DOI:
10.1039/C9QI01264H
(Research Article)
Inorg. Chem. Front., 2020,
7, 157-168
Theoretical insights into the effect of pristine, doped and hole graphene on the overall performance of dye-sensitized solar cells†
Received
30th September 2019
, Accepted 19th October 2019
First published on 21st October 2019
Abstract
Graphene, a promising two-dimensional carbon material, has been extensively employed in dye-sensitized solar cells (DSSCs) with encouraging results. However, how graphene improves the power conversion efficiency is still unclear. In this research, a series of dyes with pristine, doped and hole graphene based on the dye WS5 were designed and systematically investigated by density functional theory (DFT) and time-dependent DFT (TD-DFT), aimed at revealing the effect of these functionalized graphenes on photophysical properties. The results based on the absorption properties reveal that introducing functionalized graphene not only enhances absorption intensity, but also expands the absorption spectrum, which will lead to a better photocurrent response. According to an analysis of the intermolecular interaction between dye and I2, all the designed dyes possess a slow electron recombination rate, except WS5-HoS, and then possibly an increasing VOC. Additionally, Jph increases steadily as the electronegativity of the doped atoms (C, Si and Ge) in graphene increases. The dyes with the insertion of short functionalized graphene exhibit a superior performance in Jph to that of long functionalized graphene. Meanwhile, using graphene to decorate the dye WS5 could adjust energy levels to narrow the energy gap. Dyes with short functionalized graphene exhibit the smallest HOMO–LUMO gaps, followed by long functionalized graphene and the dye WS5. With the enhancement in the electronegativity of doped atoms (C, Si and Ge) in graphene, the energy gap decreases steadily. Inserting functionalized graphene facilitates electron transfer properties due to lower aromaticities, especially for dyes with short functionalized graphene. Taken all together, the introduction of short functionalized graphene in a dye is a valid approach to increase overall efficiency, especially for graphene doped with atoms of larger electronegativity, so the photophysical properties of DSSCs could be boosted in the future.
1. Introduction
The extensive use of fossil fuels has led to serious environmental pollution over the past few decades. With the increasing awareness of environmental protection, sunlight as a green renewable energy source is widely used in various fields, in particular in photovoltaic conversion technologies. In all types of energy devices, the dye-sensitized solar cell (DSSC) is a promising photovoltaic device due to its easy production process, low cost and easily tunable optical properties. Moreover, the conversion efficiency of DSSCs has been reported to be over 14%.1 A typical DSSC usually includes a semiconductor material (mostly TiO2), electrolyte, sensitizer, and counter electrode. The sensitizer is regarded as a crucial part among all the components, which shoulders the responsibility for light harvesting, electron transfer and injection. In general, sensitizers can be classified into metal–organic dyes and metal-free organic dyes, and the latter have been extensively investigated because of their flexible molecular design, environmental friendliness and lower cost.
Organic dyes with a D-π–A configuration have been widely studied due to their high molar extinction coefficients, which exhibit a push–pull character. This structure can promote intramolecular charge transfer, and then inject electrons into the semiconductor. However, dyes adopting a D-π–A model usually exhibit narrow absorption spectra in the UV-visible region, leading to poor harvesting of solar energy and great loss of photon conversion efficiency. Therefore, it is necessary to find a reasonable strategy to overcome the above problems. Graphene, a promising two-dimensional carbon material, has been widely researched due to its superior light absorption properties, excellent flexibility, electrochemical stability, strong photoluminescence and high electron mobility. Graphene has been extensively employed in DSSCs with encouraging results. Mihalache et al.2 found that graphene quantum dots are beneficial for improving light harvesting efficiency and charge transfer properties. Zhang et al.3 studied the impact of graphene on photoelectric properties using DFT/TD-DFT. The results indicate that dyes with graphene exhibit better performance than other designed dyes due to their enhanced light harvesting capability, and increased absorption bands and coefficients. Graphene and molecules can be linked through covalent functionalization,4 which will provide a new way to enhance the photoelectric properties of DSSCs. Hence, the introduction of graphene is a promising strategy to improve efficiency. Additionally, the photoelectric properties of graphene can be effectively tuned by introducing various heteroatoms, such as N, S, O, Si and B.5–9 This is due to the difference in electronegativity between the atomic dopant and the carbon atom. However, the relationship between atomic electronegativity and photovoltaic properties has not been completely clarified.
