DOI:
10.1039/C9QI00948E
(Research Article)
Inorg. Chem. Front., 2020,
7, 108-116
Controlled synthesis of flower-like cobalt phosphate microsheet arrays supported on Ni foam as a highly efficient 3D integrated anode for non-enzymatic glucose sensing†
Received
30th July 2019
, Accepted 13th October 2019
First published on 15th October 2019
Abstract
Cobalt phosphate (CoPO) is gaining increasing interest as an alternative to noble metals in electrocatalytic applications. Here, we report the controlled synthesis of flower-like CoPO microsheet arrays supported on Ni foam (CoPO MA/NF) via a simple hydrothermal reaction, and their direct use as a 3D integrated anode for non-enzymatic glucose sensing. The catalytic activity of CoPO MA/NF towards glucose oxidation was evaluated by electrochemical tests. Results show that CoPO MA/NF displays high performance for glucose sensing with a short response time of less than 10 s, a low detection limit of 1 μM (S/N = 3), and a high sensitivity of 3.55 mA mM−1 cm−2. In addition, CoPO MA/NF shows excellent selectivity in the presence of interfering agents such as dopamine, uric acid and ascorbic acid, which makes it reliable for glucose detection in real human serum samples. This work not only provides the controlled synthesis of a CoPO 3D integrated electrode by a simple process, but also explores the potential application of the CoPO-based electrode material for electrochemical sensing of biochemical analytes.
1. Introduction
In view of super stability and simple operation, non-enzymatic glucose electrochemical sensors have attracted enormous attention in the past few decades.1–3 Noble metals including Pt,4 Au,5 Pd,6 and their alloys7–9 show remarkable catalytic activity towards non-enzymatic glucose oxidation, but they are expensive and always suffer from the poisoning of intermediates and chloride ions. Therefore, transition metals such as Cu,10–12 Co,13–15 Mn16,17 and Ni13,18–20 are gaining increasing interest as promising alternatives to noble metals in glucose sensing, due to their low-cost and abundance in nature.
Cobalt and its compounds are an exciting family of such non-enzymatic glucose oxidation electrocatalysts that have received great attention, including Co3O4,15,21,22 Co(OH)2,23–25 CoS,26,27 CoSe,28 Co3N,13,29 CoP,30–32 Co–Bi,33etc. These materials were reported with excellent catalytic activity towards glucose electro-oxidation; however, most of them need a long preparation time or an extra immobilization process before an electrochemical test. To address such issues, a 3D integrated electrode with an attractive architecture34,35 was introduced, which not only provides a large active surface area and more exposed active sites, but also avoids the tedious immobilization process.
Cobalt phosphate (CoPO) has been recently explored and applied in the area of electrochemical energy storage36,37 and conversion38,39 due to its high electrochemical activities. Encouraged by such remarkable electrocatalytic activity of CoPO, Tomanin et al.40 have recently developed CoPO nanostructures as electrocatalysts for non-enzymatic glucose sensing at physiological pH, but this sensor shows lower catalytic activity with a sensitivity of 7.9 nA mM−1 cm−2 and a low detection limit of 0.3 mM, and its preparation requires a complex immobilization process with the use of a polymer binder as a film-forming agent. The use of a polymer binder was considered to be responsible for the generally increased series resistance, reduced active sites and decreased catalytic activity.39 Therefore, it is extremely important to construct a CoPO 3D integrated electrode to boost its performance in non-enzymatic glucose sensing, which, however, has not been explored so far.
In this paper, we report the controlled synthesis of CoPO and cobalt hydrogen phosphate (CoHPO) microsheet arrays supported on Ni foam (CoPO MA/NF and CoHPO MA/NF, respectively) via a simple hydrothermal reaction, and the their use as a 3D integrated anode in catalyzing glucose electro-oxidation. Compared to CoHPO MA/NF, CoPO MA/NF shows a wider linear detection range and higher stability in alkaline media and can be used as a promising 3D integrated electrode in enzyme-free glucose sensing. Under the optimal potential, CoPO MA/NF shows high efficiency towards glucose sensing, with a sensitivity of 3.55 mA mM−1 cm−2 and a low detection limit of 1 μM. In addition, CoPO MA/NF exhibits excellent selectivity against common interferents such as dopamine (DA), uric acid (UA) and ascorbic acid (AA), and good reliability for glucose analysis in real human serum samples, as well as excellent long-term durability for storage. All these highlight the potential use of CoPO MA/NF as a promising 3D integrated electrode for the sensitive detection of biochemical analytes.
