial interaction between two single monolayers. During the calculations, the long-range van der Waals interactions were taken into account by using the DFT-D2 method.61 The Brillouin zone was sampled with a 7 × 7 × 1 Monkhorst–Pack k-point mesh for the geometrical optimization and with a 9 × 9 × 1 k-mesh for the calculations of the electronic structure and atomic adsorptions. All atomic positions were fully relaxed during the calculations, and the force and energy convergence criteria were set as 0.01 eV Å−1 and 10−6 eV, respectively. To investigate the dynamical stability of the CP3 monolayer, the phonon spectra was calculated using the PHONOPY package.62,63 The climbing-image nudged elastic band (CI-NEB) method was used to obtain the diffusion barrier height during the ion diffusion process.64,65
3. Results and discussions
3.1 Structure and stability of the CP3 monolayer
As shown in Fig. 1(a), the bulk phase of CP3 material has the so-called ABC-type layered structure (the space group is R
m), which is similar to the GeP3 and SnP3 counterparts. Fig. 1(b) shows the structure of the CP3 monolayer. Sarkar et al. have proposed two potential fabrication methods of the CP3 monolayer.56 For the first one, it may be directly cleaved from its layered bulk structure, and shown by the process in Fig. 1(a) and (b). Here, we evaluated the cleavage energy for the CP3 monolayer. A five-layer system was used in our calculations, and the computation details are displayed in the ESI.† The cleavage energy of the CP3 monolayer was calculated to be 0.65 J m−2, which well agrees with the previous work (0.57 J m−2).56 This cleavage energy was lower than the DFT-calculated exfoliation energy for Ca2N (1.08 J m−2),66 InP3 (1.32 J m−2),67 and GeP3 (1.14 J m−2).68 For this consideration, the CP3 monolayer was quite promising to be exfoliated experimentally from its bulk phase. Besides, Sarkar et al. proposed that the CP3 monolayer was promising to be synthesized by doping C atoms into blue phosphorene,56 as displayed by the process in Fig. 1(c).
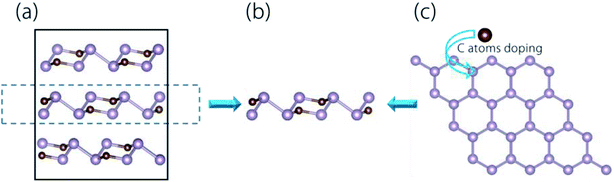 |
| Fig. 1 (a) Optimized structure of bulk CP3 with a 2 × 2 × 1 supercell. (b) Crystal structure of the CP3 monolayer delaminated from bulk CP3. (c) C atoms doping into blue phosphorene, showing another potential CP3 monolayer synthesis process. | |
In addition, we wanted to further ensure the stability of the CP3 monolayer. We estimated the cohesive energy (Ec) of the CP3 monolayer, which can be described as follows:
| Ec = (Etotal − mEC − nEP)/(m + n) | (1) |
In this equation, Etotal, EC, and EP are on behalf of the energies of the CP3 monolayer, single C and P atoms, respectively; n and m are the number of C and P atoms in the CP3 monolayer. The calculated formation energy is −4.12 eV per unit cell, which is consistent with previous results.56 In addition, it suggests that the CP3 monolayer is energetically stable.
We make further discussions on the structure of the CP3 monolayer, and top and side views are shown in Fig. 2(a) and (b), respectively. In the structure, we can find that each C atom bonds with three P atoms, and each P atom bonds with one C atom and two P atoms. Such bonding forms the connection of the P6 ring and C2P4 ring, as shown by the top view in Fig. 2(a). The optimized lattice constants of the CP3 monolayer is 6.22 Å, and the length of the C–P bond and P–P bond is 1.78 Å and 2.28 Å, respectively. These results are in good agreement with the previous proposal.56 Based on the optimized structure, we study the dynamical stability of the CP3 monolayer. The resulting phonon dispersion is displayed in Fig. 3(a). Apparently, one finds no imaginary frequency in the phonon spectra. Thus, the CP3 monolayer is believed to be dynamically stable. In addition, we show the band structure and density of state (DOSs) of the CP3 monolayer in Fig. 3(c) and (d), respectively. We can find that the CP3 monolayer shows a definite metallic electronic structure. This can enable sound conductivity when applied as an electrode material. Moreover, from the DOSs in Fig. 3(d), one can find that the electronic states near the Fermi energy are mainly provided by the p orbitals of C and P atoms.
