DOI:
10.1039/C9PY01429B
(Paper)
Polym. Chem., 2020,
11, 68-74
Heteronetwork organohydrogels with exceptional swelling-resistance and adaptive antifouling performance†
Received
23rd September 2019
, Accepted 10th November 2019
First published on 11th November 2019
Abstract
The integration of hydrophilic and oleophilic components in many organisms (cytomembrane, antifreeze protein molecules, etc.) provides a vital foundation to exert the biological functions related to the activities of life. In this study, a simple and universal strategy has been reported to obtain a robust multi-network organohydrogel based on incompatible hydrophilic and oleophilic polymer chains. The heterogeneous networks enable organohydrogels to swell optionally in liquids with different polarities. Thus, the surfaces of the multi-network organohydrogels display adaptive wettability owing to the reconfigurable surface chemistry induced by different dispersion media, which enables multi-network organohydrogels for promising applications in adaptive antifouling surfaces. In addition, water as the first dispersion medium can penetrate the multi-networks completely, while organic liquids with a low polarity interact with the organic networks as the hydrophilic networks are not hydrated. Thus, the hydrated multi-network organohydrogel displayed a remarkable swelling-resistance to organic liquids with a low polarity and stable mechanical properties.
Introduction
Soft materials consisting of hydrogels and organic elastomers have received extensive attention owing to their unique and tunable physicochemical properties. Hydrogels are a class of soft materials with crosslinked hydrophilic network structures, a high water content, and underwater superoleophobicity, which means they have promising applications as self-cleaning materials, oil/water separation devices, biomedical materials, and so forth.1–17 As for organic elastomers, they are mechanically robust and stable under various environmental conditions and thus have broad applications as sealing materials, damping devices, biomedical devices, dielectric materials, slippery surfaces, stretchable electronics, and so on.18–25 However, hydrogels or organic elastomers with homogeneous networks have various disadvantages. For example, hydrogel surfaces tend to dry and lose their antifouling and self-cleaning properties when exposed to air, and organic elastomers inevitably swell when exposed to organic liquids or vapors, which drastically weakens their mechanical properties. Meanwhile, the surfaces of organic elastomers are typically intolerant to oil contamination from painting, waxing, and secretion of plant oils, which is still a severe environmental issue that needs to be addressed.
In biological systems, the dynamic coexistence of opposing components is ubiquitous and vitally important to provide organisms with environmental adaptability.26–28 In our previous work, we successfully obtained products based on binary hydrophilic/oleophilic heteronetwork gel materials, for example, organohydrogels. Organohydrogels consisting of hydrophilic and oleophilic constituents possess adaptive wettability, excellent flexibility, tunable mechanical properties, along with anti-freezing and anti-drying properties, which demonstrate their potential in applications such as flexible supercapacitors, smart liquid transport, on-demand oil/water separation, and so forth.29–35 However, homogeneously integrating any two mutually incompatible polymer chains remains a challenge owing to the chains repelling the interaction of adjacent chains and phase separation, which inevitably restricts the diversity of the structure and functions of organohydrogels.
Herein, we report a facile strategy to obtain stable multi-network organohydrogels (MN-OHGs) based on two mutually incompatible polymer chains, poly(N,N-dimethylacrylamide) (PDMA) and polydimethylsiloxane (PDMS). By introducing a third intermediate polymer network, poly(n-butyl methacrylate)-co-(lauryl methacrylate) (PBMA-co-PLMA), stable organohydrogels with interpenetrating network structures have been obtained. The multi-network structure enables organohydrogels to optionally swell in liquids with different polarities. In addition, water as the first dispersion medium can penetrate the multi-networks unconditionally, while organic liquids with low polarity interact with the organic networks as the hydrophilic networks are not hydrated. Therefore, when applied in aqueous environments or pre-treated with water, the surfaces of the MN-OHGs display hydrogel-like wettability, for example superoleophobicity, and the MN-OHGs reveal exceptional swelling-resistance to organic liquids with low polarity. On the contrary, when equilibrated in a non-volatile oil and used in air or waterless environments, the MN-OHGs reveal organogel-like slippery surfaces to repel the adhesion of solid organic contaminations such as waxing or painting. It is anticipated that the strategy of introducing intermediate networks may provide a new route to the construction of versatile soft materials based on any incompatible polymer network. The obtained MN-OHGs display exceptional swelling-resistance, adaptive anti-fouling surfaces, and superior mechanical properties, which further broaden their application in complex and volatile scenarios.
