DOI:
10.1039/C9QI01058K
(Research Article)
Inorg. Chem. Front., 2020,
7, 72-81
Construction of a bifunctional Zn(II)–organic framework containing a basic amine functionality for selective capture and room temperature fixation of CO2†
Received
20th August 2019
, Accepted 19th September 2019
First published on 20th September 2019
Abstract
Selective carbon capture and utilization (CCU) as a C1 feedstock for the synthesis of value-added chemicals under ambient conditions catalyzed by porous MOFs constitutes one of the most promising solutions to mitigate the growing CO2 concentration in the atmosphere. Consequently, the synthesis of a novel 3D, microporous, bifunctional Zn(II)–organic framework, {[Zn2(TDC)2(DATRZ)]·(3H2O)·(DMF)}n (Zn-DAT) (where TDC = 2,5-thiophene dicarboxylate ion and DATRZ = 3,5-diamino-1,2,4-triazole), was achieved using a mixed ligand strategy. Single crystal X-ray structural analysis of the MOF revealed the presence of a 3D microporous structure with two types of 1D channels of dimensions 12.5 × 8.7 Å2 and 7.0 × 4.8 Å2 along the crystallographic c- and b-axes, respectively. The presence of basic –NH2 functionalized pores in Zn-DAT induces a selective adsorption property of CO2 with a high heat of adsorption (Qst) value of 39.5 kJ mol−1 which is supported by a theoretically computed binding energy (BE) of 40.9 kJ mol−1. Interestingly, the Qst value observed for Zn-DAT is about 8 kJ mol−1 higher than that of the analogous MOF {[Zn2(TDC)(TRZ)2]·(DMA)·(MeOH)}n (Zn-TAZ) containing the 1,2,4-triazole (TAZ) linker, which highlights the critical role of –NH2 groups in enhancing the interaction energy for CO2. The significantly high value of Qst can be attributed to the stronger interaction of the acidic CO2 molecule with the basic –NH2 groups present in the 1D channels of the Zn-DAT MOF. Furthermore, the presence of both Lewis acidic Zn2+ ions and basic –NH2 groups resulted in the Zn-DAT MOF as an efficient heterogeneous catalyst for chemical fixation of CO2 into cyclic carbonates under mild conditions at RT. Herein, we report a rare example of porous MOFs for the capture and utilization of CO2 at RT and the influence of basic –NH2 groups on the high value of Qst and catalytic conversion of carbon dioxide.
Introduction
Carbon dioxide (CO2) capture and utilization (CCU) as an abundant, non-toxic, C1 feedstock for the synthesis of valuable chemicals or fuels is one of the most promising solutions to mitigate the growing CO2 concentration in the atmosphere.1,2 To achieve this goal, several approaches have been developed for the functionalization of CO2 into valuable chemicals like polycarbonates, cyclic carbonates, and others by forming C–C, C–N, and C–O bonds.3–5 However, the kinetic inertness and thermodynamic stability of CO2 put a limitation for its conversion under mild conditions.6 Hence, the large-scale synthesis of cyclic carbonates is generally carried out under high temperature and pressure conditions.7 Therefore, it is essential to develop efficient catalytic systems for selective capture and utilization of carbon dioxide under mild conditions at room temperature (RT). To date, various homogeneous catalysts have been developed for conversion of CO2 into cyclic carbonates owing to its 100% atom efficiency generating cyclic carbonates with high yields and selectivities.8 On the other hand, several heterogeneous catalysts including porous MOFs have been developed for the catalytic conversion of CO2.9 In particular, MOFs have attracted considerable attention due to their high surface area and modular nature which facilitate the introduction of a high density of Lewis acidic and basic functionalities.10–12 However, there are a limited number of MOFs reported for efficient carbon dioxide conversion under mild conditions at RT.13 In this direction, we have been attempting to synthesize porous MOFs composed of a high density of Lewis acidic metal ions and basic functionalities suitable for selective capture and utilization of CO2.14 In continuation of our efforts, herein, we report the synthesis of a novel 3D porous MOF, {[Zn2(TDC)2(DATRZ)]·(3H2O)·(DMF)}n (Zn-DAT), constructed by utilizing a rigid ligand, 2,5-thiophene dicarboxylic acid (H2TDC), and an organic linker, 3,5-diamino-1,2,4-triazole (DATRZ), containing basic –NH2 groups. The Zn-DAT MOF possesses a 3D microporous framework structure with two types of 1D channels of dimensions 12.5 × 8.7 Å2 and 7.0 × 4.8 Å2 along the crystallographic c- and b-axes respectively. Furthermore, the presence of basic –NH2 groups induces a selective adsorption property of CO2 with a high heat of adsorption (Qst) value of 39.5 kJ mol−1 which was further supported by a theoretically computed BE of 40.9 kJ mol−1. More interestingly, the Qst value observed for Zn-DAT is about 8 kJ mol−1 higher than that of the analogous MOF {[Zn2(TDC)(TRZ)2]·(DMA)·(MeOH)}n (Zn-TAZ) containing the 1,2,4-triazole (TAZ) linker, which further supports the critical role of –NH2 groups in enhancing the interaction of CO2 with the framework. Furthermore, the presence of Lewis acidic Zn2+ and basic –NH2 groups makes the Zn-DAT MOF an excellent recyclable catalyst for chemical fixation of CO2 to cyclic carbonates under solvent-free mild conditions at RT. Herein, the design and synthesis of a rare example of a porous Zn(II)-MOF exhibiting efficient fixation of CO2 at RT and the influence of –NH2 groups on the BE of CO2 and catalytic activity are demonstrated.
Experimental section
Synthesis of {[Zn2(TDC)2(DATRZ)]·(3H2O)·(DMF)}n (Zn-DAT)
The Zn-DAT MOF was synthesized as follows: 2,5-thiophene dicarboxylic acid (34.4 mg, 0.2 mmol) and 3,5-diamino-1,2,4-triazole (9.9 mg, 0.1 mmol) were mixed in DMF (4 mL) in a 30 mL glass vial. The mixture was sonicated for 15 minutes and DMF (2 mL) solution of Zn(NO)3·6H2O (59.5 mg, 0.2 mmol) was added to the mixture with stirring. The glass vial was sealed with Teflon tape and parafilm and heated at 120 °C for 72 h. Then the vial was cooled down to room temperature slowly to obtain colorless crystals of Zn-DAT which were collected by filtration and washed with DMF thoroughly. Yield: 75%. The phase-purity of the as-synthesised sample was confirmed by powder XRD analysis (Fig. S1†). Elemental analysis calculated (%) for {[Zn2(TDC)2(DATRZ)]·(3H2O)·(DMF)}n (C17H21N6O15S2Zn2): C: 27.43; H: 2.84; N: 11.29; S: 8.62. Found: C: 28.03; H: 2.93; N: 11.91; S: 9.10. FT-IR (KBr, cm−1): 3441(w), 3330(w), 3211(w), 1586(s), 1518(s), 1463(w), 1355(s), 1103(m), 1015(m), 845(w), 804(m), 763(s), 674(m) (Fig. S2†).
