DOI:
10.1039/C9NR08187A
(Paper)
Nanoscale, 2020,
12, 155-166
Amplified light harvesting for enhancing Italian lettuce photosynthesis using water soluble silicon quantum dots as artificial antennas†
Received
23rd September 2019
, Accepted 17th November 2019
First published on 18th November 2019
Abstract
Maximizing the utilization of the ultraviolet portion in the solar radiation spectrum has always been a goal for plant growth in order to minimize the harm and harvest the benefits. For this reason, fluorescent, amine-functionalized, and water-soluble silicon quantum dots (SiQDs) were fabricated with a mono-dispersed size of 2.4 nm. The SiQDs were used as artificial antennas to amplify the light harvesting ability and consequently enhance the photosynthesis in Italian lettuce. Upon ultraviolet excitation, the intense blue emission from the quantum dots matched very well to the absorption of the chloroplasts, allowing for the ultraviolet portion in solar radiation to be effectively utilized. The consumed optical energy enhanced the photosystem II activity, which made the amplification of photosynthesis possible. More importantly, in vivo, the quantum dots significantly promoted Italian lettuce seedling growth at concentrations below 30 mg L−1 on the root length, seedling height, and biomass by increasing the soluble sugar and water content by 49.8% and 40.9%, respectively. Interestingly, the chlorophyll a and b content increased to 41.0 and 114.8%, respectively, with no inhibition, even at the highest dose of 200 mg L−1. This study provides a new perspective on the use of quantum dots to amplify the utilization of ultraviolet light in agriculture.
1. Introduction
Solar energy is one of the most sustainable sources of energy on earth. In natural photosynthesis, plants absorb light energy as well as assimilate carbon dioxide and water to provide organic matter and energy for plant growth and development. However, in plant photosynthesis, chloroplasts as important organelles in plant photosynthesis are limited to absorbing only a specific wavelength of light. Consequently, this results in only less than 10% of sunlight being utilized for photosynthesis.1 Improving the solar energy utilization in photosynthesis is critical to improve plant yields.
Photosynthesis is an extremely complex biochemical process, including a light reaction and dark reaction. In the light reaction process, water molecules driven by light are oxidized to release electrons, which are transferred through the electron transport chain similar to mitochondria, generating energy (ATP) and reducing force (NADPH), thus promoting the carbon assimilation of the dark reaction. Therefore, the light reaction (photosystem I and photosystem II) plays a decisive role in the final effective photosynthesis since the light reaction is the beginning of the whole photosynthesis.2 To effectively improve the plant photosynthesis process, researchers in different fields have designed various strategies and made unremitting efforts.3–5
Bioengineering technology is a common method used to improve the utilization and conversion efficiency of light energy in photoreactions. Li's group established an isopropanol biosynthesis pathway that consumed only NADPH by introducing NADPH-dependent dehydrogenase with cyanobacteria as the research model. This increased the cell growth rate, cell activity, and sunlight conversion efficiency, thereby increasing the photosynthetic efficiency by about 50%.6 In 2016, Kromdijk et al. injected specific genes into tobacco, and the protein expressed by these genes accelerated the shutdown of the non-photochemical quenching of the chlorophyll fluorescence and improved the light saturation and light damage threshold of the plants to sunlight. This, therefore, improved the light harvesting capacity of the chloroplast.7 However, the shortcomings of these biological photosynthetic systems are also obvious. The mismatch between the chlorophyll absorption spectrum and solar spectrum resulted in the conversion of solar energy not being realized to the maximum extent. The photosynthetic efficiency of most plants is 0.1%, and the highest is no more than 6%.
According to the principle of photosynthesis, improving the ability of the chloroplast to harvest and utilize sunlight is another important way to improve the efficiency of chlorophyll photosynthesis.8 The specific light conversion feature of luminescent materials provides unique advantages for their application as light harvesting agents to enhance plant photosynthesis. In recent years, with the rapid development of nano-luminescent materials with controllable preparation technologies, good luminescence properties, and biocompatibility, they have been used as artificial antennas, such as organic fluorescent dyes, quantum dots, transition metal complexes, and rare earth doped upconversion luminescent nanomaterials, for the light harvesting of plant photosynthesis. These nano-luminescent materials convert ultraviolet, yellow-green, or near-infrared light, which cannot be absorbed and utilized by chlorophyll itself, into light that can be effectively absorbed by chlorophyll, thereby improving the conversion efficiency of the chloroplasts to sunlight.9–11 However, different materials have their own features and advantages. Traditional organic dyes and metal quantum dots have obvious disadvantages such as high cost, complex preparation process, poor water solubility and light stability, and high biological toxicity. UCNPs often have better light stability than organic dyes, higher penetration depth, less damage to biological tissues etc. However, there are some deficiencies in the application of plant imaging such as a large particle size, difficulty in large-scale synthesis, and an insufficient excitation penetration depth,12 which limits its biological application in plants. In Wang and Liu's group, polymer nanoparticles were used to coat the surface of chloroplasts to absorb a wider spectrum energy,13 and the results showed that conjugated polymer nanomaterials were helpful in accelerating the electron transfer speed of photosystem II. Similar results were also obtained in the single-walled carbon nanotube composite chlorophyll assembly system. After the chloroplasts were assembled with single-walled carbon nanotubes, the electron transfer rate in the leaves increased by threefold compared with the control group, effectively improving the photosynthetic efficiency.14 Igor Nabiev et al. first reported a hybrid system of artificial photosynthesis using representative CdTe quantum dots via a resonance energy transfer between the quantum dots and phytochrome.4 This hybrid system increased the excitation efficiency about three times, thus illustrating that the hybrid quantum dot system, as an “antenna effect”, effectively improved the efficiency of chlorophyll photosynthesis.3 Subsequently, Hyunwoong Park et al. further demonstrated that the CdS/(Cu-NaxH2−xTi3O7) ternary composite, as electron donors, could be used as a catalyst for the photoreduction of CO2 in the presence of water to construct photosynthetic composite materials.15 However, the light harvesting quantum dots reported so far are limited to studying the enhanced photosynthetic effect of chlorophyll in vitro. No studies in literature have reported the effect and mechanism of increasing the yield in vivo. With the intensification of the global greenhouse effect, the ozone layer in the atmosphere has gradually become thinner, and the intensity of ultraviolet radiation continues to increase. Therefore, it is ideal to develop fluorescent quantum dots that can absorb ultraviolet light and emit blue light to improve the photosynthesis of plants in vitro and in vivo as well as minimize the harm.
