DOI:
10.1039/C9PY01625B
(Paper)
Polym. Chem., 2020,
11, 61-67
Inky flower-like supermicelles assembled from π-conjugated block copolymers†
Received
29th October 2019
, Accepted 13th November 2019
First published on 28th November 2019
Abstract
Flower-like hierarchical nano-/meso-structures assembled from different kinds of materials have great application potential due to their advantages of high surface-to-volume ratios, high loading and adsorption capacities. These biomorphic superarchitectures are commonly obtained using inorganic, organic–inorganic hybrid and block copolymer (BCP) materials. However, the self-assembly of functional π-conjugated BCPs with weak intermolecular interactions has rarely been realized through a facile approach; moreover, the corresponding studies on how the polymer properties and assembly conditions influence the ultimate dimensionality and morphology of micelles are also scarce. Herein, we report the realization of inky flower-like hierarchical supermicelles taking advantage of the ultrashort corona block in the system of poly(2,5-di(2′-octyloxy)-1,4-phenylenevinylene)7-block-poly(2-vinylpyridine)n (PPV7-b-P2VPn). The dimensionality and morphology are successfully controlled by altering the block ratio of BCPs and the polarity of the selective solvent. Then, the growth process of the flower-like supermicelles is observed from the initial flower heart to the final flower petals. Furthermore, it is demonstrated that the formation of micelles is driven by the π–π interactions between the PPV7 blocks. Our study on constructing and regulating hierarchical supermicelles in a new functional π-conjugated system would be valuable to other BCPs-based assembly systems.
Introduction
Various functional hierarchical superstructures that assemble in nature have inspired the development of artificially controlled self-assembly in the synthetic system.1 Amphiphilic block copolymers (BCPs) are commonly used as building blocks for self-assembly due to the diversity of their choice and functionalization.2,3 Well-controlled 1-dimensional (1D), 2-dimensional (2D) and hierarchical complex nano-/meso-micelles with various morphologies have been constructed in selective solvents.4–7
As one of the most common biomorphic superstructures, flower-like nano-/meso-structures, which are termed nanoflowers/mesoflowers, have attracted considerable interest in the field of catalysis, energy storage, sensors, and absorbent and support materials owing to their characteristics of high surface-to-volume ratios and high loading and adsorption capacity.8–10 Until now, most studies of nanoflowers have concentrated on inorganic materials such as CuO,11 SnO2,12 MoS2
13 and organic–inorganic hybrid materials like metal-biological molecules.14 BCP micelles have also been used as templates to be decorated with small inorganic nanoparticles (NPs).15 Notably, sunflower-like or butterfly-like architectures anchoring the BCPs micelles to the surface of inorganic particles have been realized through multistep self-assembly.16 In addition to the hybrid strategy with inorganic materials, BCPs themselves are also a good choice to prepare complex biomorphic superassemblies. In particular, impressive results have been achieved utilizing a crystallization driven self-assembly (CDSA) approach in poly(ferrocenyldimethylsilane) (PFS)-based BCPs.17,18 Abundant self-assemblies have been prepared using this strategy, including barbed and branched mesostructures,19 fluorescent flower-like aggregates,20 star-like supermicelles21 and so on. On the other hand, the self-assembly of π-conjugated block copolymers has attracted great attention owing to their unique electronic and optoelectronic properties. For instance, bundled and branched supermicelles were obtained from BCPs incorporating π-conjugated blocks of semiconducting poly(3-hexylthiophene) (P3HT) and poly(3-hexylselenophene) (P3HS).22–25 1D fibers with controllable length and 2D square micelles with controllable size were realized in recent reports using poly(p-phenylenevinylene) (PPV) and oligo(p-phenylenevinylene) (OPV) based BCPs.5,26–28 However, until now, there have still been limited studies on the assembly of hierarchical biomorphic flower-like supermicelles based on OPV and PPV BCPs (π-conjugated) with weak intermolecular interactions through a facile approach. Meanwhile, the related systematic study on the regulation of dimensionality and morphology through altering the assembly conditions is also lacking.