Results and discussion
Preparation of MN-OHGs with optional swelling abilities in liquids with different polarities
Generally, organic elastomers are compatible with organic liquids with low polarity; hydrogels are usually compatible with water and some polar solvents (e.g., water, ethanol, and acetone). Thus, in order to integrate the mutually incompatible polymer chains (PDMA and PDMS) and obtain a heterogeneous organohydrogel with interpenetrating network structures, the third intermediate polymer networks, PBMA-co-PLMA, were first introduced into the dehydrated PDMA scaffolds via an in situ polymerization in the presence of amphiphilic solvents (ethanol or acetone), and the precursors of PDMS were then infiltrated. Fig. 1 shows the process used to prepare the MN-OHGs based on hydrophilic and hydrophobic polymer networks. Fig. 1a shows three underlying building networks, these are PDMA, PBMA-co-PLMA, and PDMS. The brief preparation strategy is shown in Fig. 1b, in which the dehydrated PDMA, as the basic scaffold, was used to incorporate two oleophilic networks (PBMA-co-PLMA and PDMS) in sequence to obtain interpenetrating multi-network structures. The obtained MN-OHGs can be swollen by liquids with different polarities. The stable interaction of the water and other polar liquids with PDMA as the first dispersion medium can penetrate the multi-networks unconditionally, while the organic liquids with a low polarity can swell the multi-networks as the hydrophilic networks are swollen by amphiphilic solvents (ethanol or acetone). The non-polar oil-swollen MN-OHG was obtained by immersing the as-prepared MN-OHG sample in acetone and dodecane in sequence (Fig. 1c). The oil-swollen MN-OHG displays an organogel-like slippery surface, which can resist the association of organic contaminants in the air. Whereas, by immersing the as-prepared multi-network materials in water, hydrated MN-OHGs were obtained, which reveals a hydrogel-like surface and leads to improvement of the properties, such as, exceptional swelling resistance to oil, solvent tolerant stable mechanical performances, and anti-fouling properties in water.
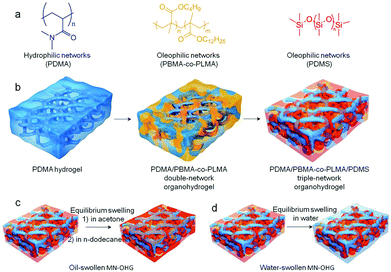 |
| Fig. 1 Schematic illustration of the process used to obtain robust MN-OHGs with optional swelling abilities in liquids with different polarities. (a) Polymer chains used for the fabrication of the multi-network organohydrogel; (b) the formation of the MN-OHGs using PDMA as the basic scaffold to incorporate PBMA-co-PLMA as an intermediate network and PDMS in sequence to obtain the interpenetrating multi-network structures; (c) and (d) the obtained multi-network organohydrogels use water and nonpolar oil as dispersion mediums. By immersing the as-prepared multi-network polymer in acetone and dodecane stepwise, the oil-swollen MN-OHG is obtained (c). Whereas, by immersing the as-prepared multi-network materials in water, the water-swollen MN-OHG is obtained (d). | |
Swelling resistance to oil of the hydrated MN-OHGs
When the as-prepared MN-OHGs were equilibrated in water, the hydrophilic networks spontaneously formed stable, hydrated hydrophilic chains in the internal and interfacial domains. In Fig. 2a and b, it is shown that the surface of the water-swollen MN-OHG is superoleophobic under water, demonstrating an oil (n-dodecane) contact angle (CA) of ∼154.6° at room temperature (25 °C); while the surface of the PDMS elastomer is highly oleophilic in water, presenting an oil (n-dodecane) CA of ∼19.9° (Fig. 2d and e). The superoleophobic surfaces formed can mainly be attributed to the extension of the hydrophilic interfacial networks and the collapse of the oleophilic interfacial networks in water. The continuous water film formed on the surfaces effectively resists the infusion of oil and hence its contamination. In addition, the hydrated MN-OHGs possess excellent swelling resistance to oil, especially to non-polar organic solvents, compared with homogeneous network elastomers such as PDMS. In Fig. 2c, the volume of MN-OHG hardly changes even after a long period (∼20 days) of being fully submerged in oil, which is different from the volume changes observed in the homogeneous network PDMS elastomers after immersion in dodecane for 48 h (Fig. 2f, about three times its original volume). The superhydrophilic surfaces combined with the mutually-constrained internal heteronetworks led to excellent swelling resistance to oil. The internal hydrophilic networks extend into water and further restrain the motion of the oleophilic networks. Various types of organic solvents were adopted to measure the contact angles (CAs) on the surfaces of MN-OHG and PDMS. The statistical data, as shown in Fig. 2g, elucidates that the MN-OHG surfaces are remarkably superoleophobic to various organic solvents in comparison with PDMS, demonstrating apparent oleophilic properties (CAs <30°). Accordingly, in these organic solvents, the experimental results indicate the swelling resistance of MN-OHGs, as show in Fig. 2h. It can be observed that the MN-OHGs can maintain their constant volumes in a variety of non-polar organic solvents, such as n-dodecane, silicone oil, and so forth. In contrast, the PDMS elastomers in solvents such as silicone oil and gasoline were dramatically swollen (Q ≥ 300%).