The analogous MOF {[Zn2(TDC)(TRZ)2]·(DMA)·(MeOH)}n (Zn-TAZ) was synthesized by following the previously reported procedure.15 The phase purity of the as-synthesised MOF was confirmed by powder XRD analysis (Fig. S3†).
Results and discussion
Structural description of {[Zn2(TDC)2(DATRZ)]·(3H2O)·(DMF)}n (Zn-DAT)
The reaction of Zn(NO)3·6H2O with 2,5-thiophene dicarboxylic acid (H2TDC) and 3,5-diamino-1,2,4-triazole (DATRZ) in DMF at 120 °C for 3 days afforded colorless crystals of Zn-DAT (Scheme 1).
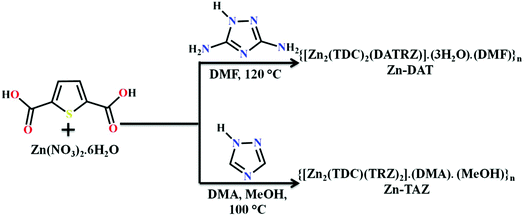 |
| Scheme 1 The synthesis scheme of the Zn-DAT and Zn-TAZ MOFs. | |
X-ray single-crystal structural determination revealed that the compound crystallizes in the monoclinic crystal system with the C2/m space group (Table S1†). The asymmetric unit consists of two distinct binuclear Zn(II) units, two 2,5-thiophene dicarboxylate (TDC) anions and one 3,5-diamino-1,2,4-triazole (DATRZ) linker (Fig. 1). The Zn–O and Zn–N bond lengths are in the range of 1.955–2.031 Å and 1.972–1.993 Å, respectively (Table S2†). The structure shows N–H⋯O hydrogen bonding interactions between the –NH2 groups of the DATRZ ligand and the carboxylate oxygens of the TDC ion (Table S3†). The coordination environment at Zn1 in the [Zn1N1O1]2 binuclear unit is satisfied by two carboxylate oxygen (O1 and O1a, a = 1 − x, y, −z) atoms of the TDC ion and two nitrogen (N1 and N1a, a = 1 − x, y, −z) atoms of the DATRZ linker, whereas the coordination at Zn2 in the Zn2(COO)4 paddle-wheel secondary building unit (SBU) is satisfied by four carboxylate oxygen (O3, O3b, O4 and O4b, b = x, 2 − y, z) atoms of two TDC ligands. As shown in Fig. 2, the Zn(II) ions are bridged by the nitrogen atoms of the DATRZ linker and the carboxylate groups of the TDC ions forming a three-dimensional (3D) microporous framework with two types of 1D channels of dimensions 12.5 × 8.7 Å2 and 7.0 × 4.8 Å2 along the crystallographic c- and b-axes, respectively (Fig. 2 and S4†). Furthermore, the –NH2 groups of the DATRZ linker are free and exposed in the 1D channels of the framework (Fig. 2c). Topological analysis by TOPOS16 suggests that the framework has 6-connected uni-nodal pcu-net topology with a vertex symbol of {4^12·6^3} (Fig. 2d and S5†). Furthermore, the effective solvent-accessible void was estimated to be 57.0% (3688.7 Å3) per unit cell calculated using PLATON17 software after removal of guest solvent molecules.
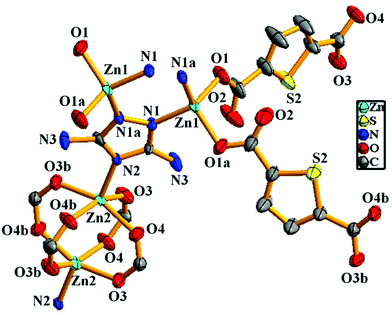 |
| Fig. 1 Asymmetric unit of the Zn-DAT MOF showing the coordination environment at the Zn(II) sites (hydrogen and guest solvent molecules are omitted for clarity, symmetry operation: a = 1 − x, y, −z and b = x, 2 − y, z). | |
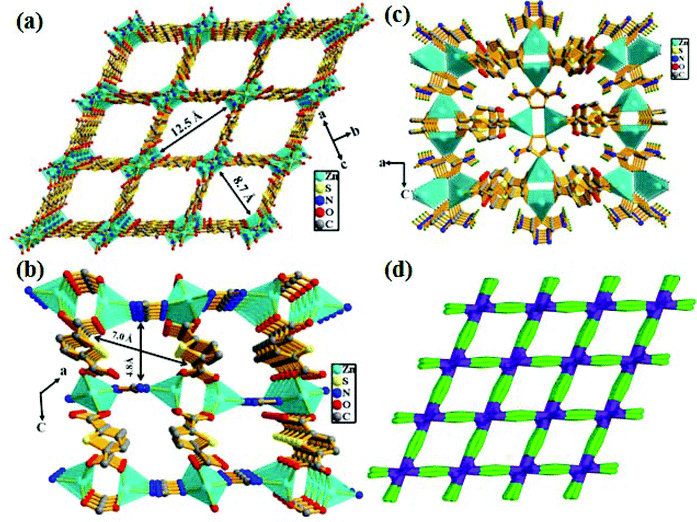 |
| Fig. 2 (a) View of the 3D framework of the Zn-DAT MOF showing the presence of rectangular 1D channels of dimension 12.5 × 8.7 Å2 along the crystallographic c-axis and (b) 1D channels of dimension 7.0 × 4.8 Å2 along the b-axis; (c) the presence of free –NH2 groups of DATRZ in the 1D channels and (d) the TOPOS picture of the MOF. | |
In order to get more insight into the critical role of basic –NH2 groups in the high heat of adsorption value (39.5 kJ mol−1) for CO2, we synthesized the analogous MOF {[Zn2(TDC)(TRZ)2]·(DMA)·(MeOH)}n (Zn-TAZ) containing the 1,2,4-triazole ligand which lacks basic –NH2 groups. As shown in Fig. 3, the crystal structure of Zn-TAZ reported before shows the presence of a 1D channel along the crystallographic a- and c-axes free from –NH2 groups.