In the self-assembly of BCPs, it is well known that the core-forming block favors 2D-platelet growth while the stretching corona-forming block causes the curvature of the core–corona interface.29,30 Accordingly, the BCPs with a longer corona tend to form 1D fibers whose interfaces are more curved, yet a shorter corona favors the development of 2D-platelet structures with a lower curvature. Thus, it can be speculated that the BCPs with an ultrashort corona block may have potential in realizing new and more complex architectures. In this work, a systematic study on the one-step formation of inky flower-like superstructures via solvent-induced micellization of amphiphilic poly(2,5-di(2′-octyloxy)-1,4-phenylenevinylene)-block-poly(2-vinylpyridine) (PPV7-b-P2VPn) is reported (Scheme 1).
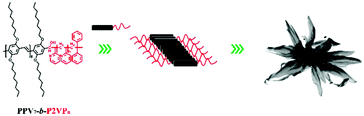 |
| Scheme 1 The chemical structure of PPV7-b-P2VPn and schematic of the formation of inky flower-like architectures. | |
Results and discussion
Design and synthesis of PPV-based diblock copolymers
Inspired by the previous work on poly(2,5-di(2′-ethylhexyloxy)-1,4-phenylenevinylene) BCPs,5 we assumed that the alkyl side chains may have a pivotal impact on the spatial arrangement of a rigid core, which may lead to other novel morphologies. Thus, we further designed poly(2,5-di(2′-octyloxy)-1,4-phenylenevinylene)-block-poly(2-vinylpyridine). In order to realize the formation of hierarchical superstructures utilizing π–π interactions, we synthesized a series of BCPs of PPV7-b-P2VP25, PPV7-b-P2VP18, and PPV7-b-P2VP10 (the subscripts refer to the number of repeat units) with the increasing block ratios of 1/3.6, 1/2.6 and 1/1.4 (denoted by RPPV/P2VP). At first, the PPV-aldehyde homopolymer was prepared through Siegrist polycondensation, then PPV-b-P2VP was prepared through quenching the anionic polymerization of P2VP using a PPV aldehyde.5 The monomer number, block ratios and polydispersity were determined by nuclear magnetic resonance (NMR) and gel permeation chromatography (GPC) (the synthesis details are shown in the ESI, Scheme S1, Fig. S1–S7, and Table S1†).
Self-assembly of PPV-b-P2VP and formation of supermicelles
The BCPs were dissolved in the selected solvent methanol at a concentration of 0.02 mg mL−1. Then, the solutions were heated at 90 °C for 1 h and slowly cooled to room temperature (25 °C) followed by aging for 24 h, as in previous work,29,30 which is called a “heating–cooling–aging” process.5 After the aging process, an aliquot of solution was drop-cast onto a piece of copper grid and transmission electron microscope (TEM) characterization was implemented. The results reveal that the assembled morphology is closely related to the block ratio. Specifically, when PPV7-b-P2VP25 with a long enough corona is used, large-scale scarf-like micelles can be observed (Fig. 1a), where several agglomerates which present radial bundle-like structures are interspersed (Fig. 1b). Herein, the analogous flower-like architectures initially appear. When the corona block is shortened to 18, there are nearly all 3D flower-like supermicelles instead of a large amount of dispersed 2D scarves (Fig. 1c and d). When the corona block further shortens to 10, more uniform inky flower-like supermicelles whose petals present a greater 2D tendency than above two can be obtained (Fig. 1e and f).