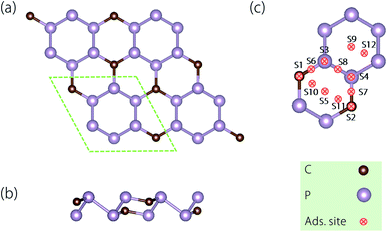 |
| Fig. 2 Crystal structures of the CP3 monolayer in (a) the top and (b) the side views. (c) The considered adsorption sites (denoted as S1–S12) on the surface of the CP3 monolayer (top view). | |
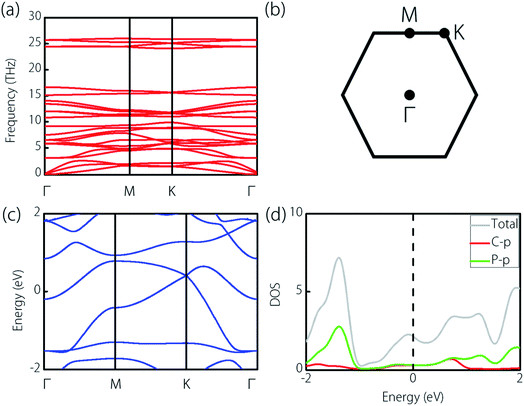 |
| Fig. 3 (a) Phonon spectra of the fully relaxed CP3 monolayer. (b) 2-D Brillouin zone of the CP3 monolayer. (c) Band structures of the CP3 monolayer. (d) Total and projected density of states of the CP3 monolayer. | |
3.2 Ion adsorption and diffusion
Then, we investigate the ion adsorption and diffusion on the CP3 monolayer. Herein, we focus on the cases for Li and Na, and the 2 × 2 supercell CP3 monolayer is considered as the substrate for the adsorption. By lattice symmetry of the CP3 monolayer, we consider nine typical adsorption sites. As shown in Fig. 2(c), these adsorption sites are denoted as S1–S12 (among them, S1–S4 are the top sites, S5 and S9 are the hollow sites, S6–S8 and S10–S12 are the bridge sites). The adsorption energy (Ead) of Li/Na atoms on the CP3 monolayer is defined as follows: | Ead = ELi/Na+CP3 − ECP3 − ELi/Na | (2) |
where ELi/Na is the energy per atom for the bulk metal Li/Na, ELi/Na+CP3 and ECP3 are the total energies of the CP3 monolayer after and before Li/Na adsorption. A negative value of adsorption energy means that Li/Na atoms tend to adsorb onto the CP3 monolayer, instead of forming a bulk metal. The calculated adsorption energies for Li/Na are shown in Fig. 4(a). All values of adsorption energy are negative, which indicates that Li/Na atoms can stably adsorb onto the CP3 monolayer.
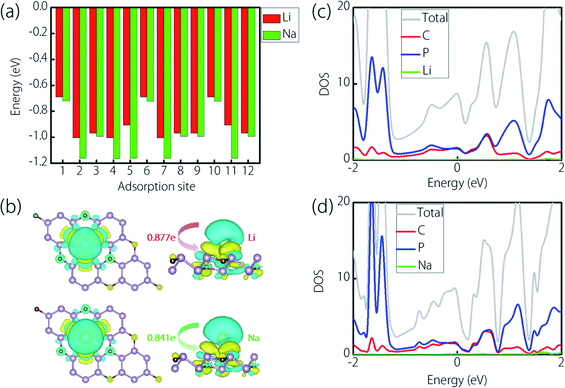 |
| Fig. 4 (a) Li/Na adsorption energies of the twelve possible adsorption sites on the surface of the CP3 monolayer. (b) Maps of charge density difference in the CP3 monolayer with Li/Na adsorption as well as the amounts of charge transfer. (c) and (d) Density of states (DOS) for Li- and Na-adsorbed CP3 monolayers, respectively. | |
After examining the optimized structure, we found that the Li atom at the S2, S4, and S7 sites would automatically shift to the bridge site (nearby S7, for clarity, we redefine this new adsorption site as S7); the Li atom at the S5 site would move to the S11 site; the Li atom at the S6 and S10 sites would move to the S1 one and that at the S3, S8, and S12 sites would move to the S9 one. The occasion for Na is slightly different. Adatoms at the S4, S5, S7, and S11 sites would automatically shift to the S2 site; the Na atom at the S3, S8, and S12 sites would move to the S9 one; the Na atom at the S6 and S10 sites would move to the S1 one. To sum up, Li has four stable adsorption sites on the CP3 monolayer, while Na has three stable adsorption sites on it. For these sites, as for Li, the stability is in the order: S7 > S9 > S11 > S1 (−1.005 eV < −0.968 eV < −0.907 eV < −0.689 eV). With regard to Na, the stability order is S2 > S9 > S1 (−1.163 eV < −0.993 eV < −0.721 eV). Besides, we compared the adsorption height at different sites, we found that the order is S11 < S7 < S9 < S1 for Li (which are 1.707 Å < 1.908 Å < 2.323 Å < 2.375 Å), and S2 < S9 < S1 for Na (which are 1.973 Å < 2.799 Å < 2.849 Å). In general, these values show a trend that the lower the ion adsorption height, the more stable the adsorption that the site can enable.
To explore the charge transfer of Li/Na atoms and the CP3 monolayer, we calculated the charge density difference (CDD) during the ion adsorption. Here, the stable adsorption site (S1) was applied. As shown in Fig. 4(b), the green regions represent charge accumulation and the cyan regions represent charge depletion. We can clearly observe the obvious charge transfers to the CP3 monolayer from Li/Na atoms. By the way, we also calculated the specific amount of the transferred charge using the Bader charge calculations.69 The results indicated that the transferred charge is 0.877e per atom for Li and 0.841e per atom for Na, respectively. Therefore, we realize that Li/Na atoms are chemically adsorbed on the CP3 monolayer. In addition, we displayed the projected density of states (PDOSs) for Li/Na atoms adsorbed on the CP3 monolayer in Fig. 4(c) and (d), respectively. We can clearly find that the CP3 monolayer still possesses metallic conductivity after the adsorption, which enables the great feasibility of the CP3 monolayer as an electrode material for ion batteries.