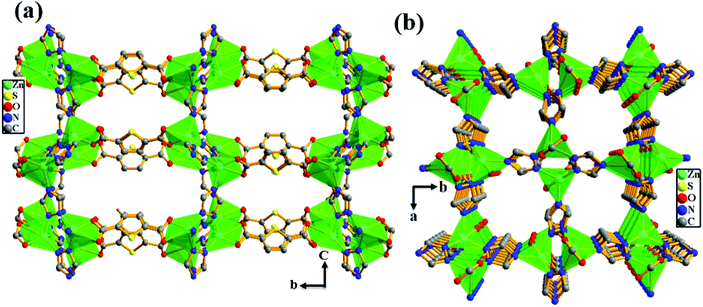 |
| Fig. 3 View of the 3D framework of the Zn-TAZ MOF showing the presence of 1D channels (a) along the crystallographic a-axis and (b) along the crystallographic c-axis. | |
Thermogravimetric analysis of the Zn-DAT MOF
Thermogravimetric analysis of the as-synthesized Zn-DAT MOF shows a weight loss of ∼8% (calc wt% 7.7) around RT–120 °C corresponding to the loss of three guest water molecules. The second weight loss of ∼11.5% (calc wt% 11.38) around 120–200 °C corresponds to the loss of a guest DMF molecule, whereas the third weight loss of ∼47% (calc wt% 47.6) around 220–380 °C is due to the combined loss of TDC and the DATRZ linker. On the other hand, the activated sample of the Zn-DAT MOF does not show any weight loss up to 200 °C, indicating the absence of guest solvent molecules (Fig. 4).
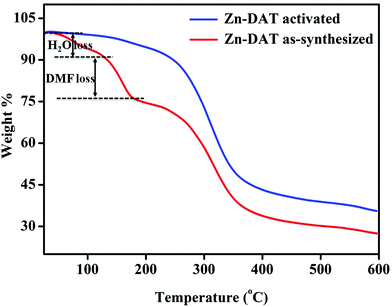 |
| Fig. 4 Thermogravimetric analysis of the Zn-DAT MOF. | |
Gas adsorption studies
As discussed before, the Zn-DAT MOF is microporous with two types of 1D channels of dimensions 12.5 × 8.7 Å2 and 7.0 × 4.8 Å2 along the crystallographic c- and b-axes, respectively. To determine the permanent porosity of the sample, N2 adsorption–desorption measurements were carried out. Before starting the adsorption measurements, the as-synthesized sample was activated at an elevated temperature of 373 K under vacuum (18 mTorr) for 15 hours to obtain a solvent-free MOF. Powder X-ray diffraction pattern (PXRD) analysis of the activated MOF confirmed the retention of the original framework structure of the MOF (Fig. S1†). Furthermore, the N2 adsorption isotherm of the Zn-DAT MOF shows a type-I profile supporting the microporous nature of the MOF and the estimated Brunauer–Emmett–Teller (BET) surface area was found to be 51.6 m2 g−1 (Fig. S6a†). Furthermore, CO2 adsorption isotherms follow a typical type-I behaviour with an uptake of 59.54, 27.07 and 17.64 cc g−1 determined at 195, 273 and 298 K, respectively (Fig. 5a). Moreover, the adsorption isotherms of CO2 were analyzed with the Langmuir–Freundlich equation18 (Fig. S7a and S8a†) to obtain the exact prediction of CO2 gas adsorbed at saturation. The calculated value of heat of adsorption (Qst) for CO2 was found to be 39.5 kJ mol−1 following the Clausius–Clapeyron equation19 (Fig. S9a†). The moderately high value of Qst indicates stronger interaction of CO2 gas with the basic –NH2 groups present in the 1D channel of the Zn-DAT MOF.20 In order to obtain further support on the role of –NH2 groups in the high value of Qst, gas adsorption studies of the analogous MOF Zn-TAZ containing the 1,2,4-triazole (TAZ) ligand instead of 3,5-diaminotriazole were carried out. The estimated Brunauer–Emmett–Teller (BET) surface area of the Zn-TAZ MOF was found to be 46.76 m2 g−1 based on the N2 adsorption isotherm determined at 77 K (Fig. S6b†). Moreover, the CO2 adsorption isotherms determined at 273 and 298 K were analyzed with the Langmuir–Freundlich equation (Fig. S7b and S8b†) to obtain the exact prediction of CO2 gas adsorbed at saturation and the calculated value of heat of adsorption was found to be 31.7 kJ mol−1 (Fig. S9b†) which is about 8 kJ mol−1 lower than that of the Zn-DAT (39.5 kJ mol−1) MOF. This clearly highlights the role of basic –NH2 groups in enhancing the interaction energy of CO2 with the Zn-DAT MOF. Furthermore, CO2 selectivity studies of the Zn-DAT MOF with other gases like N2, Ar, and H2 revealed negligible uptake of 1.85, 2.16 and 1.91 cc g−1 for N2, Ar, and H2 respectively (Fig. 5b). Thus, the observed selective uptake of CO2 by the Zn-DAT MOF can be attributed to the presence of basic –NH2 functionalized 1D channels.21 The estimated gas selectivity constants following Henry's law for CO2/H2, CO2/Ar, and CO2/N2 were found to be 40, 38, and 34 respectively (Fig. S10 and S11†). The relatively high value of the gas selectivity constant for CO2 over other gases indicates the potential scope of the Zn-DAT MOF for selective capture of CO2 from other gases like N2, Ar, and H2. The CO2 adsorption–desorption plots for both MOFs (Zn-DAT and Zn-TAZ) determined at 273 and 298 K along with the heat of adsorption plots are shown in Fig. 6.