Graphene can be classified into pristine and defective kinds. Pristine graphene consists of cir-coronene, which exhibits a planar state.10 For defective graphene, a single pentagon–heptagon membered ring is embedded in the sheet, which exhibits a warped shape.11 In recent years, various graphene models (such as C42, C54, C66 and C80) have been studied through density functional calculations.10,11 With the aim of saving computational time, we take cir-coronene C42 with few C atoms as the graphene model to make a reasonable reduction in the computational load, since it is similar to a rectangle, as shown in Fig. 1. This method is widely used in theoretical research.12–14 Previous works have reported that the length of the conjugate bridge affects the optoelectronic performance.15,16 Hence, A–B (Short) and C–D (Long) directions were selected to study the effect of graphene distance on the photoelectric properties of DSSCs. Based on the outstanding WS5 dye,17 a series of graphene-dyes were designed, in which WS5-CS (WS5-CL) represents the introduction of pristine graphene into the dye, WS5-SiS (WS5-SiL) represents the Si-doped graphene, WS5-GeS (WS5-GeL) indicates Ge-doped graphene, WS5-HoS (WS5-HoL) represents hole graphene (see Fig. 1). The total energies of the dyes before and after adsorption on the (TiO2)16 cluster are listed in Table S1.† Hole graphene is not rare, and can be obtained by synthesis, transfer, and oxidation/reduction processes or even introduced intentionally.18 Doping Si and Ge into graphene can be achieved using solution exfoliation techniques or the low-energy ion implantation technique.19–21 From the experimental results, a doped atom directly replaces a carbon atom in graphene, bonding to three C atom neighbours, which is also widely predicted by first-principles calculations.22–24 The purpose of our work is to theoretically investigate which type of graphene is beneficial for improving the photoelectric conversion efficiency of DSSCs and to reveal structure–property relationships. Herein, some key photovoltaic parameters, including charge transfer (CT) characters, energy gap, light harvesting efficiency, absorption spectra, aromaticity and maximal photon generated current (Jph) of the dyes were predicted using DFT and TD-DFT methods. According to these quantum chemical calculations, we hope to provide theoretical guidelines for future experimental research.
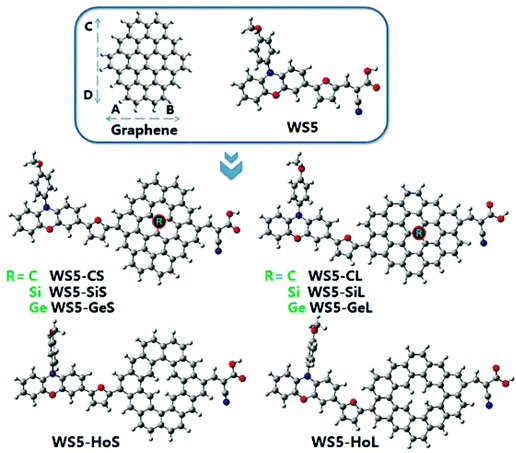 |
| Fig. 1 Structure of the investigated dyes and graphene. | |
2. Computational methods
The geometries of the ground-states of all isolated dyes in dichloromethane solution were fully optimized using the B3LYP function with the 6-31G(d,p) basis set. This level of theory has been widely used in related theoretical research due to its stable and comprehensive performance.25–27 Based on the optimized ground state geometries, the bond lengths, dihedral angles, frontier molecular orbitals (FOMs) and energy levels were obtained. Moreover, the anionic and cationic states of the dyes were optimized at the same level to evaluate the ionization potentials (IP), electron affinities (EA) and chemical reactivity parameters. The time-dependent density functional theory (TD-DFT) method was performed to calculate the optoelectronic properties of the investigated dyes with the optimized molecular structures. To select a proper functional for the TD-DFT calculation, five exchange correlation (XC) functionals, i.e., WB97XD,28 CAM-B3LYP,29 BMK,30 MPW1PW91,31 and B3LYP,32 were selected to simulate the absorption spectra of dye WS5 (Fig. 2). The maximum absorption wavelength and absorption shape simulated by the BMK functional together with the 6-31G(d,p) basis set are closest to the experimental results. Therefore, the BMK/6-31G(d,p) method was employed to predict the optical properties, including transition energies (Eg), oscillator strengths (f), and maximum absorption wavelengths (λmax), in this study. The conductor-like polarizable continuum model (CPCM)33 was used to consider the effect of solvent environment (dichloromethane). Additionally, the intramolecular charge transfer parameters, heat map and aromaticities were calculated with the Multiwfn program.34 To gain a better understanding of the interface properties between dyes and the TiO2 surface, the (TiO2)16(H2O)2 cluster model was prepared as the adsorption surface, which was obtained by cutting from the exposed anatase (101) crystal surface. (TiO2)16 as the smallest TiO2 anatase (101) cluster can give reasonable results without obvious differences compared with large models.35 This model has been widely used to study dye/TiO2 interface systems.36–39 The geometrical optimizations of dyes binding to the TiO2 cluster were investigated using the same level with the 6-31G(d) basis set for non-metal atoms and the LANL2DZ40 basis set for metal atoms. All calculations were performed with the Gaussian 09 program package.41
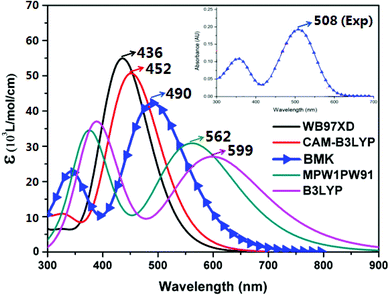 |
| Fig. 2 Absorption spectra of the dye WS5 in dichloromethane solution under different exchange–correlation functionals with the 6-31G (d) basis set. | |
3. Results and discussion
3.1 Electronic structures
The reference dye WS5 is composed of phenoxazine (POZ) as a donor, furan as a π-spacer and cyanoacrylic acid (CAA) as an acceptor. For the sake of improving light harvesting ability, a series of dyes were designed by introducing functionalized graphene between the π-spacer and acceptor, based on the dye WS5. The distortion of the π-spacer affects the charge transfer process. The dihedral angles between furan and functionalized graphene are 26.59 (40.97), 25.25 (38.48), 24.66 (38.57) and −24.04° (43.43°) for WS5-CS (WS5-CL), WS5-SiS (WS5-SiL), WS5-GeS (WS5-GeL) and WS5-HoS (WS5-HoL), respectively. It is found that inserting shorter functionalized graphene effectively improves coplanarity, which facilitates electron delocalization and intramolecular charge transfer (ICT) behavior.42 As shown in Fig. 3, the lengths of bond 1 to bond 3 are 1.411, 1.428, 1.430 Å for the C42 model; 1.745, 1.759, 1.761 Å for the C41Si model; and 1.828, 1.840, 1.842 Å for the C41Ge model. It is clear that the bond length is increasing steadily with an enhancement in the electronegativity of the doped atoms (C, Si and Ge) in graphene. It can be seen from the side view that Ge and Si are located slightly above the graphene plane due to the increase in bond length.