The diffusion performance of adatoms on the electrode is closely related to its rate capability, and low diffusion is highly required for electrode materials. Here, we estimated the Li/Na diffusion ability on the CP3 monolayer using the CI-NEB calculations. On account of the structural symmetry and adsorption sites, three most possible ion diffusion paths (P–A, P–B, and P–C) were taken into account for Li, and two most possible ion diffusion paths (P–A and P–B) were taken into account for Na. These are shown in Fig. 5(a). As for Li, the diffusion route for P–A is that: the Li atom moves from the S7 site to S11 one, and finally moves to another S7 one. In P–B, the Li atom moves from the S7 site to the S9 one, and finally moves to another S7 one. In P–C, the Li atom moves from the S7 site to the S1 one, and finally moves to another S7 one. As for Na, the diffusion route for P–A is that: the Na atom moves from the S2 site to S9 one, and finally moves to another S2 one. In P–B, the Na atom moves from the S2 site to the S1 one, and finally moves to another S2 one. The calculated diffusion profiles are shown in Fig. 5(b). We can find that the Li diffusion energy for P–A is lower than those of P–B and P–C, while the Na diffusion energy for P–B is the lower than that of P–A. Herein, the diffusion barrier is as low as 98 meV for Li and 356 meV for Na. These diffusion barriers are moderate when comparing with typical 2-D electrode materials. For example, they are a bit higher (or comparable) than electrode materials such as Ti3C2 (68 meV for Li and 96 meV for Na),26 blue-phosphorene (130 meV for Li and 110 meV for Na),70,71 and so on. However, they are much lower than graphene (370 meV for Li),22 MoN2 (780 meV for Li, and 560 meV for Na),33 and β12/χ3 borophene (600/660 meV for Li, and 300/340 meV for Na).23,24 These results indicated that the CP3 monolayer would enable good ion diffusion ability when applied as battery electrodes.
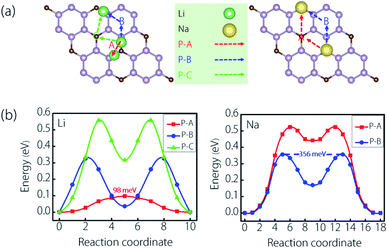 |
| Fig. 5 (a) Potential Li/Na ion diffusion paths and (b) the corresponding ion diffusion profiles on the CP3 monolayer as well as the size of the minimum diffusion barrier. | |
3.3 Storage capacity and open circuit voltage
Besides the diffusion barrier, the storage capacity and the average open circuit voltage are important characters for the electrode materials. To obtain the maximum possible storage of Li/Na, we calculated the average adsorption energies of Li/Na atoms for layers. Here, we still use the 2 × 2 supercell of the CP3 monolayer as the substrate, then display the adatoms on the CP3 monolayer layer by layer. Therefore, the average adsorption energy in each layer (Eave) can be defined as follows: | Eave = (ELi8n/Na8n+C8P24 − ELi(n−1)8/Na(n−1)8+C8P24 − 8ELi/Na)/8 | (3) |
Here, ELi/Na is the energy per atom in bulk Li/Na metals; ELi8n/Na8n+C8P24 and ELi(n−1)8/Na(n−1)8+C8P24 are the total energies of the CP3 monolayer with “n” and “n − 1” Li/Na adsorption layers. The number “8” represents eight Li/Na atoms adsorbed on both sides of the 2 × 2 supercell per layer. According to the adsorption energies, the adsorption sequence for Li can be described as: S7 (the first layer) – S9 (the second layer) – S11 (the third layer) – S1 (the fourth layer), and the adsorption sequence for Na can be described as: S2 (the first layer) – S9 (the second layer) – S1 (the third layer).
For Li adsorption on the CP3 monolayer, we found that only two layers can be stably adsorbed with negative Eave, and the values are −0.893 eV for the first layer and −0.172 eV for the second layer, respectively. As the results, we realize that the CP3 monolayer can at most enable 18 Li atoms adsorbed on the surface. The optimized structures for one and two adsorption layers are shown in Fig. 6(a). In addition, and the other side views of optimized structures are provided in the ESI.† As for Na, we are delighted to find that the CP3 monolayer can enable extremely high ability for Na adsorption. With the triple-layer configuration (S2–S9–S1) as the period, we are surprised to find that three of such adsorption periods can show negative adsorption energy. In addition, the average adsorption energy is −0.600 eV for the first triple-layers, −0.120 eV for the second triple-layers, and −0.196 eV for the third triple-layers, respectively. Therefore, nine Na layers (totally 72 Na atoms) can be adsorbed on the CP3 monolayer. Likewise, we show these optimized structures in Fig. 6(b), and the other side views of them are provided in the ESI.† In addition, we investigated the mechanical property of the CP3 monolayer before and after adsorption. The elastic constants, the Young modulus and the Poisson ratio were calculated (the results are shown in the ESI†). The elastic constants for all the cases satisfy the Born criteria (C44 > 0; C11C22 − C122 > 0). This indicates that the CP3 monolayer is mechanically stable before and after Li/Na adsorption.
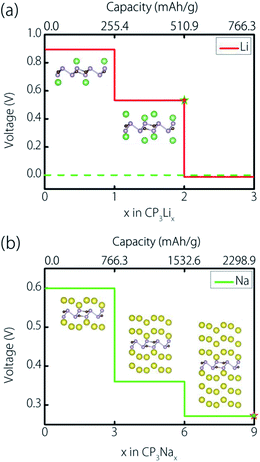 |
| Fig. 6 Voltage profiles and specific ion storage capacities for (a) Li and (b) Na adsorption on the CP3 monolayer. In (a) and (b), the optimized structures of CP3Lix and CP3Nax on the step and the maximum Li and Na capacities (the star) are provided. | |
As for CP3 monolayer, the typical half–cell reaction can be defined as follows:
| CP3 + xLi+/Na+ + xe− ↔ Li/NaCP3 | (4) |
Accordingly, we can estimate the open circuit voltage (OCV) from the following equation:
| Vave = (ECP3 + xELi/Na − ELi/NaCP3)/xe | (5) |
where
x represents the number of Li/Na atoms, and other parameters are the same for those in
eqn (1). The voltage profiles are provided in
Fig. 6(a) and (b), respectively. In the figures, we find the OCV for Li is in the range of 0.53–0.89 V, and that for Na is in the range of 0.27–0.60 V. These OCV values suggest that the CP
3 monolayer is feasible to become an electrode material for LIBs and NIBs.