In contrast, silicon quantum dots (SiQDs) have distinctive merits in the study of plant photosynthesis such as good water-solubility and biocompatibility, low biotoxicity, simple preparation process, excellent luminescence performance, and adjustable fluorescence/phosphorescence performance. Notably, it has also been reported that porous Si nanoparticles can be biodegraded in vivo to produce silicic acid, which is biocompatible with many tissues and is easily cleared via renal clearance in mice.16 In addition, a class of SiQDs possessed unique optical properties of (1) tunable luminescence from near-UV to near-IR due to their controllable size and functional groups and (2) strong ultraviolet absorption and intense emission due to their quantum confinement.17–19 Thus, the optical tunable feature of SiQDs makes it possible to fabricate the quantum dots with a matched emission of the chloroplasts to improve the photosynthetic capacity.20 On the other hand, the UV portion of solar energy cannot be absorbed by plant pigments and has an adverse effect on plant growth.21 A good approach is to deliver the SiQDs to the plants and then convert the UV light to a chloroplast absorbable color to enhance the plant photosynthesis and, at the same time, mitigate UV abiotic stressors as illustrated in Scheme 1. Thus, as excellent light harvesting materials, SiQDs have the potential to promote the photosynthesis of plants in vivo. In the present work, water-soluble SiQDs with a high quantum yield were synthesized, and were found to be beneficial in accelerating the electron transfer rate. This application of silicon nanomaterials in plant photosynthesis has an important academic value.
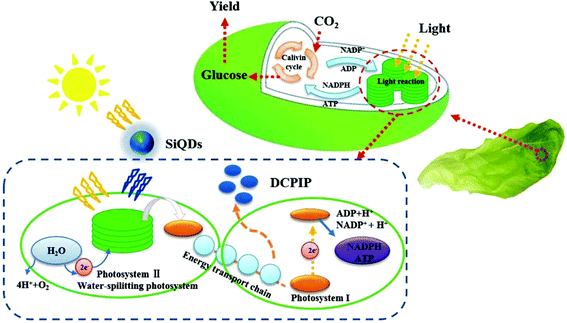 |
| Scheme 1 Illustration of the mechanisms of the SiQDs augmenting the light harvesting ability of chloroplasts in photosynthesis. | |
2. Experimental
2.1 Synthesis of SiQDs
The fluorescent SiQDs were synthesized by a one-step hydrothermal method modified from a microwave preparation.22 In a representative preparation of the 300 mL precursor solution, 11.16 g of trisodium citrate dehydrate was dissolved in 240 mL of deionized (DI) water. After 20 min of stirring, 60 mL of N-[3(trimethoxysilyl)propyl]ethylenediamine (DAMO) was added to the previous solution and continually stirred for 40 min. To remove the dissolved oxygen, the mixed solution was bubbled with nitrogen gas. The precursor solution was then transferred to a Teflon-lined stainless-steel autoclave and heated at 200 °C for 12 h. After cooling to room temperature naturally, the SiQDs were purified by dialysis, where residual reagents (MWCO 1 kDa) were removed in 10× DI water for 20 h. Finally, the SiQDs were lyophilized.
2.2 Preparation of chloroplast/SiQDs complexes for hill reaction
The chloroplasts of Italian lettuce (Lactucasativa L. var. ramosa Hort.) were isolated according to Li's work.1 The chloroplast suspension was adjusted in sucrose buffer to a 5 mg L−1 target concentration as determined by its absorption at 652 nm in a mixed solution of 80% acetone. Lyophilized SiQDs were added to the chloroplast solution at various concentrations (0.3, 3, 30, 100, and 200 mg L−1) to form the chloroplast/SiQDs complexes. The Hill reaction is a classical method used to measure photosynthetic activities of chloroplast under light irradiation. Briefly, the reaction was initiated by adding 2,6-dichlorophenolindophenol (DCPIP) (60 mM) under light irradiation. The reaction kinetics were studied by monitoring the solution absorption changes at 600 nm for 0, 1, 2, 3, 4, and 5 min. Five replications were completed for each group and tested under both visible lamp and UV light.
2.3 Cultivation of Italian lettuce
Italian lettuce (Lactucasativa L. var. ramosa Hort.) was chosen as the test model. The seeds were randomly selected and germinated in square Petri dishes (13 mm × 13 mm) containing filter paper soaked in DI water under a weak light environment for 2 days. The immature embryos were transferred into planting cups with sponge blocks and cultured for 7 days in DI water. Then, the seedlings were cultivated in a nutrient solution on cultivating shelves. The nutrient solution was composed of A (472 mg L−1 Ca(NO3)2·4H2O and 101 mg L−1 KNO3) and B (101 mg L−1 KNO3, 80 mg L−1 (NH4)NO3, 100 mg L−1 KH2PO4, 174 mg L−1 K2SO4, and 246 mg L−1 MgSO4) with a 1
:
1 volume ratio. Before being treated with the SiQD solution, the seedlings continued to grow in the nutrient solution for 7 days.
2.4 SiQD application and seedling evaluations
The lyophilized SiQDs were dissolved in 20 L of lettuce-growth nutrient medium at concentrations of 0.3, 3, 30, 100, and 200 mg L−1. The control was a 20 L nutrient solution. The pH of all solutions was adjusted from 6.5 to 7.0 with H3PO4 and the electrical conductivities were 1.55–1.60 ms cm−1. Uniform Italian lettuce seedlings were selected and randomly arranged in cultivating shelves equipped with nutrient mediums at different concentrations of SiQDs. There were four replications for each treatment and six seedlings for each replication. All seedlings were cultured at 25 °C during the daytime (14 h) and 20 °C at night (10 h) with a relative humidity of 60% under room light (180 μmol m−2 s−1) plus a 2-hour daily UV irradiation (365 nm, 600 μW cm−2). The seedlings were harvested for further analyses on day 15. The growth parameters for the root lengths, seedling heights, and fresh weights were determined in the morphogenesis study. To explore the mechanisms for the effects from the SiQDs, chlorophyll a and b, water, and soluble sugar were evaluated by the methods described in the information section of this paper. Superoxide dismutase (SOD) and malondialdehyde (MDA) were determined to predict the abiotic stressors using commercial assay kits. Finally, the uptake and distribution of the SiQDs in the seedlings were demonstrated by light sheet microscopy (LSM) images utilizing SiQDs’ fluorescence features. In detail, the plant tissue was sliced by hand and placed on a clean glass slide. The autofluorescence signal was reduced by setting the detection parameters of the confocal laser scanning microscope. No fluorescence was observed in the control group. Under the same conditions, SiQD-treated samples emitted a strong red fluorescence signal.