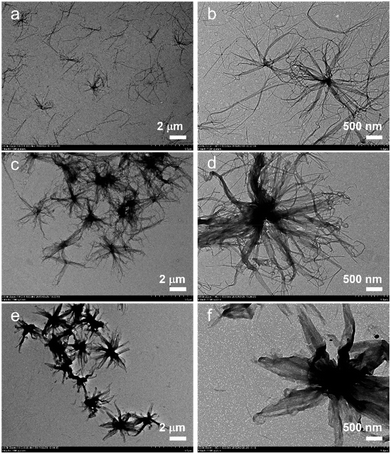 |
| Fig. 1 TEM characterizations of micelles assembled from PPV7-b-P2VPn in methanol. (a and b) PPV7-b-P2VP25 (RPPV/P2VP = 1/3.6); (c and d) PPV7-b-P2VP18 (RPPV/P2VP = 1/2.6); and (e and f) PPV7-b-P2VP10 (RPPV/P2VP = 1/1.4). | |
Such results are mainly due to two reasons. From a thermodynamic point of the view, steric repulsion between the corona blocks has an impact on these different morphologies, as the successive decline of inter-corona repulsion sprung from contraction of the corona block can lead to stronger π–π interactions which are related to the hierarchical association. From the kinetic effect, reducing the length of the corona results in an increase in dynamic behavior and triggers the morphological transition from 2D scarves to 3D flower-like supermicelles. Overall, this means that a shorter corona block makes the lateral growth of PPV core more feasible, which contributes to the morphological differences between the 2D scarves and 3D supermicelles. Thus, the ultrashort corona block leads to the formation of uniform hierarchical supermicelles.31
Inky flower morphologies have also been observed by atomic force microscopy (AFM), He-ion microscopy (HIM) and cryo-TEM (Fig. 2). Lower magnification images of HIM account for the emergence of large-scale micelles (Fig. S8†). Furthermore, a drop of PPV7-b-P2VP10 solution was extracted and dropped onto a piece of slide to complete laser scanning confocal microscopy (LSCM) characterization. As a result, the fluorescent object with flower-shapes which is extremely light in the central part and relatively weak in the parts of the petals around could be directly observed (Fig. S9†), which demonstrates that the formation of flower-like micelles has occurred in the process of cooling and aging rather than in the solvent evaporation.
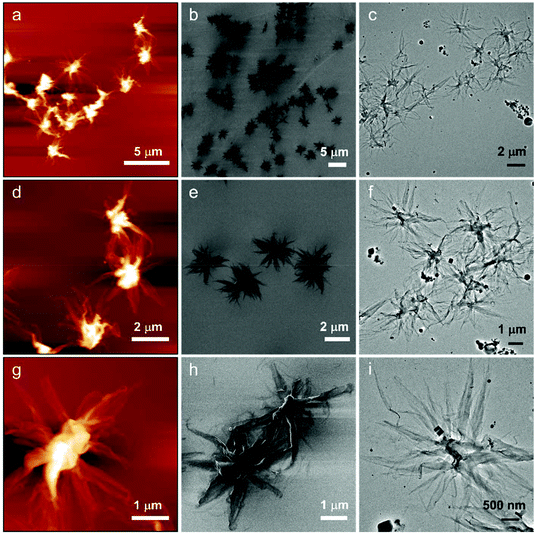 |
| Fig. 2 Inky flower-like supermicelles imaged by (a), (d), and (g) AFM; (b), (e), and (h) HIM; and (c), (f), and (i) Cryo-TEM. | |
Growth process of supermicelles
In order to investigate the assembly process to reveal how these micelles form, a series of TEM characterizations of these 3 BCPs were conducted. After the solutions were heated for 1 h, an aliquot of solution was extracted and drop-cast onto the copper grid at specific temperature nodes during the cooling process and certain time points during the aging process. It can be found that in all solutions, when the temperature decreases from 90 to 70 °C, tiny fibres appeared, meanwhile some 2D states in aggregation are observed (Fig. 3a and S10a, e†). When the temperature further decreases to 60 °C, in the solution of PPV7-b-P2VP25, the small platelets of the central parts of the scarves and overlapped platelets which are about to grow into final complex micelles exist (Fig. S10b†). While in the other two solutions, the rudiments of flower-like micelles which have grown flower hearts and preliminary petals are observed (Fig. 3b and S11b†). There are also a great deal of fibres that coexist with the growing supermicelles. Thus, we speculate that these little objects are formed by precipitation of unimers that are not involved in growth when the solution was dropped and cooled. Then, at 40 °C, the growth of micelles is basically completed on account of their nearly complete morphology, nevertheless the precipitated nanofibers mean there are still unimers in the solution, which indicates that the assembly process has not finished (Fig. 3c, S10c and S11c†). Finally, after the solutions are aged for 24 h, the supermicelles are completely obtained (Fig. 3d, S10d and S11d†). The schematic diagram of the growth process of flower-like supermicelles is shown in Scheme 2.