The above discussions show that a 2 × 2 supercell could accommodate up to 18 atoms of Li and 72 atoms of Na, respectively. Such adsorptions correspond to the chemical stoichiometry of CP3Li2 and CP3Na9. Finally, we can obtain the maximum storage capacity (Cm) using the following equation:
In this equation, xm is the maximum Li/Na concentration (for the current case, xm = 2 for Li; xm = 9 for Na); F is the Faraday constant (26.8 A h mol−1); and MCP3 is the molecular mass of CP3. As a result, the storage capacities of Li and Na on the CP3 monolayer are 510.9 mA h g−1 and 2298.9 mA h g−1, respectively.
Before ending, we want to emphasize the extremely high capacity for Na on the CP3 monolayer. Here, in Fig. 7, we list some typical 2-D anode materials for the maximum capacity of NIBs. They include Ti3C2 (352 mA h g−1),26 Mn2C (444 mA h g−1),72 2-D GaN (625 mA h g−1),73 blue phosphorene (865 mA h g−1),71 black phosphorene (865 mA h g−1),72 MnSb2S4 (879 mA h g−1),74 GeP3 (1295 mA h g−1),75 and β12/χ3 borophene (1240/1984 mA h g−1).23,24 It is very exciting to note that the maximum Na-ion capacity of the current CP3 monolayer is up to 2298.9 mA h g−1, which is much higher than all the examples listed in Fig. 7. We also examined the capacities of other 2-D Na anodes reported in the literature, and to the best of our knowledge, we found that the CP3 monolayer still has the highest Na-ion storage capacity. As we all know, high storage capacity is one of the most crucial performances for electrode materials. The results in our work indicate that the CP3 monolayer can be expected to be a 2-D anode material for NIBs with the record-high storage capacity.
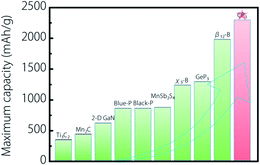 |
| Fig. 7 Comparison of CP3 monolayer with typical 2-D anode materials for the maximum Na-ion capacity. | |
3.4 Additional discussions
Before ending, we have several remarks. First, we have recently become aware of a similar work that focused on the CP3 monolayer.78 For the first time, the literature proposed the feasibility of the CP3 monolayer as a potential electrode material for Na-ion batteries.78 Although the literature has focused on the same material, we want to point out that our work still has two crucial differences. First, besides the Na-ion batteries, we studied the feasibility of the CP3 monolayer as an electrode for Li-ion batteries and found that the material shows an extremely low diffusion barrier of 98 meV and a moderate storage capacity of 510.9 mA h g−1 for Li ions, while the literature did not discuss the case of Li. Second and most importantly, the literature has greatly underestimated the capacity for Na (1022 mA h g−1). This may imply that some adsorption possibilities were not taken into account. In our work, we found that a CP3 substrate can support more Na adsorption than those reported in the literature. We have comprehensively considered twelve possible adsorption sites and found three favorable adsorption sites after full geometrical relaxations. Our calculations identified one more stable adsorption site than reported in the literature, which locates on the top of one C atom [S1 site in Fig. 2(c)]. In our calculations, at the beginning, we add Na atoms layer by layer on the CP3 substrate. Very interestingly, after adding three layers of Na atoms, we found that the CP3 substrate can stably adsorb more Na atoms. To simplify, we consider three layers of Na atoms as a period. Our calculations showed that the CP3 substrate can adsorb up to three periods of such adsorption. As the result, the maximum capacity for Na is in fact 2298.9 mA h g−1. This capacity is the highest among known 2-D anode materials for Na ions. The record-high capacity is the most important innovation point in this work.
Second, our calculations show that the CP3 monolayer can support the multilayer adsorption for Na ions, while not for Li ions. For the case of Li, the CP3 monolayer can stably adsorb two layers. When the third layer is displayed, we can find that the final structure breaks down [see ESI†]. The potential reason is that the adsorption height for Li is much lower than that for Na (1.795 Å vs. 2.104 Å for the first layer; 2.516 Å vs. 3.175 Å for the second layer). This induces more powerful interaction between the Li adatoms and CP3 monolayer than that of Na; hence, the structure collapses when multiple layers of Li are displayed. In addition, we found that such phenomenon also happens in many other 2-D electrode materials. For instance: Ca2N and Sr2N can adsorb multiple layers of Na but no Li;32 GeP3,75,76 MoN2,33 and GeS68 can adsorb multiple layers of Na but only one layer of Li.