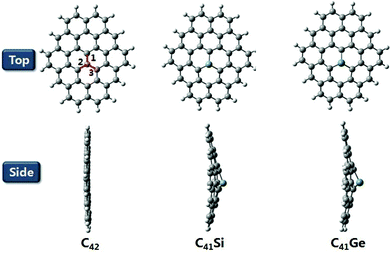 |
| Fig. 3 Optimized structures of C42, C41Si and C41Ge in top and side view. | |
The HOMO and LUMO energy levels of the dyes are very important to ensure dye regeneration and electron injection. The HOMO and LUMO energy levels and energy gaps for the studied dyes are shown in Fig. 4. Clearly, the HOMO levels of all dyes are under the redox potential of I−/I3− so that oxidized dyes can be efficiently regenerated.43 In addition, the LUMO levels are above the conduction band edge of TiO2, which ensures efficient electron injection from the excited state dye into the TiO2 photo-anode.44 Compared with the dye WS5, introducing functionalized graphene between the π-spacer and acceptor leads to the HOMO energy being up-shifted and the LUMO energy being down-shifted. It is interesting that the change in atomic electronegativity (C, Si and Ge) in the graphene and the distance of functionalized graphene have little effect on HOMO energy levels; however, the influence on LUMO energy levels is obvious. An enhancement in the electronegativity of the doped atoms (C, Si and Ge) in graphene and a decrease in the functionalized graphene distance lead to lower LUMO energy levels. The smaller energy gap promotes dye excitation and thus harvests more sunlight. The HOMO–LUMO gaps for the dyes obey the order WS5 > WS5-CL > WS5-HoL > WS5-SiL > WS5-HoS > WS5-CS > WS5-SiS > WS5-GeL > WS5-GeS. The following features are revealed: (i): the introduction of functionalized graphene will reduce the energy gap, which is beneficial to the excitation process; (ii): the dyes with an A–B (Short) direction exhibit a lower energy gap than that of the C–D (Long) direction; (iii): the energy gap decreases steadily with an enhancement in the electronegativity of the doped atoms (C, Si and Ge) in graphene.
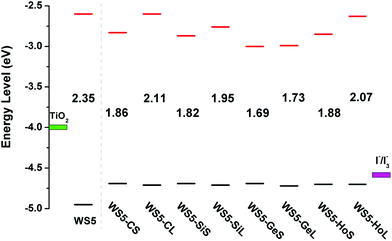 |
| Fig. 4 The energy level of HOMO and LUMO for all studied dyes. | |
3.2 IP and EA and chemical reactivity parameters
Ionization potential (IP) and electron affinity (EA) can be used to describe the energy barrier for hole and electron injection. IP denotes the energy change for injecting electrons or adding holes, and EA implies the energy change for injecting holes or absorbing electrons, which can be calculated via the following formulae: | IP = Ecation − Eneutral | (1) |
| EA = Eneutral − Eanion | (2) |
where Eneutral is the energy of a neutral molecule in the ground state, and Ecation (Eanion) is the energy of a cation (anion) calculated on the basis of the optimized neutral molecule. A smaller IP promotes hole injection, while a larger EA will promote electron injection. As can be seen in Table 1, the results show that all designed dyes have a small IP and larger EA values than that of WS5. Therefore, the insertion of functionalized graphene is beneficial to increasing the electron and hole injection capability.
Table 1 Ionization potential, electron affinity and chemical reactivity (in eV) of dyes
|
IP |
EA |
h
|
ω
+
|
ω
|
WS5 |
4.888 |
2.710 |
1.089 |
4.863 |
6.626 |
WS5-CS |
4.622 |
3.031 |
0.796 |
7.389 |
9.203 |
WS5-CL |
4.635 |
2.837 |
0.899 |
6.007 |
7.763 |
WS5-SiS |
4.615 |
3.052 |
0.782 |
7.583 |
9.402 |
WS5-SiL |
4.638 |
3.049 |
0.795 |
7.474 |
9.297 |
WS5-GeS |
4.616 |
3.197 |
0.710 |
8.890 |
10.755 |
WS5-GeL |
4.629 |
3.215 |
0.707 |
9.006 |
10.878 |
WS5-HoS |
4.624 |
3.075 |
0.775 |
7.739 |
9.567 |
WS5-HoL |
4.625 |
2.858 |
0.884 |
6.162 |
7.922 |
Chemical hardness (h) represents resistance to intramolecular charge transfer. A dye with a lower h exhibits better charge transfer, thereby emerging with a greater short circuit current density (JSC). After inserting functionalized graphene, the calculated values of h for all designed dyes are in the range of 0.707–0.899 eV, which are lower than that of WS5 (1.089 eV). Hence, designed dyes will present intramolecular charge transfer and JSC due to lower h. In addition, the h values decrease from WS5-CS (WS5-CL) to WS5-GeS (WS5-GeL) with an enhancement in electronegativity. This indicates that highly electronegative atom-doped graphene is beneficial for improving JSC. ω+ and ω represent electron-accepting power and electrophilicity index, respectively. Larger values of ω+ and ω are preferable. From Table 1, we can see that the ω+ and ω of dyes show the same trend, which are in the order WS5-GeL > WS5-GeS > WS5-HoS > WS5-SiS > WS5-SiL > WS5-CS > WS5-HoL > WS5-CL > WS5. Therefore, all the designed dyes exhibit better electron-accepting ability and stabilization energy due to larger ω+ and ω values. With an enhancement in the electronegativity of the doped atoms (C, Si and Ge) in graphene, ω+ and ω increase gradually from WS5-CS (WS5-CL) to WS5-GeS (WS5-GeL). In conclusion, all the designed dyes will exhibit prominent photoelectrical properties due to a lower h and larger ω+ and ω.