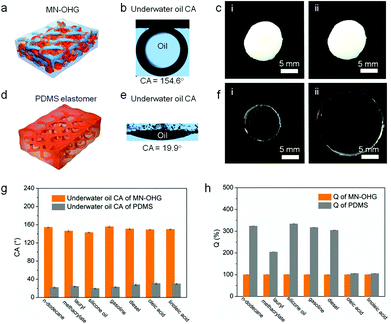 |
| Fig. 2 The swelling resistance behavior of MN-OHGs to various non-polar oils. (a) and (b) The surface of the MN-OHG sample is superoleophobic in water, featuring an oil (dodecane) CA of ∼154.6°; while the surface of PDMS elastomer is still oleophilic in water, indicating an oil (dodecane) CA of ∼19.9° (d) and (e). (c) and (f) The contrast images of the MN-OHG and PDMS which reveal a striking difference in the oil-swelling degree. After 20 days of immersion in n-dodecane, the MN-OHG sample (c) remained non-swollen; while the PDMS sample (f) remarkably increased in volume after immersion in dodecane for ∼48 h. (g) The underwater CAs of different oils on the surfaces of MN-OHG and PDMS, which were measured at room temperature with a settling time of ∼2 min (n = 5, mean ± standard deviation). (h) The swelling ratio (Q) of multi-network organohydrogel and PDMS samples equilibrated in different oils for ∼24 h at room temperature (n = 5, mean ± standard deviation). | |
The remarkable swelling-resistance to organic liquids makes the hydrated MN-OHGs with elastic mechanical properties promising sealing materials for oily liquids, especially in aqueous environments. The testing was performed by designing two simulated oil containers (∼10 mL) under an aqueous environment that was sealed using the multi-network organohydrogel and PDMS, respectively. From Fig. 3a and b, it is evident that the container sealed by MN-OHGs is more effective at resisting oil (dodecane stained with Sudan II) leakage compared with PDMS after a 24 h underwater leakage test. During this process, the MN-OHG seal maintained zero expansion, while the PDMS seal showed a dramatic volume change and deformation owing to the swelling behavior towards oil. Moreover, the oil slick (dodecane) leaked over a period (e.g., 0.5, 1, 3, and 24 h) and was collected and measured using an infrared analyzer for the oil content. From Fig. 3c, we can see that the leakage of oil from the container sealed with PDMS exceeds ∼25% within 24 h owing to the oil infiltration induced by the volume deformation of the PDMS seals. In contrast, the leakage of oil from the container sealed with MN-OHG accounts for less than 1% of the total content. This indicates that the MN-OHG seals can effectively avoid swelling and volume deformation, and ensure better sealing to prevent the leakage of oil, which has promising prospects for sealing underwater oil pipelines and vessels.