 |
| Fig. 3 Growth process of inky flower-like supermicelles from PPV7-b-P2VP10. TEM observation of growth monitored at (a) 70 °C, (b) 60 °C, (c) 40 °C and (d) aging for 24 h. | |
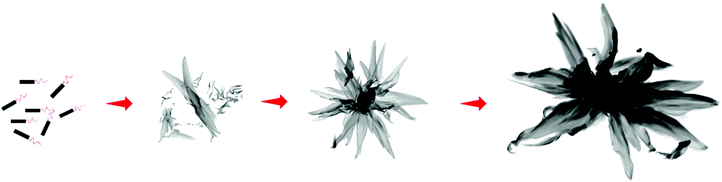 |
| Scheme 2 Schematic diagram of growth process of flower-like supermicelles. | |
UV-Vis absorption variation in assembly process
Then, to explore the growth process and driving force of the assembly, the UV-Vis absorption spectra of 3 BCPs in methanol along with changes in temperature and time were all measured (Fig. 4). The results show that all the spectra exhibit a little redshift of about 2–3 nm during the initial cooling process. This bathochromic shift is mainly caused by an increase in proximity of the BCP unimers, which is caused by the hydrophobic interactions of the conjugated core. Then, there is a relatively significant blueshift in the subsequent process, which indicates the driving force of the formation of the assembly objects is mainly from H-aggregation of the π-conjugated PPV7 block.32
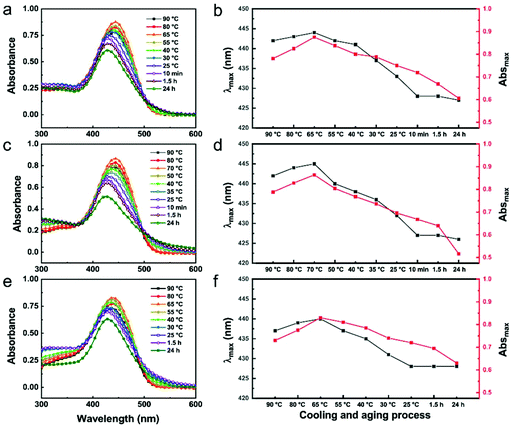 |
| Fig. 4 UV-Vis absorption spectra of PPV7-b-P2VPn with varying temperature and aging time in methanol, (a) PPV7-b-P2VP25; (c) PPV7-b-P2VP18; and (e) PPV7-b-P2VP10. Variations of the maximum absorption wavelength and intensity with varying temperature and aging time in methanol, (b) PPV7-b-P2VP25; (d) PPV7-b-P2VP18; and (f) PPV7-b-P2VP10. | |
Firstly, in the early stages of the cooling process, the peaks experience a complete redshift from 90 to 70 or 65 °C for all samples. Subsequently, during the continuous cooling and aging process, the spectra show a blueshift with a decrease in intensities due to aggregation. Until the solutions are aged for 10 min, all of the peak positions are no longer blue-shifted, which is likely to demonstrate the completion of the basic morphology. This result is also consistent with the TEM observations of the growth process. Then, after the solution was aged for 10 min to 24 h, there were only reductions in absorption intensities caused by micellar congregation (Fig. 5b, d and e). Moreover, a greater blueshift of about 14 nm in the spectra of PPV7-b-P2VP25 and PPV7-b-P2VP19 compared to that of PPV7-b-P2VP10 around 8 nm indicates that the arrangements from H-aggregation of the former two BCPs are more complete and ordered than that of the PPV7-b-P2VP10. Increasing the kinetic effect of PPV7-b-P2VP10 means the unimers do not have enough time to tune the arrangement to a more ordered mode, which contributes to this spectral difference.