Third, we want to point out that the supercell size of the substrate sometimes would affect the adsorption and ion diffusion properties. In the above discussions, the 2 × 2 supercell of the CP3 monolayer was applied. We tested the Li/Na adsorption and diffusion processes in the 3 × 3 and the 4 × 4 supercells. We found that the most favorable adsorption site and the maximum capacity are not changed in the 3 × 3 and the 4 × 4 supercells. In addition, the minimum diffusion barrier is only slightly changed for Na (356 meV for 2 × 2 supercell vs. 336 meV for 3 × 3 supercell vs. 329 meV for 4 × 4 supercell). These results indicated that the 2 × 2 supercell of the CP3 monolayer was suitable for investigating the adsorption and diffusion processes in this work. Moreover, the size of the 2 × 2 supercell was also applied to similar structures such as GeP3 (ref. 75 and 76) and SnP3.77
4. Conclusion
In conclusion, we have systematically investigated the performance of a CP3 monolayer as a potential LIB/NIB anode material by the first-principles calculations. We realized that Li/Na atoms could steadily adsorb onto the CP3 monolayer surface, and the corresponding adsorption energies and adsorption sites were obtained. We showed that the CP3 monolayer possesses good metallic conductivity before and after adsorptions, which is desirable for electrode materials. In addition, the average OCV and ion diffusion paths have been determined. The results indicated that the lowest diffusion barrier is 98 meV for Li and 356 meV for Na, which are comparable or much lower than those of most high-capacity 2-D electrode materials such as borophene. Most attractively, we realized that the storage capacity of Na on the CP3 monolayer can be up to 2298.9 mA h g−1, which is the highest among 2-D NIB anodes identified so far. To sum up, these outstanding performances suggest that the CP3 monolayer is a potential NIB anode material with extremely high storage capacity.
Conflicts of interest
There are no conflicts to declare.
Acknowledgements
This work was supported by National Natural Science Foundation of China (grant numbers 51871089, 61674051), the Program for Guangdong Introducing Innovative and Enterpreneurial Teams (No. 2016ZT06G025), and the open subject of State Key Laboratory of Research and Comprehensive Utilization of Rare Earth Resources in Baiyun Ebo (No. 2020z2123). One of the authors (X. M. Zhang) acknowledges the financial support from Young Elite Scientists Sponsorship Program by Tianjin.
References
- S. Liu, W. Xu, C. Ding, J. Yu, D. Fang, Y. Ding and H. Hou, Boosting electrochemical performance of electrospun silicon-based anode materials for lithium-ion battery by surface coating a second layer of carbon, Appl. Surf. Sci., 2019, 494, 94–100 CrossRef CAS
.
- H. D. Chen, K. X. Shen, X. H. Hou, G. Z. Zhang, S. F. Wang, F. M. Chen, L. J. Fu, H. Q. Qin, Y. C. Xia and G. F. Zhou, Si-based anode with hierarchical protective function and hollow ring-like carbon matrix for high performance lithium ion batteries, Appl. Surf. Sci., 2019, 470, 496–506 CrossRef CAS
.
- Q. F. Fu, R. J. Li, X. Z. Zhu, G. S. Liang, L. J. Luo, Y. J. Chen, C. F. Lin and X. S. Zhao, Design, synthesis and lithium-ion storage capability of Al0.5Nb24.5O62, J. Mater. Chem. A, 2019, 7, 19862–19871 RSC
.
- X. Z. Zhu, J. Xu, Y. P. Luo, Q. F. Fu, G. S. Liang, L. J. Luo, Y. J. Chen, C. F. Lin and X. S. Zhao, MoNb12O33 as a new anode material for high-capacity, safe, rapid and durable Li+ storage: structural characteristics, electrochemical properties and working mechanisms, J. Mater. Chem. A, 2019, 7, 6522–6532 RSC
.
- X. M. Lou, R. J. Li, X. Z. Zhu, L. J. Luo, Y. J. Chen, C. F. Lin, H. L. Li and X. S. Zhao, New anode material for lithium-ion batteries: aluminum niobate (AlNb11O29), ACS Appl. Mater. Interfaces, 2019, 11, 6089–6096 CrossRef CAS
.
- J. R. Dahn, T. Zheng, Y. Liu and J. S. Xue, Mechanisms for Lithium Insertion in Carbonaceous Materials, Science, 1995, 270, 590–593 CrossRef CAS
.
- M. Winter, J. O. Besenhard, M. E. Spahr and P. Novak, Insertion Electrode Materials for Rechargeable Lithium Batteries, Adv. Mater., 1998, 10, 725–763 CrossRef CAS
.
- J. M. Tarascon, Is lithium the new gold?, Nat. Chem., 2010, 2, 510 CrossRef CAS
.
- J. B. Goodenough and K. S. Park, The Li-ion rechargeable battery: a perspective, J. Am. Chem. Soc., 2013, 135, 1167–1176 CrossRef CAS
.
- J. M. Tarascon and M. Armand, Issues and challenges facing rechargeable lithium batteries, Nature, 2001, 414, 359–367 CrossRef CAS
.
- J. Liu and X. W. Liu, Two-dimensional nanoarchitectures for lithium storage, Adv. Mater., 2012, 24, 4097–4111 CrossRef CAS
.
- H. Zhang, Ultrathin two-dimensional nanomaterials, ACS Nano, 2015, 9, 9451–9469 CrossRef CAS
.
- C. Tan, X. Cao, X. J. Wu, Q. He, J. Yang, X. Zhang, J. Chen, W. Zhao, S. Han, G. H. Nam, M. Sindoro and H. Zhang, Recent advances in ultrathin two-dimensional nanomaterials, Chem. Rev., 2017, 117, 6225–6331 CrossRef CAS
.
- N. Oyama, T. Tatsuma, T. Sato and T. Sotomura, Dimercaptan–Polyaniline Composite Electrodes for Lithium Batteries with High Energy Density, Nature, 1995, 373, 598–600 CrossRef CAS
.
- J. M. Tarascon and M. Armand, Issues and Challenges Facing Rechargeable Lithium Batteries, Nature, 2001, 414, 359–367 CrossRef CAS
.
- H. Chen, T. N. Cong, W. Yang, C. Tan, Y. Li and Y. Ding, Progress in Electrical Energy Storage System: A Critical Review, Prog. Nat. Sci., 2009, 19, 291–312 CrossRef CAS
.