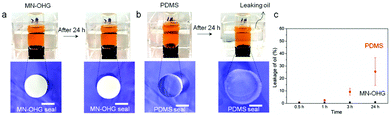 |
| Fig. 3 The contrast in the sealing effect from using MN-OHG and PDMS as sealing materials to protect the storage of dodecane. (a) and (b) Images of the MN-OHG and PDMS seals for oil containers with 20 mL dodecane stained with Sudan II, and the sealing pieces before/after immersion in dodecane for 24 h; (c) curves for oil leakage ratio vs. time using MN-OHG and PDMS as sealing materials. | |
Adaptive anti-fouling properties: oil contamination resistance of hydrated MN-OHG in aqueous environments
In water, the MN-OHGs displayed remarkable underwater superoleophobicity and low adhesion to various oils, such as long-chain alkanes, crude oil, and silicone oil with high viscosity, owing to the hydrated hydrophilic networks extending in the interface of the solid/liquid. The antifouling experiments were carried out on the surfaces of MN-OHG samples and PDMS as a control sample, which were fixed vertically in the underwater environments (Fig. 4a and c). A droplet of silicone oil (with a viscosity of ∼4000 cst) stained with Sudan III was added dropwise onto the surface of MN-OHG, and the oil droplets barely adhered to the surface and thus slipped off quickly (Fig. 4b). However, owing to the highly oleophilic surfaces of PDMS, the oil stain with a high viscosity and weak flowability stuck to the surface of the PDMS steadily (Fig. 4c). Even after removal from the water, the oil stain on the surface of PDMS was still stuck steadily (Fig. 4d). The surface nanotopographies of the organohydrogel also play an essential role in enhancing the contact areas of water and material surfaces. In Fig. 4e, it can be seen that the MN-OHG surface is rich in porous microstructures, and the average size of these micropores is ∼200 nm. Water can be trapped easily in the porous microstructures as a repelled liquid, to avoid the adhesion of various oil contaminants further. In contrast, PDMS cannot resist the adhesion of oil contamination, mainly due to the homogeneous chemical composition of its oleophilic surface.
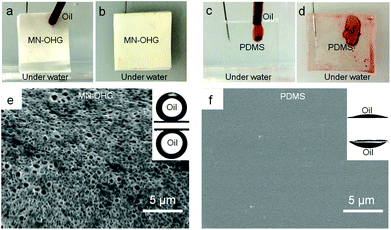 |
| Fig. 4 Tests to detect the resistance to oil contamination of MN-OHG and PDMS. (a) and (b) The surfaces of MN-OHG resist the adhesion of viscous oil (silicone oil stained with Sudan III, the viscosity is ∼4000 cst). (c) and (d) The PDMS surfaces tend to be vulnerable to oil contamination as a control sample. (e) and (f) SEM images of the MN-OHG and PDMS surfaces. The MN-OHG surface shows evident porous structures which promote the superoleophobic properties under water (the corresponding contact angles of both 1,2-dichloroethane and dodecane are ≥150°); whereas the flat PDMS surface (d), still maintains its oleophilic performance under water (the corresponding contact angles of both 1,2-dichloroethane and dodecane are ≤30°). | |
Adaptive anti-fouling properties: solid organic contamination resistance of the MN-OHG rich in non-volatile oil
Usually, hydrophilic polymers are swollen in water to form stable hydrogels; while in a dry atmosphere, the hydrophilic polymer networks gradually collapse and exhibit plastic-like mechanical properties. Meanwhile, they may lose the antifouling and self-cleaning properties originating from their low-adhesion and slippery surfaces to undergo oil contamination underwater. Compared with hydrogels losing antifouling surfaces owing to collapsed hydrophilic networks in dry air (Fig. 5d), MN-OHGs can be optionally swollen by non-volatile oil and exist as an organogel, displaying superior low-adhesion properties when exposed to air. In Fig. 5b and c, it is shown that the semi-dried oil painting can be removed from the MN-OHG surfaces quickly (Fig. 5b); and the wax membrane can slip down easily from the MN-OHG surfaces at an inclination angle of ∼20° (Fig. 5c). We deduced that the oil membrane formed on the organohydrogel surfaces plays a critical role in preventing the solidification of organic paint/wax on the solid–liquid interface. Moreover, the dodecane-swollen MN-OHG demonstrated a plastic-like performance with a higher stiffness and better abrasion resistance compared to the typical organogel elastomers, as the collapsed hydrophilic network serves as the scaffold networks to constrain the expansion of the oleophilic networks.