2. Experimental section
Materials
Ni foam (NF) was purchased from Kunshan Kuang Xun Electronics Co. Ltd, NaOH and ethanol were purchased from Chongqing Chuandong Chemical Group Co. Ltd, CoCl2·6H2O was obtained from Chengdu Cologne Chemical Co. Ltd and NaH2PO4·2H2O was supplied by Chongqing Beibei Chemical Reagent Factory. Ascorbic acid (AA), uric acid (UA), dopamine (DA) and D-(+)-glucose were supplied by J&K Chemical Ltd (China). All aqueous solutions were prepared with deionized water. Human serum samples were kindly supplied by the Chongqing Emergency Medical Central and used without further purification. The sample analysis experimental protocol was approved by the University Ethical Committee of Chongqing Medical University, and their guidelines were followed throughout this study. Additionally, the sample for glucose analysis was approved by the blood donor volunteer.
Preparation of CoPO MA/NF and CoHPO MA/NF
Prior to synthesis, a piece of NF (1 cm × 2 cm) was washed with 3 M HCl, ethanol and deionized water several times to ensure that the surface of Ni foam was well cleaned. To prepare CoPO MA/NF, 2 mmol CoCl2·6H2O was dissolved in 50 mL deionized water under vigorous stirring, followed by the addition of 2.3 mmol NaH2PO4·2H2O, obtaining 40.0 mM CoCl2 and 46.0 mM NaH2PO4 mixed solution as the reactant. After 10 min of stirring, 18 mL of the mixed solution was transferred to a 25 mL Telfon-lined stainless steel autoclave and the cleaned NF was submerged in this solution. After this, the autoclave was sealed and maintained at 120 °C for 24 h and then allowed to cool down to room temperature. The resulting material was washed with ethanol and water, respectively, and dried at 60 °C for 12 h to obtain CoPO MA/NF. The loading of CoPO MA on NF was determined to be 8.42 mg cm−2 using a high precision microbalance.
CoHPO MA/NF was prepared by the same method as CoPO MA/NF except for the use of 20 mL instead of 50 mL deionized water to dissolve 2 mmol CoCl2·6H2O and 2.3 mmol NaH2PO4·2H2O. The concentration of CoCl2 and NaH2PO4 was 100 and 115 mM, respectively.
Characterization
X-ray diffraction (XRD) data were acquired on a RigakuD/MAX 2550 diffractometer with Cu Kα radiation (λ = 1.5418 Å). Scanning electron microscopy (SEM) images were acquired on a field-emission scanning electron microscope (FE-SEM, JSM-7800) at an accelerating voltage of 6 kV. X-ray photoelectron spectrometry (XPS) measurements were performed on an ESCALABMK II X-ray photoelectron spectrometer using Mg as the excitation source.
Electrochemical tests
All electrochemical measurements were performed on an Autolab PGSTAT 302 electrochemical workstation (Metrohm Ltd, Switzerland). CoPO MA/NF or CoHPO MA/NF (0.5 cm × 0.5 cm) was directly utilized as the working electrode for electrochemical tests, with platinum wire as the counter electrode and a saturated Ag/AgCl electrode as the reference electrode. 0.1 M NaOH aqueous solution was used as the supporting electrolyte. Electrochemical tests were performed at room temperature and all the potentials were reported against the saturated Ag/AgCl reference electrode.
For sample analysis, 10 μL human serum samples without any pretreatment was added into 10 mL 0.1 M NaOH electrolyte solution and the stable values of the amperometric responses were measured at a potential of 0.55 V three times. The glucose concentration of each blood serum sample was calculated based on the amperometric measurements and compared with the values provided by the hospital.
3. Results and discussion
Controlled synthesis of CoPO MA/NF
CoPO MA/NF was prepared through a simple hydrothermal reaction using 40.0 mM CoCl2·6H2O and 46.0 mM NaH2PO4·2H2O as the reactants. Note that CoHPO MA/NF can also be prepared by the same synthesis method as that used for CoPO MA/NF except for the use of an increased amount (2.5-fold) of the reaction precursor (see the Experimental section). Scheme 1 illustrates the controlled synthesis of CoPO and CoHPO MA/NF and their application in catalyzing glucose electro-oxidation.
 |
| Scheme 1 Schematic illustration of the controlled synthesis of CoPO and CoHPO MA/NF and their applications in catalyzing glucose oxidation. | |
Through a hydrothermal reaction using 40 mM CoCl2 and 46 mM NaH2PO4 as precursors, the surface of NF turns dark purple in color (see Fig. S1a and S1b†), implying the presence of some cobalt compounds. Fig. 1a displays the corresponding X-ray diffraction (XRD) patterns of this purple product supported on NF. As shown, all diffraction peaks in the XRD pattern correspond well to the diffraction of Co3(PO4)2·4H2O (JCPDS No. 34-0844), except for three strong peaks (marked with “#”) from NF. No other peaks were observed, indicating that the purple product shouldbe none other than Co3(PO4)2·4H2O (CoPO). Scanning electron microscopy (SEM, Fig. 1b) images show plenty of petaloid CoPO sheets growing vertically on the whole NF framework. The high-magnification SEM images (Fig. 1c and d) further reveal that these CoPO sheets have a micrometer size with a thickness of about 1 μm and a width of about 3 μm, a smooth surface and a sword-like structure, forming flower-like CoPO microsheet arrays supported on the entire NF backbone. It is clearly seen that these flower-like structures are about 100 μm in diameter.