- B. Dunn, H. Kamath and J. M. Tarascon, Electrical Energy Storage for the Grid: A Battery of Choices, Science, 2011, 334, 928–935 CrossRef CAS
.
- W. Li, Y. Yang, G. Zhang and Y. W. Zhang, Ultrafast and Directional Diffusion of Lithium in Phosphorene for High-Performance Lithium-Ion Battery, Nano Lett., 2015, 15, 1691–1697 CrossRef CAS
.
- D. P. Dubal, O. Ayyad, V. Ruiz and P. Gomez-Romero, Hybrid Energy Storage: the Merging of Battery and Supercapacitor Chemistries, Chem. Soc. Rev., 2015, 44, 1777–1790 RSC
.
- K. J. Naresh, B. A. Rafael, S. Vivekanand and A. Rajeev, Borophane as a Benchmate of Graphene: A Potential 2D Material for Anode of Li and Na-Ion Batteries, ACS Appl. Mater. Interfaces, 2017, 9, 16148–16158 CrossRef
.
- J. Sun, H. W. Lee, M. Pasta, H. Yuan, G. Zheng, Y. Sun, Y. Li and Y. Cui, A Phosphorene-Graphene Hybrid Material as a High-Capacity Anode for Sodium-Ion Batteries, Nat. Nanotechnol., 2015, 10, 980–985 CrossRef CAS
.
- E. Pollak, B. Geng, K. J. Jeon, I. T. Lucas, T. J. Richardson, F. Wang and R. Kostecki, The Interaction of Li+ with Single-Layer and Few-Layer Graphene, Nano Lett., 2010, 10, 3386–3388 CrossRef CAS
.
- X. M. Zhang, J. P. Hu, Y. H. Cheng, H. Y. Yang, Y. G. Yao and S. A. Yang, Borophene as an Extremely High Capacity Electrode Material for Li-Ion and Na-Ion Batteries, Nanoscale, 2016, 8, 15340–15347 RSC
.
- H. R. Jiang, Z. Lu, M. C. Wu, F. Ciucci and T. S. Z. Borophene, A Promising Anode Material Offering High Specific Capacity and High Rate Capability for Lithium-Ion Batteries, Nano Energy, 2016, 23, 97–104 CrossRef CAS
.
- D. Datta, J. Li and V. B. Shenoy, Defective graphene as a high-capacity anode material for Na- and Ca-ion batteries, ACS Appl. Mater. Interfaces, 2014, 6, 1788–1795 CrossRef CAS
.
- D. Q. Er, J. W. Li, M. Naguib, Y. Gogotsi, V. B. ShenoyEr, D. Li, J. Naguib, M. Gogotsi and Y. Shenoy, Ti3C2 MXene as a high capacity electrode material for metal (Li, Na, K, Ca) ion batteries, ACS Appl. Mater. Interfaces, 2014, 6, 11173–11179 CrossRef CAS
.
- Q. Sun, Y. Dai, Y. Ma, T. Jing, W. Wei and B. Huang,
Ab initio prediction and characterization of Mo2C monolayer as anodes for lithium-ion and sodium-ion batteries, J. Phys. Chem. Lett., 2016, 7, 937–943 CrossRef CAS
.
- D. Çakır, C. Sevik, O. Gülserenc and F. M. Peetersa, Mo2C as a high capacity anode material: a first-principles study, J. Mater. Chem. A, 2016, 4, 6029–6034 RSC
.
- J. Hu, B. Xu, C. Ouyang, S. A. Yang and Y. Yao, Investigations on V2C and V2CX2 (X = F, OH) Monolayer as a Promising Anode Material for Li Ion Batteries from First-Principles Calculations, J. Phys. Chem. C, 2014, 118, 24274–24281 CrossRef CAS
.
- J. Hu, B. Xu, C. Ouyang, Y. Zhang and S. A. Yang, Investigations on Nb2C Monolayer as Promising Anode Material for Li or Non-Li Ion Batteries from First-Principles Calculations, RSC Adv., 2016, 6, 27467–27474 RSC
.
- Q. Wan, S. Li and J. B. Liu, First-Principle Study of Li-Ion Storage of Functionalized Ti2C Monolayer with Vacancies, ACS Appl. Mater. Interfaces, 2018, 10, 6369–6377 CrossRef CAS
.
- J. Hu, B. Xu, S. A. Yang, S. Guan, C. Ouyang and Y. Yao, 2D Electrides as Promising Anode Materials for Na-Ion Batteries from First-Principles Study, ACS Appl. Mater. Interfaces, 2015, 7, 24016–24022 CrossRef CAS
.
- X. M. Zhang, Z. M. Yu, S. S. Wang, S. Guan, H. Y. Yang, Y. G. Yao and S. A. Yang, Theoretical Prediction of MoN2 Monolayer as a High Capacity Electrode Material for Metal Ion Batteries, J. Mater. Chem. A, 2016, 4, 15224–15231 RSC
.
- S. U. Rehman, S. A. Khan, W. Uddin, Q. U. Khan, M. Kiain, I. Mehmood, M. Sohail, M. Saeed, S. Kumar and L. Zhu, First principle study of new W2N monolayer: a promising candidate for Li+ ion batteries, Int. J. Electrochem., 2019, 14, 3070–3080 CrossRef CAS
.
- Z. Xu, X. Lv and J. Chen,
et al., DFT investigation of capacious, ultrafast and highly conductive hexagonal Cr2C and V2C monolayers as anode materials for high-performance lithium-ion batteries, Phys. Chem. Chem. Phys., 2017, 19, 7807–7819 RSC
.