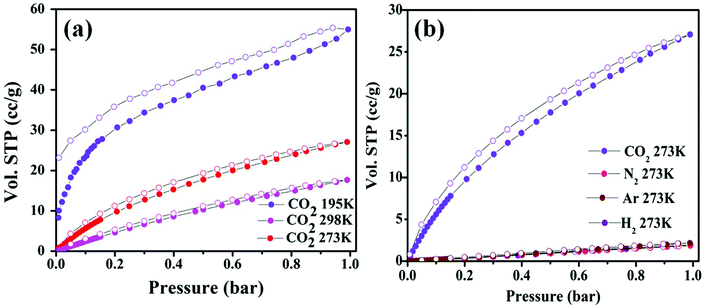 |
| Fig. 5 (a) CO2 adsorption–desorption isotherms of the Zn-DAT MOF determined at 195, 273 and 298 K. (b) Selectivity study for CO2 over other (H2, Ar, and N2) gases carried out at 273 K. | |
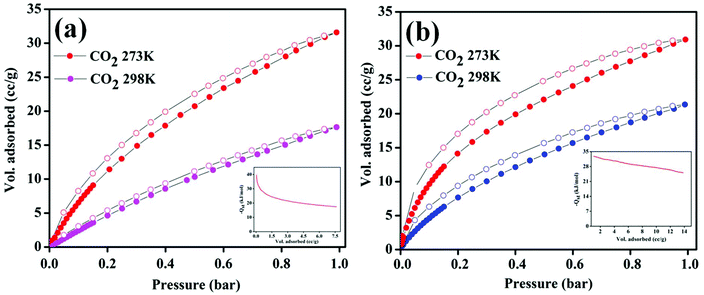 |
| Fig. 6 CO2 adsorption–desorption isotherms of MOFs determined at 273 and 298 K: (a) the Zn-DAT MOF and (b) the Zn-TAZ MOF (insets show the enthalpy of CO2 adsorption). | |
Theoretical study
In order to further establish the interaction of CO2 with the –NH2 groups of the DATRZ linker exposed in the 1D channels of the Zn-DAT MOF, theoretical calculations were performed considering a single 1D pore of the MOF as shown in Fig. 7a. Furthermore, two CO2 molecules were placed in the pore space of the Zn-DAT MOF and the resulting structures were optimized. As shown in Fig. 7b, the CO2 molecules are interacting with the –NH2 groups present in the 1D channels through HN–H⋯OCO and H–N–H⋯OCO⋯H–N–H interactions with the bond distances ranging from 2.785 to 3.781 Å indicated as type-I and type-II respectively. The computed average CO2 binding energy (BE) was found to be 40.9 kJ mol−1 which is very close to the experimentally determined heat of adsorption value of 39.5 kJ mol−1. Moreover, the bond angle of CO2 molecules deviates from 180° to 177.5° and 178.2° in the case of type-I and type-II, respectively, supporting the interaction of CO2 molecules with the –NH2 groups of the DATRZ linker.
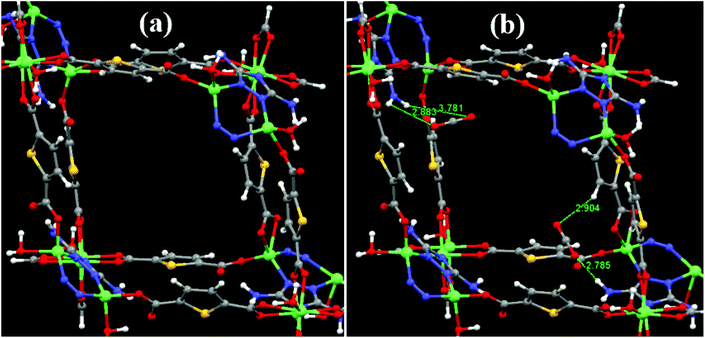 |
| Fig. 7 (a) Optimized structure of the Zn-DAT MOF showing the 1D pore and (b) with two CO2 molecules interacting with the –NH2 groups of the DATRZ linker. | |
Catalytic cycloaddition reaction of CO2
The selective CO2 adsorption property and the presence of both Lewis acidic Zn(II) sites and basic –NH2 groups motivated us to study the catalytic activity of the Zn-DAT MOF for cycloaddition of CO2 to cyclic carbonates. Initially, the catalytic activity was tested under the mild conditions at 0.1 MPa (1 bar) and RT (25 °C) for the cycloaddition of CO2 with 1,2-epoxypropane (PO) as a model substrate in the presence of tetrabutylammonium bromide (TBAB) as a cocatalyst. Remarkably, about 51% of PO was converted into the corresponding cyclic carbonate within 24 h (Table 1, entry 1, and Fig. S12†). A comparison of the catalytic activity with the literature reported MOFs revealed the higher activity of Zn-DAT for catalytic cycloaddition of CO2 to PO under mild conditions at 1 bar and RT (Table 2) highlighting the excellent catalytic activity of the Zn-DAT MOF for chemical fixation of CO2. Furthermore, increasing the pressure of CO2 from 1 bar to 8 bar (0.8 MPa) resulted in almost complete conversion (>99%) of PO into the corresponding cyclic carbonate (Table 1, entry 2, and Fig. S13†). Encouraged by the high catalytic activity of the Zn-DAT MOF, the cycloaddition reaction of CO2 was extended to other epoxides with increasing alkyl chain length and the results are shown in Table 1. Interestingly, the cycloaddition reaction of smaller epoxides like epichlorohydrin (ECH) and 1,2-epoxybutane with CO2 yielded the corresponding cyclic carbonate with >99% conversion (Table 1, entries 3 and 5, Fig. S14 and S15†). This observation can be attributed to the presence of a large 1D channel of the Zn-DAT (12.5 × 8.7 Å2) MOF which is a good fit for the epoxides to diffuse in and react with the catalytic Zn(II) sites (Table S5†). While the relatively lower conversion of longer epoxides, such as 1,2-epoxyhexane, allyl glycidyl ether, butyl glycidyl ether and 1,2-epoxydecane, compared to that of ECH can be attributed to the restricted diffusion of the epoxides in the 1D channels (Table 1, entries 7–10, and Fig. S16–S19†), the considerably lower conversion of 1,2-epoxydecane can be attributed to the bigger size of the epoxide (∼13.321 × 3.39 Å2) compared to the pore size of the Zn-DAT MOF. Furthermore, no formation of side products was observed and the selectivity for the cyclic carbonates was 100%. Furthermore, the catalytic activity of Zn-TAZ was investigated under similar optimized conditions for cycloaddition of ECH and BO; interestingly about 89% and 87% conversions of the epoxides into the corresponding cyclic carbonates was observed respectively in 24 h (Table 1, entries 4 and 6 and Fig. S20 and S21†) which are slightly lower than the conversions observed for the Zn-DAT MOF. The relatively higher catalytic activity of the Zn-DAT MOF over Zn-TAZ can be attributed to the CO2-philic nature of the former MOF owing to the presence of basic –NH2 groups in the 1D channels. Hence, from this discussion, it is clear that Zn-DAT acts as an efficient catalyst for cycloaddition of CO2 with various epoxides under mild conditions at RT. Controlled experiments carried out in the absence of Zn-DAT under similar reaction conditions showed a conversion of only 44% of ECH into the corresponding cyclic carbonate (Fig. S22†). Therefore, from the above mentioned discussion, it is clear that a synergistic cooperation between the MOF catalyst and TBAB is essential for the high yield generation of the cyclic carbonate by efficient cycloaddition of CO2 with epoxides under mild conditions at RT.