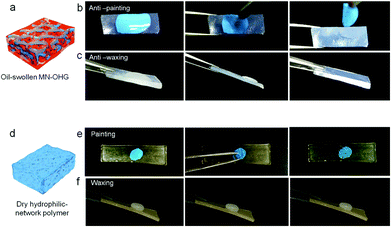 |
| Fig. 5 The excellent anti-painting and anti-waxing properties of the oil-swollen MN-OHG in comparison with the collapsed hydrophilic network surfaces. (a) Schematic diagram of the oil-swollen MN-OHG with heteronetworks; (b) and (c) the anti-painting and anti-waxing properties of the oil-swollen MN-OHG. The semi-dried oil painting (b) can be uncovered and removed easily. The anti-waxing properties of the oil-swollen MN-OHG (c); in which the solidified paraffin wax on the oil-swollen MN-OHG surfaces shows low adhesion and a smooth sliding performance (tilt angle of ∼20°). In contrast, the wax and paint were solidified and adhered firmly onto the dried hydrophilic surfaces in the air as a control (d)–(f). | |
Oil-resistant mechanical stability of the hydrated MN-OHGs
The performances in terms of the mechanical properties of the hydrated MN-OHG are characterized by the rheology and compression tests (Fig. 6a and b). The storage modulus of the hydrated MN-OHG with elastomer-like properties was much higher than that of the hydrogel and double-network organohydrogel (OHG) based on the PDEA and PBMA-co-PLMA. In addition, when ω is between 10−1 and 2 rad s−1, the G′ of MN-OHG shows a slightly linear increasing trend, which is comparable to the G′ curve of the PDMS (wprecursor/wcrosslinker = 35
:
1). The compression curves, as shown in Fig. 6b, also displayed an enhancement in the compression strength of MN-OHGs compared to the PDMA hydrogel and double-network organohydrogel. More importantly, the MN-OHG showed a stable oil-resistant mechanical stability. The inevitable swelling behaviors of organic elastomers in organic liquids can drastically weaken the mechanical properties, which severely restricts their scope of application and development. The exceptional swelling resistance to the oil of the hydrated MN-OHGs produced by the heterogeneous structures also maintained the mechanical stability in organic liquids. Fig. 6c displays the changes in the extent of G′ (when ω = 1 rad s−1) of the different samples after being swollen in dodecane for 24 h. We can see that the samples MN-OHG, OHG, and PDMA produced very slight changes in the G′ (less than 5%) owing to the collapse of the hydrophilic networks in oil, while PDMS (wprecursor/wcrosslinker ∼35
:
1 and 43
:
1) leads to a significant difference in the decreasing G′ of about 30% and 40%. Similarly, the changes in the extent of the compressive strength are shown in Fig. 6d. MN-OHG, PDMA, and OHG can maintain a relatively stable compressive strength with a reduced difference of less than 15%, owing to the existence of the hydrophilic networks, while for PDMS the resultant changes are more than 80%. In brief, MN-OHGs reveal superior elastomer-like mechanical properties compared to the PDMA hydrogel and OHG. Meanwhile, they also displayed an excellent solvent-resistant mechanical stability and shape retention compared with the elastomers with homogeneous oleophilic networks.
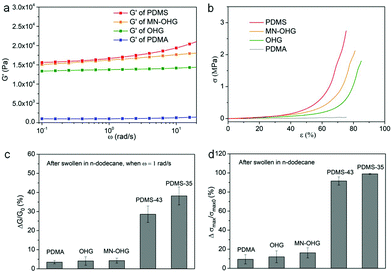 |
| Fig. 6 Mechanical properties and solvent-resistant mechanical stability of the hydrated MN-OHG samples compared with the PDMS, OHG, and PDMA samples. (a) The viscoelastic spectra (G′) of PDMS, MN-OHG, OHG, and PDMA at 25 °C when ω = 10−1–10 rad s−1. (b) Compressive stress–strain curves of the PDMS, MN-OHG, OHG, and PDMA samples; (c) after immersion in n-dodecane for 24 h, the decline of G′ induced by the swelling of different elastomers is shown, in which G0 represents G′ before the swelling when ω = 1 rad s−1, and ΔG = G0 − G1, in which G1 represents G′ after swelling when ω = 1 rad s−1; (d) the decline of σmax induced by the swelling of the different elastomers, in which σmax represents the maximum strength of different materials during the compression process, and σmax 0 represents σmax before swelling in dodecane, and Δσmax = σmax 0 − σmax 1, in which σmax 1 represents σmax after swelling in dodecane. | |
Conclusions
In summary, we report a facile strategy to prepare robust MN-OHGs based on incompatible hydrophilic and oleophilic polymer chains (PDMA and PDMS) via introducing a third intermediate polymer network. The MN-OHGs can use liquids with different polarities as dispersion media to optionally obtain hydrated MN-OHGs and oil-swollen MN-OHGs. Thus, the surfaces of the MN-OHGs display adaptive wettability owing to the reconfigurable surface features (hydrogel-like and organogel-like features), which provide multi-network organohydrogels for promising applications in adaptive antifouling materials. In addition, in aqueous environments, the hydrated MN-OHGs show excellent oil contamination resistance; while in air, the swelled MN-OHGs using non-volatile oil automatically produced solid organic contamination resistance. Moreover, water is proven to be the prior dispersion media, and can penetrate the multi-networks unconditionally. The multi-network organohydrogels display exceptional swelling-resistance in organic liquids and stable solvent-tolerant mechanical performances. This study may promote the application of soft materials in areas related to adaptive self-cleaning surfaces, sealing materials, solvent-resistant materials, and so forth. Meanwhile, the facile and universal method, as reported in this study, may apply to most of the immiscible and complementary polymer systems allowing construction of more heterogeneous soft materials with complex functions.