- Y. Jing, Z. Zhou, C. Cabrera and Z. Chen, Metallic VS2 Monolayer: A Promising 2D Anode Material for Lithium Ion Batteries, J. Phys. Chem. C, 2013, 117, 25409–25413 CrossRef CAS
.
- R. Bhandavat, L. David and G. Singh, Synthesis of Surface-Functionalized WS2 Nanosheets and Performance as Li-Ion Battery Anodes, J. Phys. Chem. Lett., 2012, 3, 1523–1530 CrossRef CAS
.
- P. He, M. Yan, G. Zhang, R. Sun, L. Chen and Q. An, Layered VS2 Nanosheet-Based Aqueous Zn Ion Battery Cathode, Adv. Energy Mater., 2017, 7, 1601920 CrossRef
.
- S. Deng, L. Wang, T. Hou and Y. Li, Two-Dimensional MnO2 as a Better Cathode Material for Lithium Ion Batteries, J. Phys. Chem. C, 2015, 119, 28783–28788 CrossRef CAS
.
- M. O. Guler, O. Cevher and T. Cetinkaya,
et al., High capacity TiO2 anode materials for Li-ion batteries, Energy Convers. Manage., 2013, 111–116 CrossRef CAS
.
- A. Eftekhari, Potassium secondary cell based on prussian blue cathode, J. Power Sources, 2004, 126, 221–228 CrossRef CAS
.
- T. Ichitsubo, T. Adachi, S. Yagi and T. Doi, Potential positive electrodes for high-voltage magnesium-ion batteries, J. Mater. Chem., 2011, 21, 11764–11772 RSC
.
- C. D. Wessells, S. V. Peddada, R. A. Huggins and Y. Cui, Nickel hexacyanoferrate nanoparticle electrodes for aqueous sodium and potassium ion batteries, Nano Lett., 2011, 11, 5421–5425 CrossRef CAS
.
- S. Liu, J. J. Hu, N. F. Yan, G. L. Pan, G. R. Li and X. P. Gao, Aluminum storage behavior of anatase TiO2 nanotube arrays in aqueous solution for aluminum ion batteries, Energy Environ. Sci., 2012, 5, 9743–9746 RSC
.
- M. D. Slater, D. Kim, E. Lee and C. S. Johnson, Sodium-ion batteries, Adv. Funct. Mater., 2013, 23, 947–958 CrossRef CAS
.
- N. Singh, T. S. Arthur, C. Ling, M. Matsui and F. Mizuno, A high energy-density tin anode for rechargeable magnesium-ion batteries, Chem. Commun., 2013, 49, 149–151 RSC
.
- D. Datta, J. Li and V. B. Shenoy, Defective graphene as a high-capacity anode material for Na- and Ca-ion batteries, ACS Appl. Mater. Interfaces, 2014, 6, 1788–1795 CrossRef CAS
.
- J. Gullman and O. Olofsson, The crystal structure of SnP3 and a note on the crystal structure of GeP3, J. Solid State Chem., 1972, 5, 441–445 CrossRef CAS
.
- L. Haggstrom, J. Gullman and T. Ericsson,
et al., Mössbauer study of tin phosphides, J. Solid State Chem., 1975, 13, 204–207 CrossRef CAS
.
- P. C. Donohue and H. S. Young, Synthesis, structure, and superconductivity of new high pressure phases in the systems GeP and GeAs, J. Solid State Chem., 1970, 1, 143–149 CrossRef CAS
.
- S. Sun, F. Meng, H. Wang and Y. Ni, Novel two-dimensional semiconductor SnP3: high stability, tunable bandgaps and high carrier mobility explored using first-principles calculations, J. Mater. Chem. A, 2018, 6, 11890–11897 RSC
.
- Y. Jing, Y. Ma, Y. Li and T. Heine, GeP3: A Small Indirect Band Gap 2D Crystal with High Carrier Mobility and Strong Interlayer Quantum
Confinement, Nano Lett., 2017, 17, 1833–1838 CrossRef CAS
.
- L. Feng, A. Li, P. Wang and Z. T. Liu, Novel Two-Dimensional Semiconductor SnP3 with High Carrier Mobility, Good Light Absorption, and Strong Interlayer Quantum Confinement, J. Phys. Chem. C, 2018, 122, 24359–24367 CrossRef CAS
.
- B. Ghosh, S. Puri, A. Agarwal and S. Bhowmick, SnP3: A Previously Unexplored Two-Dimensional Material, J. Phys. Chem. C, 2018, 122, 18185–18191 CrossRef CAS
.
- M. S. Ramzan, V. Bacic, Y. Jing and A. Kuc, Electronic Properties of a New Family of Layered Materials from Groups 14 and 15: First-Principles Simulations, J. Phys. Chem. C, 2019, 123, 25470–25476 CrossRef CAS
.
- M. Kar, R. Sarkar, S. Pal and P. Sarkar, Two-dimensional CP3 monolayer and its fluorinated derivative with promising electronic and optical properties: A theoretical study, Phys. Rev. B, 2020, 101, 195305 CrossRef CAS
.
- G. Kresse and J. Furthmuller, Efficient Iterative Schemes for Ab Initio Total-Energy Calculations Using a Plane-Wave Basis Set, Phys. Rev. B: Condens. Matter Mater. Phys., 1996, 54, 11169 CrossRef CAS
.
- G. Kresse and D. Joubert, From Ultrasoft Pseudopotentials to the Projector Augmented-Wave Method, Phys. Rev. B: Condens. Matter Mater. Phys., 1999, 59, 1758–1775 CrossRef CAS
.