Table 1 Catalytic performance of Zn-DAT for the cycloaddition reaction of CO2 with various epoxides carried out at RTa
Entry |
Substrate |
Product |
Temperature/catalyst |
Conversionc (%) |
Reaction conditions: Epoxide (20 mmol), catalyst (0.4 mol%), cocatalyst: TBAB (4 mol%), RT (25 °C), pressure: 0.8 MPa, and time: 24 h.
Time is 12 h and the other conditions are the same.
The catalytic conversions of epoxides were determined by 1H NMR analysis.
|
1. |
|
|
RT/
Zn-DAT
|
51b |
2. |
|
|
RT/
Zn-DAT
|
>99 |
3. |
|
|
RT/
Zn-DAT
|
>99 |
4. |
|
|
RT/
Zn-TAZ
|
89 |
5. |
|
|
RT/
Zn-DAT
|
>99 |
6. |
|
|
RT/
Zn-DAT
|
87 |
7. |
|
|
RT/
Zn-DAT
|
78 |
8. |
|
|
RT/
Zn-DAT
|
66 |
9. |
|
|
RT/
Zn-DAT
|
60 |
10. |
|
|
RT/
Zn-DAT
|
40 |
Table 2 Comparison of the catalytic activity of Zn-DAT for cycloaddition of CO2 to PO with various MOFs reported in the literaturea
Catalyst (mol%) |
Time (h) |
Temperature (°C) |
Pressure (MPa) |
Yield (%) |
Ref. |
Reaction conditions: Propylene oxide (PO), TBAB as a co-catalyst, RT = room temperature.
|
Cu(tactmb) |
48 |
RT |
0.1 |
47.5 |
13a
|
HKUST-1 |
48 |
RT |
0.1 |
49 |
|
MOF-505 |
48 |
RT |
0.1 |
48 |
13b
|
MMPF-9 |
48 |
RT |
0.1 |
87.4 |
22
|
Zn-DAT
|
24
|
RT
|
0.1
|
51
|
This work
|
ZnGlu |
24 |
RT |
1.0 |
65 |
13c
|
Cr-MIL-101 |
24 |
RT |
0.8 |
82 |
13e
|
Fe-MIL-101 |
24 |
RT |
0.8 |
87 |
|
UMCM-1 |
24 |
RT |
1.2 |
85 |
23
|
UMCM-1NH2 |
24 |
RT |
1.2 |
90 |
|
MOF-Zn-1 |
24 |
RT |
1.0 |
98 |
24
|
Zn-DAT
|
24
|
RT
|
0.8
|
>99 |
This work
|
Recyclability test
To test the recyclability, the Zn-DAT MOF was separated from the reaction mixture by centrifugation followed by washing with methanol and activation at 373 K and it was reused for subsequent catalytic cycles for cycloaddition of ECH with CO2 under the optimized conditions at RT and 0.8 MPa. Interestingly, no significant decrease in the catalytic activity was observed even after five cycles (Fig. 8 and S23†). Furthermore, the PXRD pattern of the recycled sample after five cycles matches well with the parent MOF suggesting retention of the original framework structure after catalysis (Fig. S1†).
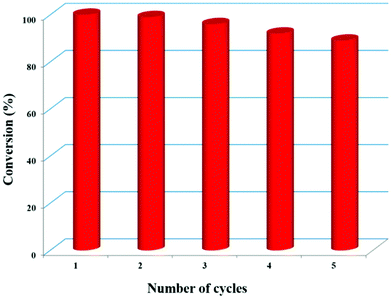 |
| Fig. 8 Recyclability of Zn-DAT for five consecutive cycles. | |
Plausible mechanism
A plausible mechanism for the catalytic cycloaddition reaction of CO2 with epoxides catalyzed by the Zn-DAT MOF is shown in Scheme 2. It involves a binary catalytic system composed of a Lewis acidic Zn(II) site and a TBAB co-catalyst which is essential for ring-opening of the epoxides.25 As proposed in Scheme 2, the first step involves coordination of the epoxide to the Lewis acidic Zn(II) site followed by the polarization of the CO2 molecule through interaction with the basic –NH2 groups of the DATRZ linker which were further supported by the theoretical calculations discussed before.26 Then, ring-opening of the epoxide takes place by a nucleophilic attack of the Br anion of TBAB on the less hindered β-carbon atom of the epoxide. The subsequent intramolecular ring-closure reaction by a nucleophilic attack of the oxyanion with CO2 generates the cyclic carbonate which undergoes reductive elimination to regenerate the catalyst and the catalytic cycle continues.