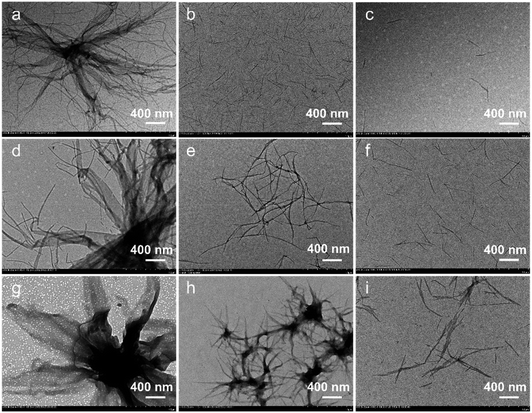 |
| Fig. 5 TEM images (×10k) of micelles grown from PPV7-b-P2VP25, PPV7-b-P2VP18 and PPV7-b-P2VP10 in various polar solvents, (a), (d), and (g) methanol; (b), (e), and (h) isopropanol and (c), (f), and (i) isobutanol. | |
Solvent effect on self-assembly
The solvent is a direct and vital factor in the solution assembly. Adjusting the solvent has been demonstrated to be an effective approach to controlling the morphologies of assembly micelles.33,34 Therefore, we studied the assembly behavior in solvents with varied polarity in detail (Fig. 5, S12 and S13†). The assembly of BCPs in methanol whose polarity is much greater has been discussed above and 2 other less polar solvents including isopropanol and isobutanol are chosen as selective solvents. For PPV7-b-P2VP25, which grows up to mixed micelles dominated by 2D scarves in methanol, it ultimately forms 1D fibers in isopropanol and isobutanol (Fig. 5a–c). For PPV7-b-P2VP18, which exhibits 3D complex supermicelles in methanol (Fig. 5d), ill-organized 2D nanoribbons are formed in less polar isopropanol (Fig. 5e). Besides this, it is obvious that the 2D tendency of such nanoribbons is much weaker than that of flower petals in methanol. Then, in much less polar isobutanol, there are only 1D fibrous micelles (Fig. 5f). Finally, PPV7-b-P2VP10, which grows into inky flower-like supermicelles in methanol (Fig. 5g), still assembles into 3D flower-like supermicelles in isopropanol, but the difference is that these flowers are much smaller and the petals are thinner, which shows that they have weaker 2D properties (Fig. 5h). When isobutanol is used, the final micelles are 2D irregular nanoribbons (Fig. 5i). Therefore, the morphological transformation from 3D supermicelles to 2D nanoribbons and 1D nanofibers is successfully achieved.
Such solvent effects are contributed to the kinetic effect. When the polarity of solvent is greater, the solubility of BCPs is much worse, the kinetic behavior of the BCPs is stronger, and so the BCPs aggregate rapidly. On the other hand, in less polar solvents, the solubility is much better so BCPs move more slowly and have enough time to adjust their own alignment. This decrease in dynamic behavior drives the dimensional and morphological transformation.
Conclusions
In summary, uniform hierarchical supermicelles with an inky flower-like morphology at the micron scale have been successfully realized. The growth processes of the micelles from flower heart to petals are observed intuitively by TEM. Then, the UV-Vis absorption spectra of the BCPs illustrate that the driving force of the micelles’ formation primarily depends on the H-aggregation coming from the PPV7 block. Furthermore, the dimensional and morphological transformation among 3D hierarchical supermicelles, 2D ribbons and 1D fibres could be achieved through tuning the polarity of the selective solvents. This work offers an effective strategy to construct hierarchical superstructures from the functional π-conjugated system, which can broaden the range of the micelles’ morphologies and facilitate the investigation of potential applications.