3. Results and discussion
3.1 Physical and chemical properties
In the present work, a hydrothermal reaction used to synthesize the water soluble SiQDs was developed using DAMO as silane molecules and trisodium citrate as a stabilizer/reducer. DAMO has the structure shown in ESI Fig. S1,† with three methoxy groups on the Si end, a primary amine on the opposite end, and one secondary amine in between them. In aqueous media, the methoxy groups were rapidly hydrolyzed, but the C–Si bond was stable so that the aminopropyl group was still contacted.23 The resulting silanol underwent a self-condensation with the other silanols to initiate the formation of the SiQDs. Trisodium citrate, however, functioned as a stabilizer/reducer to regulate the size of the SiQDs.22 In contrast to previous findings,24 at our reaction conditions (200 °C for 12 hours), the C–Si bonds were stable without any cleaving. As a result, the amines decorated the SiQDs on the surface. The concentration of the as-prepared SiQDs was up to 26 g L−1, which was higher than that previously reported (10 g L−1).25
In the transmission electron microscopy (TEM) image (Fig. 1a), the SiQDs appeared as spherical particles with a good monodispersity and uniformity. The size distribution showed an average diameter of 2.4 nm (Fig. 1b). The high-resolution TEM image of the SiQDs in Fig. 1c indicated a uniform lattice plane spacing of 0.28 nm, demonstrating a highly ordered arrangement. The X-ray diffraction (XRD) pattern in Fig. 1d displayed a broad Si peak at 21° (2θ), indicating that the Si atoms were disordered.26
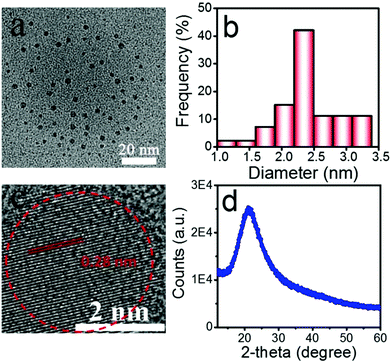 |
| Fig. 1 Morphology of the SiQDs by TEM images (a). Size distribution calculated by measuring the height of more than 150 particles (b). High-resolution TEM image (c). XRD pattern (d). | |
To confirm the various functional groups, we performed Fourier-transform infrared (FTIR) absorption spectroscopy (Fig. 2a). The two orange ovals were the amine-related peaks. The broad absorption band between 3000 and 3600 cm−1 was attributed to the stretching vibration of N–H, which could be interpreted as a mixture of unresolved feature peaks of primary and secondary amines. The bending vibration of N–H at 1576 cm−1 further confirmed the existence of the amine groups.25 The two cyan ovals were the absorption of the C–H stretching (2850–3000 cm−1) and bending (1471 cm−1) modes.27 The peak at 1650 cm−1 circled by a black oval was assigned to the bending vibration of C
O.27 The magenta oval-labelled band between 1010 cm−1 and 1160 cm−1 resulted from the stretching vibration of Si–O.27 The FTIR data confirmed that the SiQDs consisted of NH, CH, CO, and SiO groups. The NH groups existed in the SiQDs, indicating that the NH group attached on the surface of the silicon core, which was understood from the previously discussed SiQD synthesis mechanism.23,27 The advantages of these amine-functionalized surfaces have been discussed previously.28 In this work, the biocompatible feature was important to enhance the water dispersibility of the quantum dots, which made it possible to apply the SiQDs in a situation when a solution was needed.
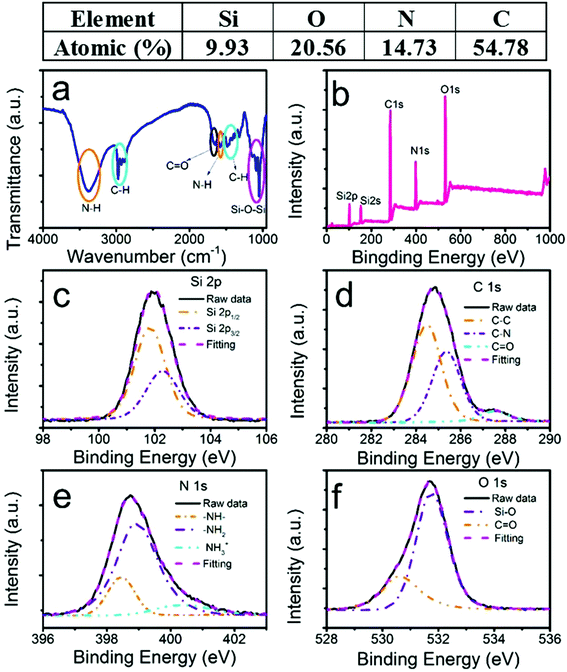 |
| Fig. 2 Characteristics of the SiQDs. FTIR spectrum of lyophilized SiQDs (a). XPS survey spectrum (b). High-resolution XPS spectra of Si 2p (c), C 1s (d), N 1s (e), and O 1s (f). For the high-resolution XPS (c–f), the solid black and magenta dashed curves are the raw data and curve fitting lines, respectively. The dashed lines are deconvoluted peaks. | |
To confirm the chemical composition, an X-ray photoelectron spectroscopy (XPS) survey spectrum (Fig. 2b) showed the elemental peaks of Si, O, N, and C. The atomic ratios were 9.93%, 20.56%, 14.73%, and 54.78%, respectively. The result was consistent with our FTIR results. No Na element was detected, which was different from a previous report.22 Moreover, Fig. 2c–f depict the high-resolution XPS (HRXPS) spectra of those elements. In Fig. 2c (Si 2p), a nearly symmetric emission at 102 eV was identified as organic Si,28 which shifted to a lower energy compared with the reported Si–O–Si.19 The sum of the two deconvoluted peaks from the Si 2p1/2 and Si 2p3/2 energy levels at 101.83 and 102.28 eV, respectively, excellently fit the raw data. The energy difference between them (0.45 eV) and the intensity ratio (0.51) of I (2p1/2) to I (2p2/3) agreed well with the reported values by D. Pleul.29 As expected, the peak position shifting to a lower energy from 104 eV in the DAMO raw material to 102 eV in our SiQDs indicated the formation of the Si–O–Si bond from Si–O–H as the binding energy decreased.24 However, the Si–O–Si–C composition was not resolvable from Si–O–Si due to the broadening of the Si 2p peak in the SiQDs.24 In contrast to a previous work, we did not detect any Si at the lower oxygen states, indicating that trisodium citrate could function as a stabilizer only.23,24 The XPS spectrum of C 1s (Fig. 2d) had three deconvoluted peaks at 284.46 (55.5%), 285.36 (38.8%), and 287.53 eV (5.7%), which were assigned to C–C, C–N, and C
O, respectively, according to an appropriate binding energy order of C–C < C–N < C
O. The existence of the small percentage of C
O demonstrated that the surface amines may have interacted with the carboxyl acid on citrate to form small amounts of amides. In Fig. 2e, the HRXPS spectrum of N 1s again deconvoluted three emissions at 398.5 (17%), 398.9 (71.2%), and 400.3 eV (10.8%), representing the secondary, primary, and protonated amines, respectively.30 The N 1s HRXPS indicated that H2N(CH2)2NH(CH2)3Si was still linked together in our synthesis conditions. More specifically, the C–Si bond did not undergo cleavage, which led to the formation of amine-functionalized SiQDs.24 Two peaks at 530.6 (26.6%) and 531.7 eV (73.4%) of the O 1s HRXPS spectrum (Fig. 2e) were due to the C
O and Si–O bonds,31 respectively. Again, the C
O peak was from a small percent of amides.