Experimental
Materials
To prepare the poly(N,N-dimethylacrylamide) (PDMA) hydrogel, the following reagents were used: N,N-dimethylacrylamide (DMA) as the monomer, N,N′-methylene bis (acrylamide) (BIS) as the crosslinking agent, ammonium persulfate (APS) as the initiator, and de-ionized water (DI-water) from Milli-Q. Unless otherwise noted, all the reagents employed were analytical reagents (J&K Scientific) and used as received.
Organogel polymerization precursors consisted of n-butyl methacrylate (BMA) and lauryl methacrylate (LMA) as monomers, ethylene glycol dimethacrylate (EGDMA) as the crosslinking agent, 2,2-diethoxyacetophenone (DEAP) as the photoinitiator, and anhydrous ethanol (Sinopharm Chemical Reagent Co. Ltd) as a solvent. PDMS elastomer (polydimethylsiloxane Sylgard 184) precursor and curing agent were purchased from Dow Corning Corporation. Dodecane and other non-volatile organic liquids (lauryl methacrylate, oleic acid, and linoleic acid) were purchased from J&K Scientific. Gasoline and diesel were provided by PetroChina.
Preparation of the PDMA/PBMA-co-PLMA/PDMS based multi-network organohydrogel
A hydrophilic polymer network was first prepared by the radical polymerization of N,N-dimethylacrylamide (10 wt%) as the monomer with methylene-bisacrylamide (0.1 wt%) as the crosslinker at 70 °C for 2 h. Through adequate dehydration using acetone as the dispersion medium, the PDMA networks were then swollen in the as-prepared ethanol solution consisting of lauryl methacrylate (34.1 wt%), n-butyl methacrylate (35.0 wt%), ethylene glycol dimethacrylate (0.62 wt%) as a crosslinker and 2,2-diethoxyacetophenone (0.41 wt%) as a photoinitiator. Then, the as-swollen PDMA networks were irradiated under a UV light (500 W high-pressure mercury arc lamp, Perfectlight) for 60 min, to obtain the PDMA/PBMA-co-PLMA based double-network organohydrogels. The as-prepared double-network organohydrogels were further immersed in an organic solution of PDMS (including 30 wt% acetone, 30 wt% n-dodecane, 36.4 wt% PDMS precursor, and 3.64 wt% curing agent) for ∼30 h. Finally, the swollen networks were heated continuously at 80 °C for ∼48 h. The weight ratio of the three polymer networks was determined via measuring the dry-weight of parallel samples. If not specified, the MN-OHG samples used were composed of ∼40 wt% hydrophilic networks, ∼45 wt% PBMA-co-PLMA networks, and ∼15 wt% PDMS networks.
Swelling process in oil/water dispersion media
To obtain MN-OHGs with swelling-resistance and underwater oil contaminant-resistance properties, water-swelling was necessary by immersing the as-prepared MH-OHGs (with/without oil) in water to reach a saturation state. To achieve the anti-painting and anti-waxing properties, oil-swollen MN-OHGs were equilibrated in acetone first and then the samples were immersed in a non-volatile oil to reach the saturation state.
Contact angle measurements
The contact angle measurements were performed using an OCA-20 machine (Dataphysics, Germany) at ambient temperature. The droplet volume was controlled precisely at 2 μl. For each sample, more than five spots were determined, and then the average of the results was considered. All of the samples, including the MN-OHGs and PDMS elastomers, were cut into flat sheets with a thickness of 2–4 mm. The data for the CAs of different organic liquids (including n-dodecane, lauryl methacrylate, silicone oil, gasoline, diesel, oleic acid, and linoleic acid) on the samples under water were collected at room temperature (∼25 °C).