Conflicts of interest
There are no conflicts to declare.
Acknowledgements
F. H. is thankful for the financial support from the National Natural Science Foundation of China (21733005, 21975115, 51773087), the Natural Science Foundation of Guangdong Province (2016A030313637), the Shenzhen Fundamental Research program (JCYJ20170817111214740 and KQJSCX20180319114442157), the Shenzhen Nobel Prize Scientists Laboratory Project (C17213101), the Guangdong Innovative and the Entrepreneurial Research Team Program under contract no. 2016ZT06G587. We would like to thank the SUSTech Core Research Facilities, Life Science Platform and Cryo-EM Center for our transmission electron microscopy work.
Notes and references
- D. Philp and J. F. Stoddart, Angew. Chem., Int. Ed. Engl., 1996, 35, 1154–1196 CrossRef.
- Y. Y. Mai and A. Eisenberg, Chem. Soc. Rev., 2012, 41, 5969–5985 RSC.
- F. H. Schacher, P. A. Rupar and I. Manners, Angew. Chem., Int. Ed., 2012, 51, 7898–7921 CrossRef CAS.
- F. He, T. Gaedt, I. Manners and M. A. Winnik, J. Am. Chem. Soc., 2011, 133, 9095–9103 CrossRef CAS.
- L. Han, M. Wang, X. Jia, W. Chen, H. Qian and F. He, Nat. Commun., 2018, 9, 865 CrossRef.
- X. M. He, M. S. Hsiao, C. E. Boott, R. L. Harniman, A. Nazemi, X. Y. Li, M. A. Winnik and I. Manners, Nat. Mater., 2017, 16, 481–489 CrossRef CAS.
- J. B. Gilroy, T. Gadt, G. R. Whittell, L. Chabanne, J. M. Mitchels, R. M. Richardson, M. A. Winnik and I. Manners, Nat. Chem., 2010, 2, 566–570 CrossRef CAS.
- B. Sharma, A. Mahata, S. Mandani, N. Thakur, B. Pathak and T. K. Sarma, New J. Chem., 2018, 42, 17983–17990 RSC.
- B. I. Kharisov, Recent Pat. Nanotechnol., 2008, 2, 190–200 CrossRef CAS.
- D. D. Majumder, R. Banerjee, C. Ulrichs, I. Mewis and A. Goswami, IETE Tech. Rev., 2007, 24, 9–25 Search PubMed.
- C. Yang, F. Xiao, J. D. Wang and X. T. Su, Sens. Actuators, B, 2015, 207, 177–185 CrossRef CAS.
- C. P. Gu, X. J. Xu, J. R. Huang, W. Z. Wang, Y. F. Sun and J. H. Liu, Sens. Actuators, B, 2012, 174, 31–38 CrossRef CAS.
- Q. Z. Huang, Y. Y. Fang, J. F. Shi, Y. L. Liang, Y. Q. Zhu and G. Xu, ACS Appl. Mater. Interfaces, 2017, 9, 36431–36437 CrossRef CAS.
- S. W. Lee, S. A. Cheon, M. I. Kim and T. J. Park, J. Nanobiotechnol., 2015, 13, 54 CrossRef.
- Z. H. Nie, A. Petukhova and E. Kumacheva, Nat. Nanotechnol., 2010, 5, 15–25 CrossRef CAS PubMed.
- L. Jia, G. Zhao, W. Shi, N. Coombs, I. Gourevich, G. C. Walker, G. Guerin, I. Manners and M. A. Winnik, Nat. Commun., 2014, 5, 3882 CrossRef CAS.
- H. Wang, W. J. Lin, K. P. Fritz, G. D. Scholes, M. A. Winnik and I. Manners, J. Am. Chem. Soc., 2007, 129, 12924–12925 CrossRef CAS.
- S. F. M. Yusoff, J. B. Gilroy, G. Cambridge, M. A. Winnik and I. Manners, J. Am. Chem. Soc., 2011, 133, 11220–11230 CrossRef CAS.