3.2 Optical properties
Fig. 3a displays the ultraviolet-visible absorption between 300 and 500 nm with a maximum at around 360 nm. Compared to the DAMO Si nanoparticles reported by F. Wu et al.,22 our SiQDs had more than 5 times higher absorbance. The blue and magenta curves in Fig. 3b are the excitation and emission spectra of the photoluminescence (PL) of the SiQDs, respectively, showing the maximum emission peak at 445 nm upon excitation at 365 nm. The excitation spectrum when monitored at 445 nm emission had a similar profile as the absorption spectrum, indicating that the UV light could be effectively absorbed and converted to blue emission through photoluminescence (PL). The as-prepared SiQDs appeared to be a yellow solution that turned to a bright-blue color under UV irradiation (365 nm) (Fig. 3b inset, left and right, respectively). In order to further explore the fluorescence properties of the SiQDs, the excitation-dependent emission spectra were recorded under an excitation from 300 nm to 400 nm (Fig. 3c) with an increment of 20 nm. The result showed that the PL intensity increased first and then gradually decreased with an increase in the excitation wavelength. As the excitation wavelength changed, the emission peaks remained at the same position of 445 nm, indicating the good uniformity of the SiQDs in size and that they agreed well with our finding of the size distribution in Fig. 1b.31 The emission peak position being independent of the excitation wavelength revealed that the as-prepared SiQDs avoided autofluorescence during the application.32 Thus, they could be used as a fluorescence probe to monitor the transportation process and distribution within plant tissues without autofluorescence interference via a selective excitation technique. The relative PL quantum yield of the as-prepared SiQDs was determined to be 81% using quinine sulphate as a reference (Table S1†). The flower drawn using the SiQD solution further provided compelling evidence of a high PL quantum yield of SiQDs (Fig. 3d).
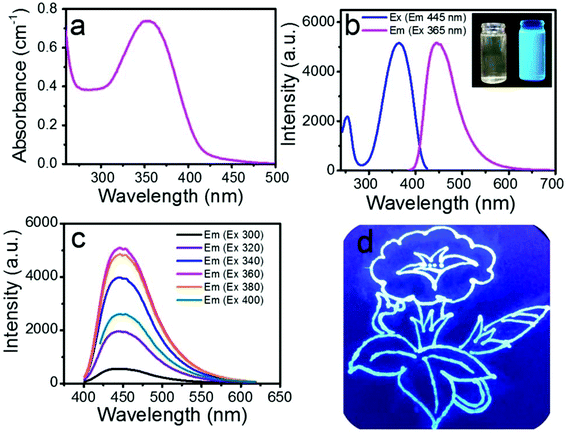 |
| Fig. 3 Optical properties of the SiQDs. Ultraviolet-visible absorption spectrum of a 1 mg mL−1 solution of SiQDs (a). PL excitation (blue) and emission (magenta) spectra (b). Inset photograph of the SiQD solutions taken under daylight and UV (365 nm) irradiation on the left and right, respectively (inset b). PL emission spectra of the SiQDs at different excitation wavelengths as indicated (c). Flowers drawn on the filter paper using the SiQD solution under UV (365 nm) irradiation (d). | |
3.3 Chloroplasts reabsorb blue emission from SiQDs
Chloroplasts are essential in the photosynthesis process used by plants and other organisms. Chloroplasts absorb blue and red emissions but no pronounced peaks appear in the UV region (Fig. 4a, blue). In order to harvest UV light, which is a part of the sunlight spectrum, we used the SiQDs’ broad UV absorption around 365 nm (Fig. 4a black curve) to convert to blue light emission around 445 nm (Fig. 3a red curve). After combining the SiQDs with the chloroplasts, the absorption spectrum of chloroplast/SiQD mixture had a strong UV absorption at around 360 nm (Fig. 4a, magenta). The Fig. 4b inset shows that the emission from the SiQDs (Fig. 3b) perfectly matches the absorption of the chloroplasts to make UV light harvesting possible. To examine if the chloroplasts reabsorbed the blue emission from the SiQDs, we collected a PL emission of the mixture under 365 nm excitation. When the chloroplasts were added to the SiQD solution, the PL intensity of the SiQDs denoted by the purple curve decreased in comparison to the blue curve in Fig. 4b, indicating that the chloroplasts absorbed the blue light emitted from the SiQDs. We estimated that the reabsorption consumed 45.07% of the blue emission from the SiQDs by counting the difference in the area under the curves with and without chloroplasts. The blue light reabsorption made unitizing UV energy by the chloroplasts possible. To prove this finding, we plotted the emission difference between the two curves and found that the profile of the missing blue emission (Fig. 4b, magenta curve) yielded a clear peak at 440 nm that matched well with the absorption of the chloroplasts at the same wavelength as shown in Fig. 4a.