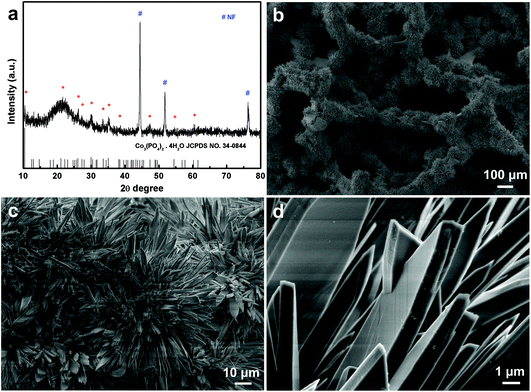 |
| Fig. 1 (a) XRD patterns of CoPO MA/NF. (b) Low- and (c, d) high-magnification SEM images of CoPO MA/NF. | |
It is interesting to note that the increased concentration of the precursor (2.5-fold) (100 and 115 mM for CoCl2 and NaH2PO4, respectively) causes the NF surface to turn pink in color (Fig. S1a and S1c†). This pink product supported on NF was also characterized by XRD. As shown in Fig. 2a, all diffraction peaks were well indexed to the diffraction of (CoHPO4)2·3H2O (JCPDS No. 39-0705) and that of NF (JCPDS No. 04-0850), suggesting that the pink product is composed of only CoHPO. These flower-like CoHPO microsheet arrays (Fig. 2b and c) have a similar morphology to CoPO MA/NF except for the variation of the sheet shape, CoPO microsheet appears sword-shaped, while CoHPO looks more like a rectangular sheet. All these findings indicated the successful controlled synthesis of CoPO and CoHPO MA/NF.
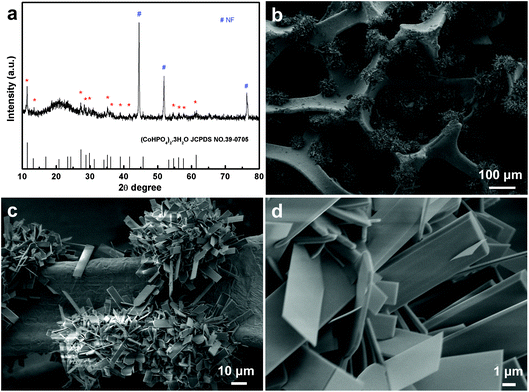 |
| Fig. 2 (a) XRD patterns of CoHPO MA/NF. (b) Low- and (c, d) high-magnification SEM images of CoHPO MA/NF. | |
To further identify the elemental composition and valence state of the obtained CoPO MA/NF, X-ray photoelectron spectroscopy (XPS) analysis was also performed. Fig. 3a presents the XPS survey spectrum of the as-prepared CoPO MA/NF, indicating the presence of the Co, P and O elements. Fig. 3b shows the XPS spectrum in the Co 2p region; the binding energies (BEs) at 781.5 and 797.8 eV are assigned to Co 2p3/2 and Co 2p1/2, respectively, confirming the presence of the Co(II) state.41Fig. 3c shows the XPS spectrum in the P 2p region; the fitted peaks located at 133.1 and 134.0 eV reveal the characteristic P 2p3/2 peaks of P(V).41Fig. 3d shows the XPS spectrum in the O 1s region; the peak at 530.9 eV is assigned to the central oxygen atoms in phosphate,39,40 and those at 531.8 and 532.8 eV are indexed well to the OH species and loosely bound oxygen on the surface of CoPO,40 respectively. All these XPS results agree well with the result of XRD in Fig. 1a.
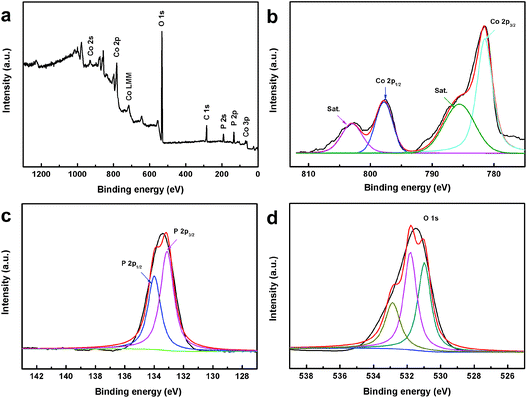 |
| Fig. 3 (a) XPS survey spectra of CoPO MA/NF. (b–d) XPS spectra of Co 2p, P 2p and O 1s, respectively, for CoPO MA/NF. | |
Additionally, the survey and high-resolution XPS spectra of CoHPO MA were also conducted to ascertain the valence state of the elements (Fig. S3†). The survey spectrum displayed Co, P and O signals further confirming the formation of CoHPO MA. The deconvoluted high-resolution spectra of Co 2p, P 2p and O 1s were in good agreement with the previous reports,42 demonstrating the successful controlled synthesis of CoHPO MA/NF.