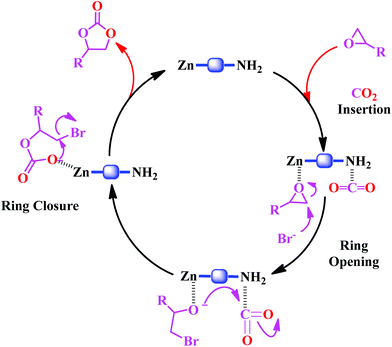 |
| Scheme 2 Proposed mechanism for the catalytic cycloaddition of epoxides to CO2 catalyzed by the Zn-DAT MOF. | |
Conclusions
In summary, this work demonstrates the synthesis of a novel 3D, microporous, bifunctional MOF, {[Zn2(TDC)2(DATRZ)]·(3H2O)·(DMF)}n (Zn-DAT), by a solvothermal route using a mixed ligand strategy. Furthermore, the MOF features a 3D framework structure with two types of 1D channels of dimensions 12.5 × 8.7 Å2 and 7.0 × 4.8 Å2 along the crystallographic c and b-axes, respectively. Due to the presence of basic –NH2 functionalized pores, the Zn-DAT MOF shows selective adsorption of CO2 with a high heat of adsorption (Qst) value of 39.5 kJ mol−1 which is further confirmed by a theoretical BE of 40.9 kJ mol−1. Interestingly, the Qst value observed for Zn-DAT is about 8 kJ mol−1 higher than that of the analogous MOF {[Zn2(TDC)(TRZ)2]·(DMA)·(MeOH)}n (Zn-TAZ) containing the 1,2,4-triazole (TAZ) linker, which supports the critical role of –NH2 groups in CO2 capture. The significantly high value of Qst indicates stronger interaction of Lewis acidic CO2 molecules with the basic –NH2 groups present in the 1D channels of Zn-DAT. Furthermore, the Zn-DAT MOF acts as a bifunctional heterogeneous catalyst for conversion of CO2 into cyclic carbonates under solvent-free mild conditions at RT. This work demonstrates the influence of basic –NH2 groups on the selective capture and conversion of CO2 into cyclic carbonates under mild conditions.
Conflicts of interest
The authors declare no conflict of interest.
Acknowledgements
CMN acknowledges the Science & Engineering Research Board (SERB), Department of Science and Technology, Government of India for financial support (CRG/2018/001176). The authors thank Mr Sandeep Kumar for the theoretical optimization. RD and SSD acknowledge IIT Ropar for JRF and SRF, respectively.
References
-
(a) D. M. D. Alessandro, B. Smit and J. R. Long, Angew. Chem., Int. Ed., 2010, 49, 6058–6082 CrossRef;
(b) M. Z. Jacobson, Energy Environ. Sci., 2009, 2, 148–173 RSC;
(c) S. Tiba and A. Omri, Renewable Sustainable Energy Rev., 2017, 69, 1129–1146 CrossRef.
-
(a) S. Chu, Science, 2009, 325, 1599 CrossRef CAS;
(b) T. Sakakura, J. C. Choi and H. Yasuda, Chem. Rev., 2007, 107, 2365–2387 CrossRef CAS;
(c) H. He, J. A. Perman, G. Zhu and S. Ma, Small, 2016, 12, 6309–6324 CrossRef CAS;
(d) A. C. Kathalikkattil, R. Babu, R. K. Roshan, H. Lee, H. Kim, J. Tharun, E. Suresh and D. W. Park, J. Mater. Chem. A, 2015, 3, 22636–22647 RSC.
-
(a) M. North, R. Pasquale and C. Young, Green Chem., 2010, 12, 1514–1539 RSC;
(b) Y. N. Li, R. Ma, L. N. He and Z. F. Diao, Catal. Sci. Technol., 2014, 4, 1498–1512 RSC;
(c) B. Yu and L. N. He, ChemSusChem, 2015, 8, 52–62 CrossRef CAS.
- T. Sakakura and J. C. Choi Yasuda, Chem. Rev., 2007, 107, 2365–2387 CrossRef CAS.
-
(a) P. Unnikrishnan and D. Srinivas, J. Mol. Catal. A: Chem., 2015, 398, 42–49 CrossRef CAS;
(b) J. Yun, L. Zhang, Q. Qu, H. Liu, X. Zhang, M. Shen and H. Zheng, Electrochim. Acta, 2015, 167, 151–159 CrossRef CAS;
(c) S. Fujita, B. M. Bhanage, H. Kanamaru and M. Arai, J. Mol. Catal. A: Chem., 2005, 230, 43–48 CrossRef CAS;
(d) S. H. Kim and S. H. Hong, ACS Catal., 2014, 4, 3630–3636 CrossRef CAS;
(e) A. Duval and L. Avérous, ACS Sustainable Chem. Eng., 2017, 5, 7334–7343 CrossRef CAS;
(f) J. H. Clements, Ind. Eng. Chem. Res., 2003, 42, 663–674 CrossRef CAS;
(g) H. L. Parker, J. Sherwood, A. J. Hunt and J. H. Clark, ACS Sustainable Chem. Eng., 2014, 2, 1739–1742 CrossRef CAS.
- D. Yun, D. S. Park, K. R. Lee, Y. S. Yun, T. Y. Kim, H. Park, H. Lee and J. Yi, ChemSusChem, 2017, 10, 3671–3678 CrossRef CAS PubMed.
-
(a) Q. Liu, L. Wu, R. Jackstell and M. Beller, Nat. Commun., 2015, 6, 5933 CrossRef;
(b) Q.-W. Song, Z.-H. Zhou and L.-N. He, Green Chem., 2017, 19, 3707–3728 RSC;
(c) S. He, F. Wang, W.-L. Tong, S.-M. Yiu and M. C. W. Chan, Chem. Commun., 2016, 52, 1017–1020 RSC.
-
(a) M. L. Man, K. C. Lam, W. N. Sit, S. M. Ng, Z. Zhou, Z. Lin and P. Lau, Chem. – Eur. J., 2006, 12, 1004–1015 CrossRef CAS;
(b) W. Clegg, R. W. Harrington, M. North and R. Pasquale, Chem. – Eur. J., 2010, 16, 6828–6843 CrossRef CAS;
(c) S. Elmas, M. A. Subhani, M. Harrer, W. Leitner, J. Sundermeyer and T. E. Müller, Catal. Sci. Technol., 2014, 4, 1652–1657 RSC;
(d) M. Liu, K. Gao, L. Liang, J. Sun, L. Sheng and M. Arai, Catal. Sci. Technol., 2016, 6, 6406–6416 RSC;
(e) C. J. Whiteoak, A. Nova, F. Maseras and A. W. Kleij, ChemSusChem, 2012, 5, 2032–2038 CrossRef CAS;
(f) M. E. Wilhelm, M. H. Anthofer, M. Cokoja, I. I. E. Markovits, W. A. Herrmann and F. E. Kühn, ChemSusChem, 2014, 7, 1357–1360 CrossRef CAS;
(g) J. Langanke, L. Greiner and W. Leitner, Green Chem., 2013, 15, 1173–1182 RSC;
(h) Y. Ren and J. J. Shim, ChemCatChem, 2013, 5, 1344–1349 CrossRef CAS;
(i) H. Yasuda, L. N. He, T. Sakakura and C. Hu, J. Catal., 2005, 233, 119–122 CrossRef CAS.