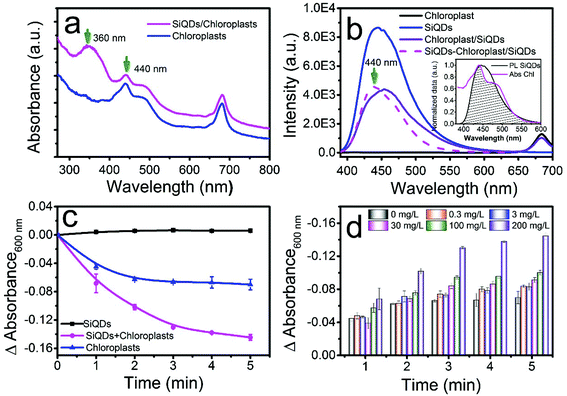 |
| Fig. 4 Utilizing UV light to accelerate the Hill reaction in vitro. Ultraviolet-visible absorption of the chloroplast (blue) and chloroplast/SiQDs (magenta) mixture (a). PL emission spectra of the SiQDs alone (blue), chloroplast/SiQDs mixture (purple), and chloroplasts alone (black) after excitation at 365 nm. The emission difference profile (magenta) by subtracting the emission spectrum of the mixture from that of the SiQDs alone (b). Changes in the absorption of DCPIP at 600 nm during the Hill reaction of the SiQDs alone (black), chloroplasts alone (blue), and chloroplast/SiQDs (5 mg L−1/200 mg L−1) mixture (magenta) (c). Absorption changes for DCPIP at 600 nm during the Hill reaction when mixed with various concentrations of the SiQDs at a fixed chloroplast concentration of 5 mg L−1 (d). | |
3.4 Electron transferring through light dependent photosynthesis in vitro
To prove our assumption of utilizing UV energy reabsorption by the chloroplasts through the SiQDs, the Hill reaction was performed with and without the SiQDs. In a set of Hill reactions using the isolated chloroplasts in the presence of different concentrations of SiQDs, we measured the absorption changes of DCPIP, a terminal electron acceptor, at 600 nm after xenon lamp irradiation (6 mW cm−2). The light intensity from the xenon lamp (320–780 nm) was approximately 45% of the photosynthesis saturation.33 The absorption decreasing with time was associated with the rate of the formation of reduced DCPID when capturing the electrons released from water by photosystem II in a chloroplast photoreaction. Therefore, the DCPIP reduction assay was developed as a common method to measure the photosystem II activity.1 In our study, the absorption changed with time in the mixture solution of DCPIP, chloroplasts with/without SiQDs, as well as SiQDs alone as shown in Fig. 4c. The chloroplast mixtures with various SiQD concentrations are displayed in Fig. 4d. The static curve from the solution containing only SiQDs (Fig. 4c, black line) meant that the quantum dots did not interfere with the reaction. However, the decreases in the absorption of both the chloroplast and chloroplast/SiQD solutions suggested the occurrence of a photosynthetic reaction. The rapid decrease in the DCPIP absorption in the latter indicated that the SiQDs accelerated the electron transfer process. Fig. 4d shows the SiQD dose-dependent effect pattern on the rate of the Hill reaction. As the concentration of the SiQDs increased, the reduction rate of DCPIP was gradually enhanced and reaches a maximum at the concentration of 200 mg L−1, indicating that the SiQDs had the ability to promote the photosynthetic activity of the chloroplast by enhancing light-harvesting. To further prove the light-harvesting hypothesis of the SiQDs, Hill reactions were also performed under UV lamp irradiation alone (365 nm, 600 μW cm−2). After illumination, the DCPIP reduction assays were performed with the chloroplasts, chloroplast/SiQD mixture, and SiQDs alone. The fact that the DCPIP absorption decreased faster with time in the chloroplast/SiQD solution compared to that in the chloroplasts alone (Fig. S2†) signalled that the SiQDs, indeed, accelerated the electron-transferring process in the photosynthesis reaction under UV irradiation in vitro. However, the SiQD control alone did not participate in the reaction under UV. From the above data, we concluded that the SiQDs enhanced the photosynthetic activity of the chloroplasts in vitro due to the reabsorption of the chloroplast by the SiQD emission.
3.5 Effects of SiQDs on Italian lettuce seedling growth in vivo
3.5.1 Effects on seedling morphogenesis.
To study the effects of the SiQD treatment in vivo, we monitored the growth progress of the Italian lettuce seedlings under regular daylight plus two hours of daily UV at 365 nm. The lettuce seedlings were cultivated with a growth medium containing the SiQDs at different concentrations of 0, 0.3, 3, 30, 100, and 200 mg L−1. The evaluation was conducted on day 15. Fig. 5 displays pictures of a representative Italian lettuce from each treatment group, illustrating dose-dependent effects on the growth. As the SiQD concentration increased, the leaves became greener and differences in the morphological features were observed. Quantitatively, the growth parameters of the root length, seedling height, and fresh weight were measured. As shown in Fig. 6, the addition of the SiQDs in the nutrient medium had significantly positive dose-dependent effects on all three parameters up to 30 mg L−1, but inhibits the growth at a concentration higher than 100 mg L−1. The root length (Fig. 6a), seedling height (Fig. 6b), and fresh weight (Fig. 6c) reached a maxima at the concentration of 3 mg L−1, increasing by 27.2%, 14.1%, and 40.6%, respectively, compared with the controls. However, at a concentration of 200 mg L−1, all three parameters were lower than that in the control group, showing a paradox of abiotic stress at a high concentration of SiQDs. Our data suggested that the SiQDs had no phytotoxicity on the Italy lettuce seedlings and had the potential to stimulate plant growth within a limited concentration (<30 mg L−1).
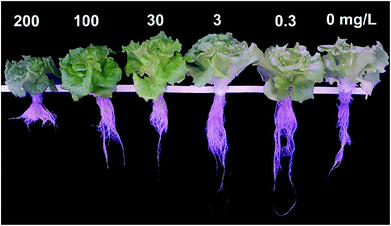 |
| Fig. 5 SiQD effects on the phenotype of Italian lettuce. The phenotype of the Italian lettuce seedlings treated with different concentrations of SiQD solution. | |
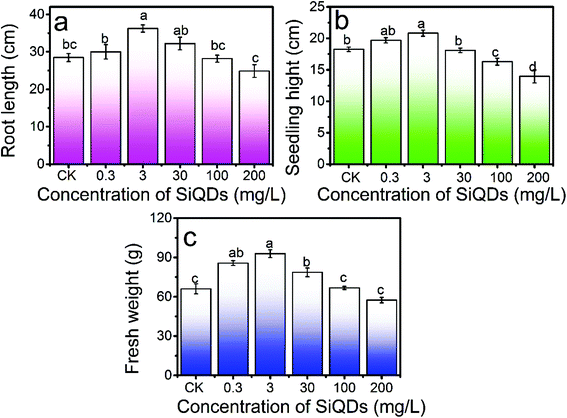 |
| Fig. 6 Dose dependent growth morphology. Root length (a), seedling height (b), and fresh weight (c) of the Italian lettuce seedlings under the treatment of different concentrations of SiQDs. CK is the control group without SiQDs treatment. N = 4. Average ± SD. The different letters indicate the statistical difference among the groups (P < 0.05). | |
3.5.2 Chlorophyll content enhancement by SiQDs.
To understand seedlings becoming greener at higher SiQD concentrations, we explored the effect of the SiQDs on chlorophyll synthesis by measuring the chlorophyll a and b content at day 15 after the SiQD treatment. Chlorophyll, as the main pigment of plants in photosynthesis, plays a central role in light absorption and conversion into chemical energy. Higher concentrations of chlorophyll could make seedlings absorb more light and produce more energy and accordingly more soluble sugar during photosynthesis. As shown in Fig. 7a and b, the content constantly increased as the SiQD concentration increased with no inhibition appearing within the tested doses. At the highest SiQD concentration of 200 mg L−1, the chlorophyll a and chlorophyll b content reached the highest and increased by 41.04% and 114.83%, respectively, compared with the control group. Obviously, the SiQDs greatly promoted both, but much more of chlorophyll b production. These enhancements were reported in a few publications in different plants treated with nanoparticles such as TiO2 nanoparticles where a significant increase in the synthesis of chlorophyll b was observed compared to chlorophyll a in broad bean plant leaves.34 Chlorophyll biosynthesis is complicated and involves assembling of binding protein assembling, which is not fully understood.35 More experimental results are needed to elucidate the mechanisms of the enhancement of chlorophyll by the treatment of SiQDs at molecular and genetic levels. The increase in the production of chlorophylls can accordingly enhance plant photosynthesis by harvesting more light, including UV, to promote the lettuce seedling growth. However, the continuous increase in the green pigments did not follow the pattern of the morphology changes observed in Fig. 6. Therefore, we examined the influence of the SiQD treatments on seedling's water and soluble sugar content.