Electrochemical behavior of CoPO MA/NF
The electrochemical behavior of CoPO MA/NF was investigated using cyclic voltammetry (CV) in 0.1 M NaOH solution. As shown in Fig. 4a, NF causes a weak current response in 0.1 M NaOH at a scan rate of 20 mV s−1 in the potential range from 0 to 0.8 V, while CoPO MA/NF displays a notable current response, indicating high electrochemical activity of CoPO MA rather than NF. In the absence of glucose, the anodic oxidation current increases at about 0.35 V and a well-defined anodic peak was observed at 0.59 V, which could be assigned to the oxidation of Co(II) and Co(III), respectively.30 In the presence of 1.0 mM glucose, the anodic peak current density at 0.59 V was found to increase while the cathodic peak current density at 0.53 V decreased, demonstrating good catalytic activity of CoPO MA/NF for glucose oxidation. Since the current related to the redox couple Co(II)/CO(III) at 0.35 V remains nearly constant while that related to the Co(III)/Co(IV) couple at 0.59 V shows a notable increase in current with the addition of 1.0 mM glucose, it is reasonable to conclude that the electro-oxidation of glucose is mainly catalyzed by Co(III)/Co(IV) rather than Co(II)/CO(III) (Fig. 4b). The mechanism may be expressed as follows: | Co(IV) + glucose → Co(III) + gluconolactone | (3) |
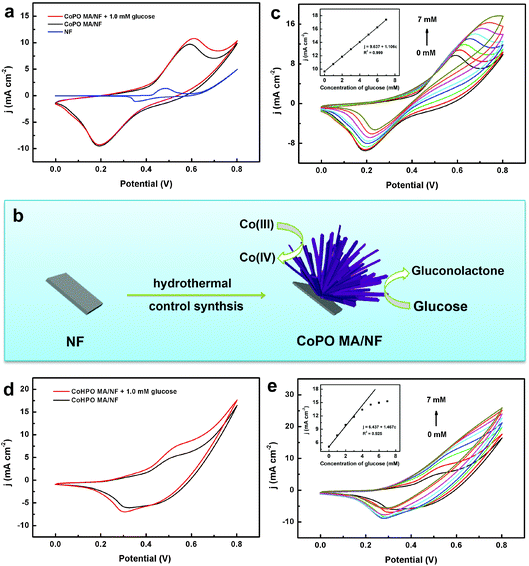 |
| Fig. 4 (a) CV curves of NF, CoPO MA/NF in the absence and presence of 1.0 mM glucose in 0.1 M NaOH solution. (b) The mechanism of CoPO MA/NF in catalyzing glucose oxidation. (c) CV curves of CoPO MA/NF in the presence of different glucose concentrations (from 0 to 7.0 mM) with a scan rate of 20 mV s−1. The inset shows the plots of anodic peak current density vs. glucose concentration. (d) CV curves of CoHPO MA/NF in the absence and presence of 1.0 mM glucose in 0.1 M NaOH solution. (e) CV curves of CoHPO MA/NF in the presence of different glucose concentrations (from 0 to 7.0 mM) with a scan rate of 20 mV s−1. The inset shows the plots of anodic peak current density vs. glucose concentration. | |
Fig. 4c shows the CV curves of CoPO MA/NF in 0.1 M NaOH with different concentrations of glucose at a scan rate of 20 mV s−1 within a potential range from 0 to 0.8 V. The increased glucose concentration leads to enhanced current density of the anodic peak with an insignificant potential shift. The corresponding calibration curve (inset) exhibits a good linearity between peak current densities and increasing glucose concentrations from 0 to 7.0 mM.
For comparison, the electrochemical behavior of CoHPO MA/NF was also investigated. As shown in Fig. 4d, CoHPO MA/NF displays two obvious anodic peaks at 0.4 and 0.56 V, and both of them increased in the presence of 1.0 mM glucose, suggesting its catalytic activity towards glucose oxidation. However, it suffers from narrower linearity (0 to 3.0 mM) between the peak current densities and increasing glucose concentrations (Fig. 4e and inset). The inferior performance of CoHPO MA/NF may be ascribed to its insufficient stability in alkaline media. As observed in Fig. S2,† the surface of CoHPO MA/NF changes from pink to black after ten CV cycles. According to a previous report,42 this black substance should be mainly attributed to the electrochemical transformation of Co(II) to Co3O4. In contrast, the surface of CoPO MA/NF maintains its dark purple color after ten CV cycles. All these results demonstrated the superior electrocatalytic oxidation activity of CoPO MA/NF towards glucose compared to CoHPO MA/NF.