- L. Jia, G. Guerin, Y. J. Lu, Q. Yu, I. Manners and M. A. Winnik, Angew. Chem., Int. Ed., 2018, 57, 17205–17210 CrossRef CAS.
- F. He, T. Gaedt, M. Jones, G. D. Scholes, I. Manners and M. A. Winnik, Macromolecules, 2009, 42, 7953–7960 CrossRef CAS.
- H. B. Qiu, G. Russo, P. A. Rupar, L. Chabanne, M. A. Winnik and I. Manners, Angew. Chem., Int. Ed., 2012, 51, 11882–11885 CrossRef CAS.
- A. C. Kamps, M. Fryd and S. J. Park, ACS Nano, 2012, 6, 2844–2852 CrossRef CAS PubMed.
- Y. Wang, X. F. Liu, J. Peng and F. Qiu, RSC Adv., 2015, 5, 107970–107976 RSC.
- D. W. Hayward, D. J. Lunn, A. Seddon, J. R. Finnegan, O. E. C. Gould, O. Magdysyuk, I. Manners, G. R. Whittell and R. M. Richardson, Macromolecules, 2018, 51, 3097–3106 CrossRef CAS.
- J. Gwyther, J. B. Gilroy, P. A. Rupar, D. J. Lunn, E. Kynaston, S. K. Patra, G. R. Whittell, M. A. Winnik and I. Manners, Chem. – Eur. J., 2013, 19, 9186–9197 CrossRef CAS.
- D. Tao, C. Feng, Y. Cui, X. Yang, I. Manners, M. A. Winnik and X. Huang, J. Am. Chem. Soc., 2017, 139, 7136–7139 CrossRef CAS.
- D. L. Tao, C. Feng, Y. J. Lu, Y. N. Cui, X. Yang, I. Manners, M. A. Winnik and X. Y. Huang, Macromolecules, 2018, 51, 2065–2075 CrossRef CAS.
- C. Feng, M. J. Gonzalez-Alvarez, Y. Song, I. Li, G. Y. Zhao, G. Molev, G. Guerin, G. Walker, G. D. Scholes, I. Manners and M. A. Winnik, Soft Matter, 2014, 10, 8875–8887 RSC.
- M. E. Robinson, A. Nazemi, D. J. Lunn, D. W. Hayward, C. E. Boott, M. S. Hsiao, R. L. Harniman, S. A. Davis, G. R. Whittell, R. M. Richardson, L. De Cola and I. Manners, ACS Nano, 2017, 11, 9162–9175 CrossRef CAS.
- Z. M. Hudson, C. E. Boott, M. E. Robinson, P. A. Rupar, M. A. Winnik and I. Manners, Nat. Chem., 2014, 6, 893–898 CrossRef CAS.
- J. R. Finnegan, X. M. He, S. T. G. Street, J. D. Garcia-Hernandez, D. W. Hayward, R. L. Harniman, R. M. Richardson, G. R. Whittell and I. Manners, J. Am. Chem. Soc., 2018, 140, 17127–17140 CrossRef CAS PubMed.
- F. C. Spano and C. Silva, Annu. Rev. Phys. Chem., 2014, 65, 477–500 CrossRef CAS.
- G. F. Liu, J. H. Sheng, W. L. Teo, G. B. Yang, H. W. Wu, Y. X. Li and Y. L. Zhao, J. Am. Chem. Soc., 2018, 140, 16275–16283 CrossRef CAS.
- H. B. Qiu, Y. Gao, C. E. Boott, O. E. C. Gould, R. L. Harniman, M. J. Miles, S. E. D. Webb, M. A. Winnik and I. Manners, Science, 2016, 352, 697–701 CrossRef CAS PubMed.
Footnote |
† Electronic supplementary information (ESI) available. See DOI: 10.1039/c9py01625b |
|
This journal is © The Royal Society of Chemistry 2020 |
Click here to see how this site uses Cookies. View our privacy policy here.