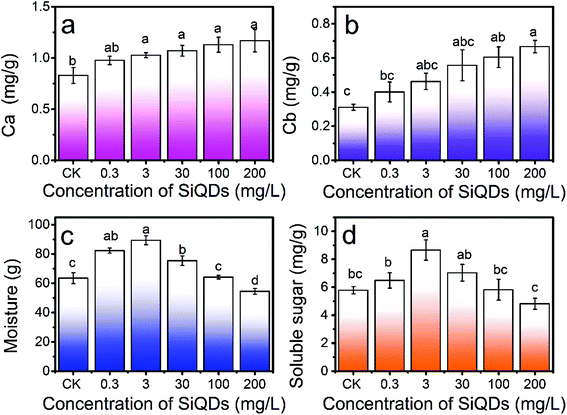 |
| Fig. 7 SiQDs effects on chlorophyll production, water content, and soluble sugar content. Dose-dependent effects on chlorophyll a (a), chlorophyll b (b), soluble sugar (c), and moisture (d) content of Italian lettuce seedlings under different concentrations of SiQDs treatments. CK is the control group without SiQDs treatment. N = 4. Average ± SD. The different letters indicate a significant difference among the different concentration treatments of the SiQD solution at the P < 0.05 level. | |
3.5.3 Effects on water and soluble sugar contents.
In a photosynthesis reaction, inside the chloroplast, water together with light absorbed by the chlorophylls produce energy to support the dark reaction in order to produce sugars. To test if the increased chlorophylls constantly produced soluble sugars, we examined the water content in the seedlings treated with different doses of the SiQDs first. The water content illustrated in Fig. 7c did not exhibit a constant increase in the SiQD concentrations. The highest water content indicated a 40.9% increase at 3 mg L−1 compared with that for the untreated seedlings. The beneficial effect may have been because the SiQDs could stimulate water uptake at a low dose as described in our previous work.36 However, the water content was below the baseline level at 200 mg L−1. Therefore, we tested the water uptake rate at this highest dose, which indicated a 27.4% rate attenuation as shown in Fig. S3.† Keeping in mind that water quantity and light quantity/quality together determine the energy generation in photosynthesis and consequently affect soluble sugar production, we analyzed the soluble sugar content in the seedling afterward. The result in Fig. 7d showed the same dose dependent trend as that observed for the water content. The produced sugar reached a maximum for the 3 mg L−1 dose with a 49.78% increase compared to that for the control group, but then decreased to below the baseline level at a 200 mg L−1 dose. The constantly increasing chlorophyll failed to constantly promote sugar production, which could have resulted from the attenuation of the water uptake at a high dose. Therefore, we hypothesized that the high concentration of the SiQDs may have induced plant abiotic stress to generate reactive oxygen species (ROS), thereby inhibiting the absorption of water and nutrients by roots and inhibiting plant photosynthesis.
With all the synergies together, the SiQDs significantly promoted lettuce seedling growth at a dose less than 30 mg L−1. However, at concentrations higher than 100 mg L−1, all three morphogenesis quantities were inhibited, showing a paradox of abiotic stress. The SiQDs (200 mg L−1) significantly reduced the moisture content in the seedlings too. The paradox was similar to a previous report in which multiwall carbon nanotubes promoted cell growth at low concentrations (5 μg mL−1) while significantly inhibiting cellular growth at higher concentrations (100–500 μg mL−1).37 We believe that the roots were a key factor for this paradox in the morphogenesis behavior. The excess of the SiQDs could have applied abiotic stressors to cause a decrease in the root growth. Extensive studies were conducted in the past decade on Ag-NPs, ZnO, TiO2, etc. in various crops, indicating that they could apply beneficial and malignant effects to plants, especially on the roots.38 Generally, roots are very sensitive to the surrounding environment and play an important role in plant growth since they directly connect to water and essential nutrients during the growth and development period. We observed that as the root shortened, water uptake was inhibited and water content in the seedlings decreased at a high concentration of the SiQDs, which could have been the cause of the inhibition. The insufficient water uptake could have conversely inhibited the root cellular growth and led to a shortening of the root system. The constant increase in the chlorophyll failed to constantly promote sugar production, which could have resulted from the attenuation of the water uptake at high doses.
3.5.4 Abiotic stress effects on SOD and MDA generations.
Plant growth is an aerobic metabolism process, in which reactive oxygen species (ROS) are constantly being produced as by-products. They are harmful if the concentration is high, but beneficial at appropriate concentrations during the repairing process against abiotic stress.38 Plants have an antioxidant system to maintain the right balance between generating and removing ROS. Abiotic nanoparticles or quantum dots could cause mechanical stress on plants and perturb the balance at high concentrations. Therefore, the generation of ROS can sensitively reflect the impact of stress from the particles or dots. Normally, one can use the increased levels of antioxidant enzymes to relate the generation of ROS since ROS are unstable and hard to be detected. For example, superoxide radicals produced predominantly by the photo-reduction of oxygen during photosynthesis can be removed by SOD. Therefore, we examined the effects of the SiQDs on the SOD activity and MDA content, a sensitive marker for oxidative stress to evaluate ROS damage to the seedlings. The results in Fig. S4a† revealed that the SOD activity increased gradually with the increase in the SiQD concentrations. Notably, the activity was significantly elevated when the concentration was higher than 100 mg L−1. Thus, it was concluded that the excess SiQDs induced abiotic stress, which may have been strong enough to inhibit seedling growth and biomass production. Since oxidative damage is usually measured by lipid peroxidation, the MDA content, a main product of membrane lipid peroxidation, was used to indicate the degree of damage on a cellular level. In our study, the MDA content remained nearly constant at all SiQD concentrations compared with the control group, except at the highest dose of 200 mg L−1 (Fig. S4b†). However, there were no significant differences between the control group and all of the other groups. This result demonstrated that the membrane systems of the Italian lettuce seedling cells were not disrupted or could be self-repaired. Analogously, the previous studies also attested to similar results.1,34,39–41 In Li's work,1 lettuce treated with CDs presented a little negative effect on the cytomembrane, while the chlorophyll content increased in the CD treatment group compared with that for the control group.