To find out the kinetics of glucose oxidation, we studied the CV behavior of CoPO MA/NF at different scan rates in 0.1 M NaOH in the presence of 1.0 mM glucose. As observed in Fig. 5a, both anodic and cathodic peak current densities increase with increasing scan rates ranging from 10 to 100 mV s−1. Fig. 5b demonstrates a good linear relationship between the anodic and cathodic peak current densities and the scan rates, implying a typical absorption-controlled process of glucose oxidation on CoPO MA/NF.
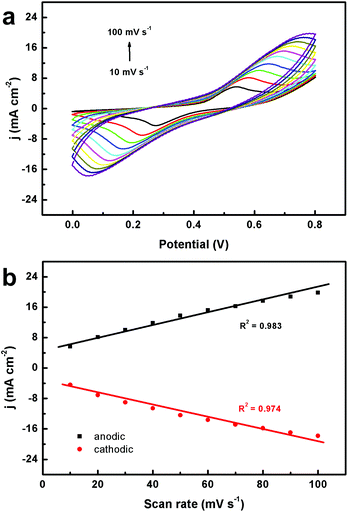 |
| Fig. 5 (a) CVs of CoPO MA/NF in the presence of 1.0 mM glucose at various scan rates of 10, 20, 30, 40, 50, 60, 70, 80, 90, and 100 mV s−1. (b) The corresponding plots of the current density vs. the scan rate. | |
Amperometric detection of glucose
To achieve a sensitive signal, the optimal operation potential for glucose sensing was investigated. Fig. 6a shows the amperometric current response of CoPO MA/NF in 0.1 M NaOH with the continuous addition of 0.1 mM glucose at different potentials (from 0.50 to 0.65 V). Stable and rapid changes in the current response were observed with the increase in the applied potential, and the biggest response was obtained when the applied potential was set at 0.55 V. Therefore, 0.55 V was selected as the optimum potential in the following experiments.
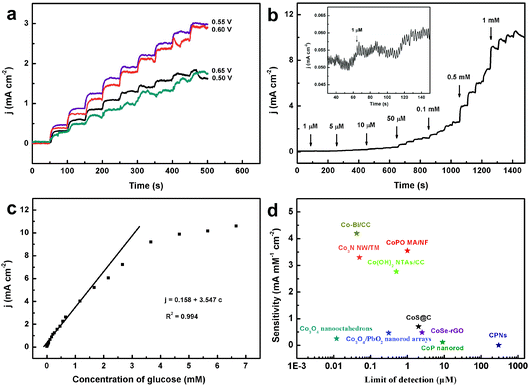 |
| Fig. 6 (a) Amperometric responses of CoPO MA/NF at different potentials (from 0.50 to 0.65 V) with the continuous addition of 0.1 mM glucose in 0.1 M NaOH. (b). Amperometric response of CoPO MA/NF with successive addition of glucose in 0.1 M NaOH. The inset shows the current response of CoPO MA/NF toward the addition of 1 μM glucose. (c) Calibration curve for current response to glucose concentration. (d) Comparison of the sensing performance of CoPO MA/NF and other Co-based enzyme-free glucose electrochemical sensors. | |
The amperometric response of CoPO MA/NF with successive addition of different glucose concentrations was measured as shown in Fig. 6b. A well-defined and stable amperometric response can be observed by successive additions of glucose, and a 95% steady-state oxidation current is obtained in less than 10 s (inset), suggesting a fast glucose detection. The response currents display a linear variation with increasing concentration of glucose over the range from 1 μM to 1.16 mM (Fig. 6c). The response sensitivity and the low detection limit (S/N = 3) were estimated to be 3.55 mA mM−1 cm−2 and 1 μM (Fig. 6b inset), respectively. Such performances compare favorably to most of the reported Co-based enzyme-free glucose sensors (Fig. 6d), such as Co3O4 nanooctahedra,15 Co3O4/PbO2 nanorod arrays,22 Co(OH)2 NTAs/CC,23 CoS@C,26 CoSe-rGO,28 Co3N NW/TM,29 CoP nanorods,31 Co–Bi/CC,33etc. Notably, the sensitivity of the present sensor is several thousand times higher than that of the cobalt phosphate nanostructure (CPN) sensor at physiological pH with a sensitivity of 7.9 nA mM−1 cm−2.40 The superior performance of CoPO MA/NF for glucose sensing may be attributed to the high catalytic activity of CoPO MA and its 3D integrated nanoarray architecture.