-
(a) Y. Xie, Z. Zhang, T. Jiang, J. He, B. Han, T. Wu and K. Ding, Angew. Chem., Int. Ed., 2007, 46, 7255–7258 CrossRef CAS;
(b) X. L. Meng, Y. Nie, J. Sun, W. G. Cheng, J. Q. Wang, H. Y. He and S. J. Zhang, Green Chem., 2014, 16, 2771–2778 RSC;
(c) Y. Chen, R. Luo, Q. Xu, J. Jiang, X. Zhou and H. Ji, ChemSusChem, 2017, 10, 2534–2541 CrossRef CAS;
(d) J. Li, D. Jia, Z. Guo, Y. Liu, Y. Lyu, Y. Zhou and J. Wang, Green Chem., 2017, 19, 2675–2686 RSC;
(e) J. Roeser, K. Kailasam and A. Thomas, ChemSusChem, 2012, 5, 1793–1799 CrossRef CAS;
(f) R. Ma, L. N. He and Y. B. Zhou, Green Chem., 2016, 18, 226–231 RSC;
(g) M. V. Escárcega-Bobadilla, E. M. Martínez Belmonte, E. Martin, E. C. EscuderoAdan and A. W. Kleij, Chem. – Eur. J., 2013, 19, 2641–2648 CrossRef;
(h) Z. Z. Yang, Y. N. Zhao, L. N. He, J. Gao and Z. S. Yin, Green Chem., 2012, 14, 519–527 RSC;
(i) K. Maity, C. K. Karan and K. Biradha, Chem. – Eur. J., 2018, 24, 10988–10993 CrossRef CAS.
-
(a) Themed Issue: Introduction to Metal-Organic Frameworks, Chem. Rev., 2012, 112, 673–674; ;
(b) Themed Issue: Metal-Organic Frameworks, Chem. Soc. Rev., 2014, 43, 5415–5418; ;
(c) Virtual Issue: Emerging Applications of Metal-Organic Frameworks and Covalent Organic Frameworks, Chem. Mater., 2016, 28, 8079–8081; ;
(d) S. Hasegawa, S. Horike, R. Matsuda, S. Furukawa, K. Mochizuki, Y. Kinoshita and S. Kitagawa, J. Am. Chem. Soc., 2007, 129, 2607–2614 CrossRef CAS;
(e) A. M. Shultz, O. K. Farha, J. T. Hupp and S. T. Nguyen, J. Am. Chem. Soc., 2009, 131, 4204–4205 CrossRef CAS;
(f) S. Horike, M. Dincǎ, K. Tamaki and J. R. Long, J. Am. Chem. Soc., 2008, 130, 5854–5855 CrossRef CAS;
(g) M. Yoon, R. Srirambalaji and K. Kim, Chem. Rev., 2012, 112, 1196–1231 CrossRef CAS;
(h) A. Corma, H. García and F. X. LlabrésiXamena, Chem. Rev., 2010, 110, 4606–4655 CrossRef CAS;
(i) J. Liu, L. Chen, H. Cui, J. Zhang, L. Zhang and C. Y. Su, Chem. Soc. Rev., 2014, 43, 6011–6061 RSC;
(j) T. Zhang and W. Lin, Chem. Soc. Rev., 2014, 43, 5982–5933 RSC;
(k) M. S. Deenadayalan, N. Sharma, P. K. Verma and C. M. Nagaraja, Inorg. Chem., 2016, 55, 5320–5327 CrossRef CAS.
-
(a) Y. L. Wu, J. Qian, G. P. Yang, F. Yang, Y. T. Liang, W. Y. Zhang and Y. Y. Wang, Inorg. Chem., 2017, 56, 908–913 CrossRef CAS;
(b) Z. Hu and D. Zhao, CrystEngComm, 2017, 19, 4066–4081 RSC;
(c) L. An, H. Wang, F. Xu, X. L. Wang, F. Wan and J. Li, Inorg. Chem., 2016, 55, 12923–12929 CrossRef CAS;
(d) J. Zhao, W. W. Dong, Y. P. Wu, Y. N. Wang, C. Wang, D. S. Li and Q. C. Zhang, J. Mater. Chem. A, 2015, 3, 6962–6969 RSC;
(e) K. Sumida, D. L. Rogow, J. A. Mason, T. M. McDonald, E. D. Bloch, Z. R. Herm, T. H. Bae and J. R. Long, Chem. Rev., 2012, 112, 724–781 CrossRef CAS;
(f) C. M. Nagaraja, R. Haldar, T. K. Maji and C. N. R. Rao, Cryst. Growth Des., 2012, 12, 975–981 CrossRef CAS;
(g) R. Vaidhyanathan, S. S. Iremonger, G. K. H. Shimizu, P. G. Boyd, S. Alavi and T. K. Woo, Science, 2010, 330, 650–653 CrossRef CAS;
(h) S. S. Dhankhar and C. M. Nagaraja, New J. Chem., 2019, 43, 2163–2170 RSC;
(i) M. Ding, R. W. Flaig, H.-L. Jiang and O. M. Yaghi, Chem. Soc. Rev., 2019, 48, 2783–2828 RSC;
(j) Q. Yang, C.-C. Yang, C.-H. Lin and H.-L. Jiang, Angew. Chem., Int. Ed., 2019, 58, 3511–3515 CrossRef CAS;
(k) M. Ding and H.-L. Jiang, ACS Catal., 2018, 8, 3194–3201 CrossRef CAS;
(l) H.-Q. Xu, J. Hu, D. Wang, Z. Li, Q. Zhang, Y. Luo, S.-H. Yu and H.-L. Jiang, J. Am. Chem. Soc., 2015, 137, 13440–13443 CrossRef CAS;
(m) B. Ugale and C. M. Nagaraja, RSC Adv., 2016, 6, 28854–28864 RSC.
-
(a) M. S. Liu, L. Liang, X. Li, X. X. Gao and J. M. Sun, Green Chem., 2016, 18, 2851–2863 RSC;
(b) Y.-H. Han, Z.-Y. Zhou, C.-B. Tian and S.-W. Du, Green Chem., 2016, 18, 4086–4091 RSC.