To better understand the uptake, translocation, and metabolic processes, we tracked the SiQDs during the Italian lettuce seedling growth using LSM by utilizing their PL properties. As shown in Fig. 8, after cultivating for 15 days in the 100 mg L−1 SiQD solution, the seedlings clearly retained the SiQDs in the root and leaf as indicated by the blue emission areas compared with that of the control samples (Fig. S5†). From the images of the longitudinal section of the root and transverse section of the leaf, it was evident that the SiQDs were absorbed and deposited on the root tissues and then transmitted into the leaves. In the transverse section of the leaf, the SiQDs distributed mainly on the mesophyll cells and stomata, which verified that it was feasible to use the SiQDs to promote the photosynthesis of the Italian lettuce seedlings. The transverse section of the root in the LSM image showed that the SiQDs were transferred from the root to the leaf through the vascular bundle.
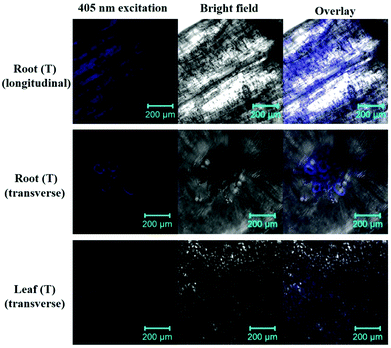 |
| Fig. 8 Uptake and distribution of the SiQDs. From top to bottom, LSM images of a longitudinal section of the root as well as a transverse section of the root and leaf with 100 mg L−1 SiQDs (T). All images were taken under same exposure conditions. | |
To quantitatively detect the silicon content in the aerial and underground part of the Italian lettuce, we adopted an elemental analysis (ESI†). As shown in Fig. 9, the concentrations of the SiQDs significantly affected the absorption and distribution of silicon in the Italian lettuce. The silicon content in the leaves and roots gradually increased with the increase in the SiQD concentration. At a concentration of 200 mg L−1, the silicon content in the leaves was significant increased by 98.55% compared with that for the control group (Fig. 9a). Thus, more SiQDs coated the surface of the chloroplasts at a high concentration. The silicon content in the roots significantly increased more than that for the control group and was significantly increased by 335.42% at 200 mg L−1 (Fig. 9b). The data suggested that a great number of SiQDs accumulated in the root system. It confirmed the above results that the high concentrations of SiQDs significantly inhibited the growth of the Italian lettuce, especially root elongation.
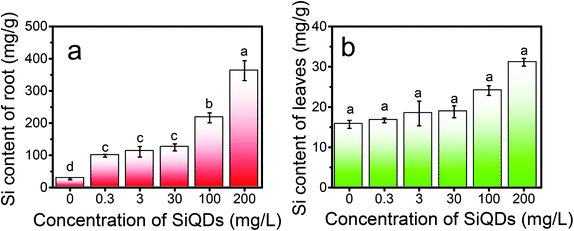 |
| Fig. 9 Si content in the root (a) and leaves (b) of the Italy lettuce seedlings under different concentration treatments of SiQDs. | |
4. Conclusions
Superior to the microwave-assisted method,22 our one-step-hydrothermal synthesis was a high yield and scalable method performed at controllable and relatively low temperatures. The SiQDs reported herein had a delta-shape size distribution with a center at 2.4 nm and a final concentration of 26 g L−1 as prepared. The uniformity in size was further confirmed by the PL emission-wavelength, independent of the excitation-wavelength. More importantly, the primary and secondary amines on organosilicon DAMO were not undergoing an observable cleavage during the hydrolysis-condensation process at the reaction temperature. The existence of a large number of amines was confirmed by the results from FTIR spectroscopy and HRXPS. The final amine-functionalized SiQDs were biocompatible and water soluble. Our SiQDs with a unique size gave a blue emission after excitation by UV light, which matched the blue absorption of the chloroplasts. The reabsorption of 45% of the blue light was observed. The in vitro Hill reaction signalled for the reabsorbed energy to be consumed in order to accelerate the electron transfer in photosynthesis. Finally, the in vivo study on Italian lettuce seedling growth provided evidence that the SiQDs promoted growth not only for the growth morphological parameters but also increased the water and soluble sugar content up to 30 mg L−1 in the nutrient solution. However, the growth was inhibited at a 200 mg L−1 dose. Interestingly, no inhibition was observed during the production of chlorophyll a and b. We explained that the reabsorbed energy could not be unlimitedly utilized by the seedlings due to the attenuation of the water uptake and elevated abiotic stress by the SiQDs at a high dose. The important implications of the present work were not only utilizing the UV portion of solar energy but also attenuating UV toxicities to plants. Si nanoparticles or quantum dots are an excellent class of size tunable, surface modifiable, and low toxic candidates for an application in agriculture. In conclusion, the observed positive effects on the Italian lettuce seedlings provided a new perspective for the use of SiQDs in agriculture.
Conflicts of interest
There are no conflicts to declare.
Acknowledgements
The present work was supported by the National Natural Science Foundations of China (Grant No. 21671070). The project was supported by GDUPS (2018) from Prof. Bingfu LEI, the Project for Construction from High-level University in Guangdong Province of China, the Key Foundation for Basic and Application Research in Higher Education of Guangdong, China (No. 2017KZDXM005), the Guangzhou Science & Technology Project, China (No. 201707010033), and the Special Funds for the Cultivation of Guangdong College Students’ Scientific and Technological Innovation (“Climbing Program” special funds No. pdjhb0080).
Notes and references
- W. Li, S. Wu, H. Zhang, X. Zhang, J. Zhuang, C. Hu, Y. Liu, B. Lei, L. Ma and X. Wang, Adv. Funct. Mater., 2018, 1804004 CrossRef.
-
Q. Jin and Y. Bian, The sunshine industry in the 21st century - ecological agriculture, Tsinghua Univ. Press, Beijing, 2002 Search PubMed.
- S. Chandra, S. Pradhan, S. Mitra, P. Patra, A. Bhattacharya, P. Pramanik and A. Goswami, Nanoscale, 2014, 6, 3647 RSC.
- I. Nabiev, A. Rakovich, A. Sukhanova, E. Lukashev, V. Zagidullin, V. Pachenko, Y. P. Rakovich, J. F. Donegan, A. B. Rubin and A. O. Govorov, Angew. Chem., Int. Ed., 2010, 49, 7217 CrossRef PubMed.
- Y. Xu, J. Fei, G. Li, T. Yuan, Y. Li, C. Wang, X. Li and J. Li, Angew. Chem., Int. Ed., 2017, 56, 12903 CrossRef CAS PubMed.
- J. Zhou, F. Zhang, H. Meng, Y. Zhang and Y. Li, Metab. Eng., 2016, 38, 217 CrossRef CAS PubMed.