Anti-interference and anti-poisoning capacity of CoPO MA/NF
Anti-interference capacity is an important parameter in evaluating the performance of a glucose sensor. Some electrochemically active substances such as dopamine (DA), uric acid (UA) and ascorbic acid (AA) usually co-exist with glucose and always interfere with glucose detection in the human blood. Fig. 7a shows the amperometric responses of CoPO MA/NF towards consecutive introduction of 0.1 mM glucose and 0.01 mM DA, AA and UA in 0.1 M NaOH. As observed, CoPO MA/NF shows remarkable response for glucose but negligible response for the interfering species and the current density increases again with the addition of glucose. All these results suggest that CoPO MA/NF exhibits excellent selectivity against common interfering species.
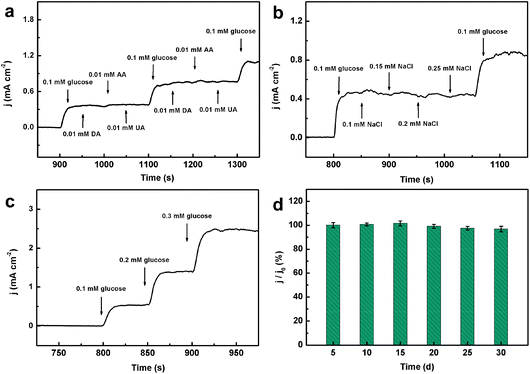 |
| Fig. 7 (a) Amperometric responses of CoPO MA/NF toward the addition of 0.1 mM glucose and 0.01 mM various interfering substances (DA, AA and UA) in 0.1 M NaOH. (b). Amperometric response of CoPO MA/NF toward 0.1 mM glucose and various amounts of chloride ions. (c). Amperometric response of CoPO MA/NF toward 0.1, 0.2 and 0.3 mM glucose in a 1000-fold diluted human serum sample. (d). The histogram of the current response of CoPO MA/NF toward 1.0 mM glucose in 0.1 M NaOH for 30 days. | |
Furthermore, the anti-poisoning capacity of CoPO MA/NF against chloride ions was also investigated. Fig. 7b presents the amperometric response of CoPO MA/NF towards 0.1 mM glucose and various amounts of chloride ions ranging from 0.10 to 0.25 mM in 0.1 M NaOH. It was clearly seen that 0.1 mM glucose causes a notable increase in the current density response, while chloride ions show a negligible current response change even at 0.25 mM, and the current density increases again with the addition of glucose. These results demonstrate the excellent anti-poisoning capacity of the present CoPO MA/NF sensor.
Reliability and stability
The reliability of the present sensor was also evaluated by assessing the recovery of glucose in human serum samples. Here, 1000-fold diluted human serum samples in 0.1 M NaOH was used as the electrolyte and the corresponding amperometric response was taken as the current baseline. As shown in Fig. 7c, the addition of glucose causes a notable amperometric response. The recovery of 0.1, 0.2 and 0.3 mM glucose was found to be 98.9%, 104.2% and 98.7%, respectively (Table S1†). Each sample has been tested three times and the relative standard deviations (RSD) were less than 3.02%. These results indicated excellent reliability and repeatability of the present sensor for glucose detection in human serum samples. Additionally, the long-term stability of CoPO MA/NF was also tested by recording the current response towards 1.0 mM glucose every five days for 30 days. As shown in Fig. 7d, CoPO MA/NF retains approximately 96.0% of its initial current response after 30 days storage, implying good long-term stability.
Practical application
To assess its feasibility for routine analysis, CoPO MA/NF was applied for glucose detection in human serum samples which was kindly supplied by the Chongqing Emergency Medical Centre. Fig. 8 shows the amperometric responses of CoPO MA/NF with the introduction of 10 μL glucose (0.01 M) and 10 μL human serum samples into 10 mL of 0.1 M NaOH. The glucose concentration of each blood serum sample was calculated by comparing their current densities and all measurements were carried out three times. Table S2† lists the glucose concentrations of each serum sample determined by CoPO MA/NF and that provided by the hospital. As is shown, the bias and RSD value observed were less than 0.10 mM and 2.26%, respectively, suggesting good reliability and high precision of glucose determination by CoPO MA/NF. All the above indicate that CoPO MA/NF is promising for practical analysis.