- J. P. Perdew, K. Burke and Y. Wang, Generalized Gradient Approximation for the Exchange-Correlation Hole of a Many-Electron System, Phys. Rev. B: Condens. Matter Mater. Phys., 1996, 54, 16533–16539 CrossRef CAS
.
- J. P. Perdew, K. Burke and M. Ernzerhof, Generalized Gradient Approximation Made Simple, Phys. Rev. Lett., 1996, 77, 3865–3868 CrossRef CAS
.
- S. Grimme, Semiempirical GGA-Type Density Functional Constructed with a Long-Range Dispersion Correction, J. Comput. Chem., 2006, 27, 1787–1799 CrossRef CAS
.
- A. Togo, F. Oba and I. Tanaka, First-principles calculations of the ferroelastic transition between rutile-type and CaCl2-type SiO2 at high pressures, Phys. Rev. B: Condens. Matter Mater. Phys., 2008, 78, 134106 CrossRef
.
- X. Gonze and C. Y. Lee, Dynamical matrices, Born effective charges, dielectric permittivity tensors, and interatomic force constants from density-functional perturbation theory, Phys. Rev. B: Condens. Matter Mater. Phys., 1997, 55, 10355–10368 CrossRef CAS
.
- G. Henkelman, B. P. Uberuaga and H. Jónsson, A Climbing Image Nudged Elastic Band Method for Finding Saddle Points and Minimum Energy Paths, J. Chem. Phys., 2000, 113, 9901 CrossRef CAS
.
- G. Henkelman and H. Jónsson, Improved Tangent Estimate in the Nudged Elastic Band Method for Finding Minimum Energy Paths and Saddle Points, J. Chem. Phys., 2000, 113, 9978 CrossRef CAS
.
- S. Zhao, Z. Li and J. Yang,
et al., Obtaining Two-Dimensional Electron Gas in Free Space without Resorting to Electron Doping: An Electride Based Design, J. Am. Chem. Soc., 2014, 136(38), 13313–13318 CrossRef CAS
.
- N. Miao, B. Xu, N. C. Bristowe, J. Zhou and Z. Sun, Tunable Magnetism and Extraordinary Sunlight Absorbance in Indium Triphosphide Monolayer, J. Am. Chem. Soc., 2017, 139(32), 11125–11131 CrossRef CAS
.
- F. Li, X. Liu, Y. Wang and Y. Li, Germanium monosulfide monolayer: a novel two-dimensional semiconductor with a high carrier mobility, J. Mater. Chem. C, 2016, 4(11), 2155–2159 RSC
.
- W. Tang, E. Sanville and G. Henkelman, A Grid-Based Bader Analysis Algorithm without Lattice Bias, J. Phys.: Condens. Matter, 2009, 21, 084204 CrossRef CAS
.
- Q. Li, C. Duan and X. Wan,
et al., Theoretical Prediction of Anode Materials in Li-Ion Batteries on Layered Black and Blue Phosphorus, J. Phys. Chem. C, 2015, 119, 8662–8670 CrossRef CAS
.
- S. Mukherjee, L. Kavalsky and C. V. Singh, Ultrahigh storage and fast diffusion of Na and K in blue phosphorene anodes, ACS Appl. Mater. Interfaces, 2018, 10, 8630–8639 CrossRef CAS
.
- X. M. Zhang, W. Z. Meng and T. L. He,
et al., Mn2C monolayer: a superior anode material offering good conductivity, high storage capacity and ultrafast ion diffusion for Li-ion and Na-ion batteries, Appl. Surf. Sci., 2020, 503, 144091 CrossRef CAS
.
- X. M. Zhang, L. Jin, X. F. Dai, G. F. Chen and G. D. Liu, Two-dimensional GaN: an excellent electrode material providing fast ion diffusion and high storage capacity for Li-ion and Na-ion batteries, ACS Appl. Mater. Interfaces, 2018, 10, 38978–38984 CrossRef CAS
.
- Z. Z. Zhang, Y. F. Zhang, Y. Li, J. Lin, D. Truhlar and S. P. Hua, MnSb2S4 monolayer as an anode material for metal-ion batteries, Chem. Mater., 2018, 30, 3208–3214 CrossRef CAS
.
- X. Deng, X. Chen and Y. Huang,
et al., Two-dimensional GeP3 as a high capacity anode material for non-lithium-ion batteries, J. Phys. Chem. C, 2019, 123, 4721–4728 CrossRef CAS
.
- C. M. Zhang, Y. L. Jiao, T. W. He, F. X. Ma, L. Z. Kou, T. Liao, S. Bottle and A. J. Du, Two-dimensional GeP3 as a high capacity electrode material for Li-ion batteries, Phys. Chem. Chem. Phys., 2017, 19, 25886–25890 RSC
.
- C. S. Liu, X. L. Yang, J. Liu and X. J. Ye, Theoretical Prediction of Two-Dimensional SnP3 as a Promising Anode Material for Na-Ion Batteries, ACS Appl. Energy Mater., 2018, 1, 3850–3859 CrossRef CAS
.
- Z. Y. Zhao, Y. Tong, S. T. Zhang, H. Y. Xu, G. C. Yang and Y. C. Liu, Metallic P3C monolayer as anode for sodium-ion batteries, J. Mater. Chem. A, 2019, 7, 405–411 RSC
.
Footnote |
† Electronic supplementary information (ESI) available. See DOI: 10.1039/d0na00746c |
|
This journal is © The Royal Society of Chemistry 2020 |
Click here to see how this site uses Cookies. View our privacy policy here.