- J. Kromdijk, K. Glowacka, L. Leonelli, S. T. Gabilly, M. Iwai, K. K. Niyogi and S. P. Long, Science, 2016, 18(354), 857 CrossRef PubMed.
- M. D. Ooms, C. T. Dinh, E. H. Sargent and D. Sinton, Nat. Commun., 2016, 7, 12699 CrossRef CAS.
- P. Sharma, S. Brown, G. Walter, S. Santra and B. Moudqil, Adv. Colloid Interface Sci., 2006, 123, 471–485 CrossRef.
- C. Buzea, I. Pacheco and K. Robbie, Biointerphases, 2007, 2, MR17 CrossRef PubMed.
- S. Lim, W. Shen and Z. Gao, Chem. Soc. Rev., 2015, 44, 362 RSC.
- M. Yin, E. Ju, Z. Chen, Z. Li, J. Ren and X. Qu, Chem. – Eur. J., 2014, 20, 14012 CrossRef CAS.
- Y. Wang, S. Li, L. Liu, F. Lv and S. Wang, Angew. Chem., 2017, 129, 5392 CrossRef.
- J. P. Giraldo, M. P. Landry, S. M. Faltermeier, T. P. Mcnicholas, N. M. Iverson, A. A. Boghossian, N. F. Reuel, A. J. Hilmer, F. Sen, J. A. Brew and M. S. Strano, Nat. Mater., 2014, 13, 400–408 CrossRef CAS PubMed.
- H. Park, H. H. Ou, A. J. Colussi and M. R. Hoffmann, J. Phys. Chem. A, 2015, 119, 4658 CrossRef CAS.
- J. H. Park, L. Gu, G. Von Maltzahn, E. Ruoslahti, S. N. Bhatia and M. J. Sailor, Nat. Mater., 2009, 8, 331 CrossRef CAS.
- L. Wang, Q. Li, H. Y. Wang, J. C. Huang, R. Zhang, Q. D. Chen, H. Xu, W. Han, Z. Shao and H. Sun, Light: Sci. Appl., 2015, 4, e245 CrossRef.
- X. Chen, X. Zhang, L. Y. Xia, H. Y. Wang, Z. Chen and F. G. Wu, Nano Lett., 2018, 18, 1159 CrossRef CAS PubMed.
- B. Ghosh and N. Shirahata, Sci. Technol. Adv. Mater., 2014, 15, 014207 CrossRef PubMed.
- H. Wang, P. Cao, Z. He, X. He, W. Li, Y. Li and Y. Zhang, Nanoscale, 2019, 11, 17018 RSC.
- A. M. S. A. Qados, Am. J. Exp. Agric., 2015, 7, 78 Search PubMed.
- F. Wu, X. Zhang, S. Kai, M. Zhang, H. Y. Wang, J. N. Myers, Y. Weng, P. Liu, N. Gu and Z. Chen, Adv. Mater. Interfaces, 2016, 2, 1500360 CrossRef.
- N. Aissaoui, L. Bergaoui, J. Landoulsi, J. F. Lambert and S. Boujday, Langmuir, 2012, 28, 656 CrossRef CAS.
- B. Qiao, T. J. Wang, H. Gao and J. Yong, Appl. Surf. Sci., 2015, 351, 646 CrossRef CAS.
- Y. Zhong, X. Sun, S. Wang, F. Peng, F. Bao, Y. Su, Y. Li, S. T. Lee and Y. He, ACS Nano, 2015, 9, 5958 CrossRef CAS PubMed.
- Y. He, Y. Chen, B. Lei, J. Zhuang, Y. Xiao, Y. Liang, M. Zheng, H. Zhang and Y. Liu, Small, 2017, 13, 1700075 CrossRef PubMed.
- J. Yang, X. Zhang, Y. Ma, G. Gao, X. Chen, H. Jia, Y. Li, Z. Chen and F. Wu, ACS Appl. Mater. Interfaces, 2016, 8, 32170 CrossRef CAS.
- L. Giraud, R. Nadarajah, Y. Matar, G. Bazin and S. Giasson, Appl. Surf. Sci., 2016, 370, 476 CrossRef CAS.
- D. Pleul, R. Frenzel, E. Michael and F. Simon, Anal. Bioanal. Chem., 2003, 375, 1276 CrossRef CAS PubMed.
- Y. Z. Wang, T. H. Ko, W. Y. Huang, T. H. Hsieh, K. S. Ho, Y. Y. Chen and S. Hsieh, Polymers, 2018, 10, 1388 CrossRef PubMed.
- K. Dohnalová, A. N. Poddubny, A. A. Prokofiev, W. D. De Boer, C. P. Umesh, J. M. Paulusse, H. Zuilhof and T. Gregorkiewicz, Light: Sci Appl., 2013, 2, e47 CrossRef.
- J. Ruan, P. M. Fauchet, L. DalNegro, M. Cazzanelli and L. Pavesi, Appl. Phys. Lett., 2003, 83, 5479 CrossRef CAS.
- L. Pavesi, N. L. Dal, C. Mazzoleni, G. Franzo and F. Priolo, Nature, 2001, 17, 41 Search PubMed.
- A. A. H. Abdel Latef, A. K. Srivastava, M. S. A. El-Sadek, M. Kordrostami and L. S. P. Tran, Land Degrad. Develop., 2017, 29, 1065 CrossRef.
- P. Wang and B. Grimm, Photosynth. Res., 2015, 126, 189 CrossRef CAS.
- Y. Li, W. Li, H. Zhang, R. Dong, D. Li, Y. Liu, L. Huang and B. Lei, J. Mater. Chem. B, 2019, 7, 1107 RSC.
- M. V. Khodakovskaya, S. K. De, A. S. Biris, E. Dervishi and H. Villagarcia, ACS Nano, 2012, 6, 2128 CrossRef CAS PubMed.
- J. Zhu, Cell, 2016, 167, 313 CrossRef CAS PubMed.
- J. Siegel, K. Záruba, V. Švorčík, K. Kroumanová, L. Burketová and J. Martinec, Nanoscale Res. Lett., 2018, 13, 95 CrossRef.
- L. Zheng, F. Hong, S. Lu and C. Liu, Biol. Trace Elem. Res., 2005, 104, 83 CrossRef CAS.
- X. Gui, M. Rui, Y. Song, Y. Ma, Y. Rui, P. Zhang, X. He, Y. Li, Z. Zhang and L. Liu, Sci. Pollut. Res., 2017, 24, 13775 CrossRef CAS.
Footnote |
† Electronic supplementary information (ESI) available. See DOI: 10.1039/c9nr08187a |
|
This journal is © The Royal Society of Chemistry 2020 |
Click here to see how this site uses Cookies. View our privacy policy here.