-
(a) W. Y. Gao, Y. Chen, Y. Niu, K. Williams, L. Cash, P. J. Perez, L. Wojtas, J. Cai, Y. S. Chen and S. Ma, Angew. Chem., Int. Ed., 2014, 53, 2615–2619 CrossRef CAS;
(b) J. Song, Z. f. Zhang, S. Hu, T. Wu, T. Jiang and B. Han, Green Chem., 2009, 11, 1031–1036 RSC;
(c) A. C. Kathalikkattil, R. Roshan, J. Tharun, R. Babu, G. S. Jeong, D. W. Kim, S. J. Cho and D. W. Park, Chem. Commun., 2016, 52, 280 RSC;
(d) R. Babu, A. C. Kathalikkattil, R. Roshan, J. Tharun, D. W. Kimb and D. W. Park, Green Chem., 2016, 18, 232–242 RSC;
(e) O. V. Zalomaeva, A. M. Chibiryaev, K. A. Kovalenko, O. A. Kholdeeva, B. S. Balzhinimaev and V. P. Fedin, J. Catal., 2013, 298, 179–185 CrossRef CAS.
-
(a) B. Ugale, S. Kumar, T. J. D. Kumar and C. M. Nagaraja, Inorg. Chem., 2019, 58, 63925–63936 CrossRef;
(b) N. Sharma, S. Dhankhar, S. Kumar, T. J. D. Kumar and C. M. Nagaraja, Chem. – Eur. J., 2018, 24, 16662–16669 CrossRef CAS;
(c) B. Ugale, S. S. Dhankhar and C. M. Nagaraja, Inorg. Chem., 2016, 55, 9757–9766 CrossRef CAS;
(d) N. Sharma, S. S. Dhankhar and C. M. Nagaraja, Microporous Mesoporous Mater., 2019, 15, 372–378 CrossRef;
(e) B. Ugale, S. S. Dhankhar and C. M. Nagaraja, Cryst. Growth Des., 2018, 18, 2432–2440 CrossRef CAS;
(f) N. Sharma, S. S. Dhankhar and C. M. Nagaraja, Sustainable Energy Fuels, 2019 10.1039/c9se00282k.
- F.-H. Liu, C. Qin, Y. Ding, H. Wu, K.-Z. Shaoa and Z.-M. Su, Dalton Trans., 2015, 44, 1754–1760 RSC.
- V. A. Blatov, A. P. Shevchenko and D. M. Proserpio, Cryst. Growth Des., 2014, 14, 3576–3586 CrossRef CAS.
- A. L. Spek, Single-crystal structure validation with the program PLATON, J. Appl. Crystallogr., 2003, 36, 7–13 CrossRef CAS.
-
(a)
R. T. Yang, Gas Separation by Adsorption Processes, Butterworth, Boston, 1997 CrossRef;
(b) B. Ugale, S. S. Dhankhar and C. M. Nagaraja, Cryst. Growth Des., 2017, 17, 3295–3305 CrossRef CAS;
(c) S. S. Dhankhar and C. M. Nagaraja, RSC Adv., 2016, 6, 86468–86476 RSC;
(d) N. Sikdar, A. Hazra and T. K. Maji, Inorg. Chem., 2014, 53, 5993–6002 CrossRef CAS PubMed.
- H. Pan, J. A. Ritter and P. B. Balbuena, Langmuir, 1998, 14, 6323–6327 CrossRef CAS.
-
(a) S. Couck, J. F. M. Denayer, G. V. Baron, T. Rémy, J. Gascon and F. Kapteijn, J. Am. Chem. Soc., 2009, 131, 6326–6327 CrossRef CAS;
(b) D. Farrusseng, C. Daniel, C. Gaudillère, U. Ravon, Y. Schuurman, C. Mirodatos, D. Dubbeldam, H. Frost and R. Q. Snurr, Langmuir, 2009, 25, 7383–7388 CrossRef CAS.
-
(a) H.-Y. Yang, Y.-Z. Li, W.-J. Shi, L. Hou, Y.-Y. Wang and Z. Zhu, Dalton Trans., 2017, 46, 11722–11727 RSC;
(b) H.-H. Wang, L.-N. Jia, L. Hou, W.-J. Shi, Z.-H. Zhu and Y.-Y. Wang, Inorg. Chem., 2015, 54, 1841–1846 CrossRef CAS;
(c) S. S. Dhankhar, N. Sharma, S. Kumar, T. J. D. Kumar and C. M. Nagaraja, Chem. – Eur. J., 2017, 23, 16204–16212 CrossRef;
(d) B. Ugale, S. S. Dhankhar and C. M. Nagaraja, Inorg. Chem. Front., 2017, 4, 348–359 RSC.
- W. Y. Gao, L. Wojtas and S. Ma, Chem. Commun., 2014, 50, 5316–5318 RSC.
- R. Babu, A. C. Kathalikkattil, R. Roshan, J. Tharun, D. W. Kimb and D. W. Park, Green Chem., 2016, 18, 232–242 RSC.
- J. Lan, M. Liu, X. Lu, X. Zhang and J. Sun, ACS Sustainable Chem. Eng., 2018, 6, 8727–8735 CrossRef CAS.
-
(a) N. Wei, R. X. Zuo, Y. Y. Zhang, Z. B. Han and X. J. Gu, Chem. Commun., 2017, 53, 3224–3227 RSC;
(b) H. Xu, B. Zhai, C. S. Cao and B. Zhao, Inorg. Chem., 2016, 55, 9671–9676 CrossRef CAS;
(c) J. Liang, Y. Q. Xie, X. S. Wang, Q. Wang, T. T. Liu, Y. B. Huang and R. Cao, Chem. Commun., 2018, 54, 342–345 RSC.
-
(a) Y. Lin, C. Kong and L. Chen, RSC Adv., 2016, 6, 32598–32614 RSC;
(b) K. Sumida, D. L. Rogow, J. A. Mason, T. M. McDonald, E. D. Bloch, Z. R. Herm, T.-H. Bae and J. R. Long, Chem. Rev., 2012, 112, 724–778 CrossRef CAS.
Footnote |
† Electronic supplementary information (ESI) available: Crystallographic data of Zn-DAT (CIF), table of bond lengths and angles, PXRD plots for the compounds, structural data, gas adsorption–desorption isotherms, 1H NMR plots of the catalytic reactions, Cartesian coordinates of the optimized structures. CCDC 1918936. For ESI and crystallographic data in CIF or other electronic format see DOI: 10.1039/c9qi01058k |
|
This journal is © the Partner Organisations 2020 |
Click here to see how this site uses Cookies. View our privacy policy here.