SEM characterization
The MN-OHG samples were swollen in distilled water to a saturation state and then frozen quickly in liquid nitrogen, followed by lyophilization at −85 °C for ∼48 h. A field-emission scanning electron microscope (JSM-7500F, Japan) was used to characterize the surface topographies of the MN-OHG and PDMS samples.
Rheological characterization
Rheological characterization was performed on a modular compact rheometer (MCR302, Anton Paar) with a parallel plate (PP 15 mm), which was used to carry out the oscillatory tests. The gel samples were melded into a round plate with a diameter of 15 mm and a thickness of ∼4 mm.
Compressive tests
The compressive stress–strain tests were performed using a universal tensile machine (Instron). The cylindrical samples (∼10 mm diameter and ∼5 mm thickness) were placed on the lower plate and then compressed by the upper plate, at the rate of 5 mm min−1.
Swelling ratio measurements of elastic MN-OHGs and homonetwork PDMS elastomers in different organic liquids
The swelling ratios (Q) of elastic MN-OHGs and homonetwork PDMS elastomers in different organic liquids were determined based on the changes in the macroscopic volume. This was calculated from the volume ratio of the elastomers after (V) and before (V0) swollen by the organic solvents, as shown below.
In which V is the volume of the elastomer after swelling, and V0 is the initial volume of the elastomer.
Conflicts of interest
The authors declare no competing financial interests.
Acknowledgements
This research was supported by the National Natural Science Foundation (21905009), the Science Foundation for Young Scientists (PXM2019_014213_000007), and Funding of the Key Laboratory of Bio-inspired Materials and Interfacial Science, TIPC, CAS.
Notes and references
- Y. Zhu, J. Wang, F. Zhang, S. Gao, A. Wang, W. Fang and J. Jin, Adv. Funct. Mater., 2018, 28, 1804121 CrossRef.
- W. Lu, X. Le, J. Zhang, Y. Huang and T. Chen, Chem. Soc. Rev., 2017, 46, 1284–1294 RSC.
- L. Ma, S. Chen, D. Wang, Q. Yang, F. Mo, G. Liang, N. Li, H. Zhang, J. A. Zapien and C. Zhi, Adv. Energy Mater., 2019, 9, 1803046 CrossRef.
- W. Kong, C. Wang, C. Jia, Y. Kuang, G. Pastel, C. Chen, G. Chen, S. He, H. Huang, J. Zhang, S. Wang and L. Hu, Adv. Mater., 2018, 30, 1801934 CrossRef.
- P. Lin, S. Ma, X. Wang and F. Zhou, Adv. Mater., 2015, 27, 2054–2059 CrossRef CAS.
- H. He, M. Liu, J. Wei, P. Chen, S. Wang and Q. Wang, Adv. Healthcare Mater., 2016, 5, 648–652 CrossRef CAS.
- C. Yang and Z. Suo, Nat. Rev. Mater., 2018, 3, 125–142 CrossRef CAS.
- H. Yuk, S. Lin, C. Ma, M. Takaffoli, N. X. Fang and X. Zhao, Nat. Commun., 2017, 8, 14230 CrossRef CAS.
- T. B. H. Schroeder, A. Guha, A. Lamoureux, G. Vanrenterghem, D. Sept, M. Shtein, J. Yang and M. Mayer, Nature, 2017, 552, 214 CrossRef CAS.
- E. Prince and E. Kumacheva, Nat. Rev. Mater., 2019, 4, 99–115 CrossRef.
- R. Takahashi, T. L. Sun, Y. Saruwatari, T. Kurokawa, D. R. King and J. P. Gong, Adv. Mater., 2018, 30, 1706885 CrossRef PubMed.
- Z. Xue, S. Wang, L. Lin, L. Chen, M. Liu, L. Feng and L. Jiang, Adv. Mater., 2011, 23, 4270–4273 CrossRef CAS.
- Y. Cai, Q. Lu, X. Guo, S. Wang, J. Qiao and L. Jiang, Adv. Mater., 2015, 27, 4162–4168 CrossRef CAS.
- S. Liang, Y. Zhang, H. Wang, Z. Xu, J. Chen, R. Bao, B. Tan, Y. Cui, G. Fan, W. Wang, W. Wang and W. Liu, Adv. Mater., 2018, 30, 1704235 CrossRef.