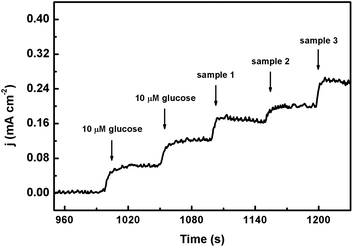 |
| Fig. 8 Amperometric response of CoPO MA/NF with the addition of 10 μM glucose and three serum samples into 0.1 M NaOH solution. | |
4. Conclusions
In summary, CoPO MA/NF was synthesized in a controlled way by a simple hydrothermal reaction. Compared to CoHPO MA/NF, CoPO MA/NF exhibits superior catalytic activity towards glucose oxidation including a wider linear range and higher stability. As a 3D integrated anode for non-enzymatic glucose detection, CoPO MA/NF shows high sensitivity, excellent selectivity and good reliability, and it was suitable for routine glucose analysis. This work not only offers a controlled method for the preparation of CoPO MA/NF by a simple process, but also explores a novel Co-based 3D integrated electrode for non-enzymatic glucose sensing.
Conflicts of interest
The authors declare no conflict of interest.
Acknowledgements
This work was supported by the Natural Science Foundation of China (Grant No. 21601022), the Livelihoods Science and Technology Innovation Project of Chongqing (cstc2016shmszx20004), the Foundation and Cutting-Edge Research Plan of Chongqing (cstc2018jcyjAX0698 and cstc2019jcyj-msxmX0528).
Notes and references
- A. S. Nugraha, C. Li, J. Bo, M. Iqbal, S. M. Alshehri, T. Ahamad, V. Malgras, Y. Yamauchi and T. Asahi, ChemElectroChem, 2017, 4, 2571–2576 CrossRef CAS.
- D. W. Hwang, S. Lee, M. Seo and T. D. Chung, Anal. Chim. Acta, 2018, 1033, 1–34 CrossRef CAS.
- H. Zhu, L. Li, W. Zhou, Z. Shao and X. Chen, J. Mater. Chem. B, 2016, 4, 7333–7349 RSC.
- X. Fu, Z. Chen, S. Shen, L. Xu and Z. Luo, Int. J. Electrochem. Sci., 2018, 13, 4817–4826 CrossRef CAS.
- N. Verma, Appl. Surf. Sci., 2018, 462, 753–759 CrossRef CAS.
- F. Wang, X. Niu, W. Wang, W. Jing, Y. Huang and J. Zhang, J. Taiwan Inst. Chem. Eng., 2018, 93, 87–93 CrossRef CAS.
- S. Nantaphol, T. Watanabe, N. Nomura, W. Siangproh, O. Chailapakul and Y. Einaga, Biosens. Bioelectron., 2017, 98, 76–82 CrossRef CAS PubMed.
- J. Yang, X. Liang, L. Cui, H. Liu, J. Xie and W. Liu, Biosens. Bioelectron., 2016, 80, 171–174 CrossRef CAS.
- Y. Zhao, L. Fan, B. Hong, J. Ren, M. Zhang, Q. Que and J. Ji, Sens. Actuators, B, 2016, 231, 800–810 CrossRef CAS.
- M. Figiela, M. Wysokowski, M. Galinski, T. Jesionowski and I. Stepniak, Sens. Actuators, B, 2018, 272, 296–307 CrossRef CAS.
- L. Y. Lin, B. B. Karakocak, S. Kavadiya, T. Soundappan and P. Biswas, Sens. Actuators, B, 2018, 259, 745–752 CrossRef CAS.
- C. Yu, J. Cui, Y. Wang, H. Zheng, J. Zhang, X. Shu, J. Liu, Y. Zhang and Y. Wu, Appl. Surf. Sci., 2018, 439, 11–17 CrossRef CAS.
- X. Dai, W. Deng, C. You, Z. Shen, X. Xiong and X. Sun, Anal. Methods, 2018, 10, 1680–1684 RSC.
- T. Wang, L. Xi and J. Wang, Talanta, 2018, 178, 481–490 CrossRef CAS.
- J. Xu, F. Li, D. Wang, M. H. Nawaz, Q. An, D. Han and L. Niu, Biosens. Bioelectron., 2019, 123, 25–29 CrossRef CAS.
- M. Li, L. Liu, Y. Xiong, X. Liu, A. Nsabimana, X. Bo and L. Guo, Sens. Actuators, B, 2015, 207, 614–622 CrossRef CAS.
- S. Yang, G. Li, G. Wang, J. Zhao, X. Gao and L. Qu, Sens. Actuators, B, 2015, 221, 172–178 CrossRef CAS.
- N. I. Chandrasekaran and M. Matheswaran, Sens. Actuators, B, 2019, 288, 188–194 CrossRef CAS.
- W. C. Lee, K. B. Kim, N. G. Gurudatt, K. K. Hussain, C. S. Choi, D. S. Park and Y. B. Shim, Biosens. Bioelectron., 2019, 130, 48–54 CrossRef CAS.