- M. Liu, S. Wang, Z. Wei, Y. Song and L. Jiang, Adv. Mater., 2009, 21, 665–669 CrossRef CAS.
- J. Sun, X. Zhao, W. R. K. Illeperuma, O. Chaudhuri, K. H. Oh, D. J. Mooney, J. J. Vlassak and Z. Suo, Nature, 2012, 489, 133–136 CrossRef CAS.
- J. S. Kahn, Y. Hu and I. Willner, Acc. Chem. Res., 2017, 50, 680–690 CrossRef CAS.
- H. Liu, P. Zhang, M. Liu, S. Wang and L. Jiang, Adv. Mater., 2013, 25, 4477–4481 CrossRef CAS.
- X. Yao, S. Wu, L. Chen, J. Ju, Z. Gu, M. Liu, J. Wang and L. Jiang, Angew. Chem., Int. Ed., 2015, 54, 8975–8979 CrossRef CAS.
- M. Park, J. Im, M. Shin, Y. Min, J. Park, H. Cho, S. Park, M.-B. Shim, S. Jeon, D.-Y. Chung, J. Bae, J. Park, U. Jeong and K. Kim, Nat. Nanotechnol., 2012, 7, 803 CrossRef CAS.
- Y. Qiu, E. Zhang, R. Plamthottam and Q. Pei, Acc. Chem. Res., 2019, 52, 316–325 CrossRef CAS PubMed.
- M. Tang, R. Zhang, S. Li, J. Zeng, M. Luo, Y.-X. Xu and G. Huang, Angew. Chem., Int. Ed., 2018, 57, 15836–15840 CrossRef CAS.
- F. Wu, S. Chen, B. Chen, M. Wang, L. Min, J. Alvarenga, J. Ju, A. Khademhosseini, Y. Yao, Y. S. Zhang, J. Aizenberg and X. Hou, Small, 2018, 14, 1702170 CrossRef.
- Y. Wu, L. Wang, X. Zhao, S. Hou, B. Guo and P. X. Ma, Biomaterials, 2016, 104, 18–31 CrossRef CAS.
- E. Ducrot, Y. Chen, M. Bulters, R. P. Sijbesma and C. Creton, Science, 2014, 344, 186–189 CrossRef CAS.
- M. Liu and L. Jiang, Sci. China Mater., 2016, 59, 239–246 CrossRef CAS.
- B. Su, W. Guo and L. Jiang, Small, 2015, 11, 1072–1096 CrossRef CAS.
- K. Liu, C. Wang, J. Ma, G. Shi, X. Yao, H. Fang, Y. Song and J. Wang, Proc. Natl. Acad. Sci. U. S. A., 2016, 113, 14739–14744 CrossRef CAS.
- Y. Shang, J. Wei, C. Wu and Q. Wang, ACS Appl. Mater. Interfaces, 2018, 10, 42959–42966 CrossRef CAS.
- F. Chen, D. Zhou, J. Wang, T. Li, X. Zhou, T. Gan, S. Handschuh-Wang and X. Zhou, Angew. Chem., Int. Ed., 2018, 57, 6568–6571 CrossRef CAS.
- Z. Zhao, K. Zhang, Y. Liu, J. Zhou and M. Liu, Adv. Mater., 2017, 29, 1701695 CrossRef.
- H. Gao, Z. Zhao, Y. Cai, J. Zhou, W. Hua, L. Chen, L. Wang, J. Zhang, D. Han and M. Liu, Nat. Commun., 2017, 8, 15911 CrossRef CAS.
- Q. Rong, W. Lei, L. Chen, Y. Yin, J. Zhou and M. Liu, Angew. Chem., 2017, 129, 14347–14351 CrossRef.
- Q. Rong, W. Lei, J. Huang and M. Liu, Adv. Energy Mater., 2018, 8, 1801967 CrossRef.
- Z. Zhao, C. Li, Z. Dong, Y. Yang, L. Zhang, S. Zhuo, X. Zhou, Y. Xu, L. Jiang and M. Liu, Adv. Funct. Mater., 2019, 29, 1807858 CrossRef.
Footnote |
† Electronic supplementary information (ESI) available. See DOI: 10.1039/c9py01429b |
|
This journal is © The Royal Society of Chemistry 2020 |
Click here to see how this site uses Cookies. View our privacy policy here.