- L. Tian, G. He, M. Chen, J. Wang, Y. Yao and X. Bai, Nanoscale Res. Lett., 2018, 13, 342 CrossRef.
- J. Yu, Y. Ni and M. Zhai, J. Alloys Compd., 2017, 723, 904–911 CrossRef CAS.
- T. Chen, X. Li, C. Qiu, W. Zhu, H. Ma, S. Chen and O. Meng, Biosens. Bioelectron., 2014, 53, 200–206 CrossRef CAS.
- M. Huang, X. Luo, D. He and P. Jiang, Anal. Methods, 2017, 9, 5903–5909 RSC.
- D. Jiang, Z. Chu, J. Peng, J. Luo, Y. Mao, P. Yang and W. Jin, Electrochim. Acta, 2018, 270, 147–155 CrossRef CAS.
- I. Shackery, U. Patil, A. Pezeshki, N. M. Shinde, S. Im and S. C. Jun, Microchim. Acta, 2016, 183, 2473–2479 CrossRef CAS.
- P. Qu, Z. Gong, H. Cheng, W. Xiong, X. Wu, P. Pei, R. Zhao, Y. Zeng and Z. Zhu, RSC Adv., 2015, 5, 106661–106667 RSC.
- W. Wu, B. Yu, H. Wu, S. Wang, Q. Xia and Y. Ding, Mater. Sci. Eng., C, 2017, 70, 430–437 CrossRef CAS.
- M. C. D. Cooray, X. Zhang, Y. Zhang, S. J. Langford, A. M. Bond and J. Zhang, J. Mater. Chem. A, 2017, 5, 19289–19296 RSC.
- F. Xie, X. Cao, F. Qu, A. M. Asiri and X. Sun, Sens. Actuators, B, 2018, 255, 1254–1261 CrossRef CAS.
- Y. Liu, X. Cao, R. Kong, G. Du, A. M. Asiri, Q. Lu and X. Sun, J. Mater. Chem. B, 2017, 5, 1901–1904 RSC.
- Q. Q. Sun, M. Wang, S. J. Bao, Y. C. Wang and S. Gu, Analyst, 2016, 141, 256–260 RSC.
- Z. Wang, X. Cao, D. Liu, S. Hao, G. Du, A. M. Asiri and X. Sun, Chem. Commun., 2016, 52, 14438–14441 RSC.
- W. Deng, R. Dai, C. You, P. Hu, X. Sun, X. Xiong, K. Huang and F. Huo, ChemistrySelect, 2018, 3, 10580–10584 CrossRef CAS.
- Y. Li, M. Xie, X. Zhang, Q. Liu, D. Lin, C. Xu, F. Xie and X. Sun, Sens. Actuators, B, 2019, 278, 126–132 CrossRef CAS.
- Z. Wei, W. Zhu, Y. Li, Y. Ma, J. Wang, N. Hu, Y. Suo and J. Wang, Inorg. Chem., 2018, 57, 8422–8428 CrossRef CAS.
- B. Li, P. Gu, Y. Feng, G. Zhang, K. Huang, H. Xue and H. Pang, Adv. Funct. Mater., 2017, 27, 1605784 CrossRef.
- H. Mao, F. Zhang, X. Liu, J. Qiu, B. Li and Z. Jin, J. Mater. Sci.: Mater. Electron., 2018, 29, 16721–16729 CrossRef CAS.
- C. Z. Yuan, Y. F. Jiang, Z. Wang, X. Xie, Z. K. Yang, A. Bin Yousaf and A. W. Xu, J. Mater. Chem. A, 2016, 4, 8155–8160 RSC.
- L. Xie, R. Zhang, L. Cui, D. Liu, S. Hao, Y. Ma, G. Du, A. M. Asiri and X. Sun, Angew. Chem., Int. Ed., 2017, 56, 1064–1068 CrossRef CAS.
- P. P. Tomanin, P. V. Cherepanov, Q. A. Besford, A. J. Christofferson, A. Amodio, C. F. McConville, I. Yarovsky, F. Caruso and F. Cavalieri, ACS Appl. Mater. Interfaces, 2018, 10, 42786–42795 CrossRef CAS.
- Y. Shu, B. Li, J. Chen, Q. Xu, H. Pang and X. Hu, ACS Appl. Mater. Interfaces, 2018, 10, 2360–2367 CrossRef CAS.
- Y. P. Zhu, T. Y. Ma, M. Jaroniec and S. Z. Qiao, Angew. Chem., Int. Ed., 2017, 56, 1324–1328 CrossRef CAS.
Footnote |
† Electronic supplementary information (ESI) available. See DOI: 10.1039/c9qi00948e |
|
This journal is © the Partner Organisations 2020 |
Click here to see how this site uses Cookies. View our privacy policy here.