3.3 Intramolecular charge transfer (ICT) analysis
Effective charge separation will lead to a better charge transfer (CT) property, which is beneficial for improving conversion efficiency. To visualize the CT characteristics more insightfully, the centroids of the charge for WS5 and the designed dyes are depicted in Fig. 5 and Fig. S1,† in which the blue and green zones correspond to the positive and negative charge distributions. Apparently, all the dyes exhibit a considerable charge separation, except for WS5-SiS, WS5-GeS and WS5-HoL. As can be seen from the heat map, the holes of WS5 mainly localize on the POZ group, while the electrons mainly locate on the furan and CAA groups. The CT originates from POZ to furan and CAA due to the greater contribution of furan for the electrons than for the holes. For WS5-CS, WS5-CL, WS5-SiL, WS5-GeL and WS5-HoS, the CT originates from POZ and furan to CAA. For WS5-SiS, WS5-GeS and WS5-HoL, there is a larger overlap on the functionalized graphene. With the purpose of quantifying the extent of the ICT, the CT indexes are calculated and presented in Table 2. These important parameters include the CT distance (DCT), which is the difference between the density distribution of the two bary centers upon photo-excitation. The Sr index can be obtained from the integration of electron and hole density over all space, which describes the extent of overlap of the hole–electron distribution. H represents half of the sum of the centroid axis along the electron transfer direction. The t index is the difference between DCT and H (t = DCT − H), which can be used to estimate the through-space character with CT excitation. WS5-CL has the largest DCT (11.46 Å), followed by WS5-CS (9.04 Å), WS5-HoS (7.28 Å), WS5-GeL (7.06 Å) and WS5-SiL (6.50 Å), which are larger than that for WS5 (4.55 Å), implying that those dyes have better ICT properties. Estimated values of H imply that the introduction of functionalized graphene can increase the distance of the centroid axis along the electron transfer direction. The negative charge density of all designed dyes is centered at functionalized graphene (except for WS5-CS/L), which leads to a larger extent of overlap of the hole–electron distribution, which can be confirmed by the Sr values.
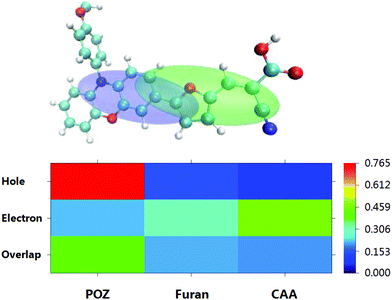 |
| Fig. 5 The centroids of charge (up) and heat map (down) of WS5. | |
Table 2 Calculated charge transfer parameters of investigated dyes
|
D
CT (Å) |
Sr |
H (Å) |
t (Å) |
WS5 |
4.55 |
0.62 |
3.51 |
1.04 |
WS5-CS |
9.04 |
0.61 |
6.16 |
2.88 |
WS5-CL |
11.46 |
0.51 |
5.57 |
5.89 |
WS5-SiS |
2.31 |
0.81 |
4.41 |
−2.10 |
WS5-SiL |
6.50 |
0.73 |
6.19 |
0.31 |
WS5-GeS |
0.75 |
0.84 |
4.05 |
−3.30 |
WS5-GeL |
7.06 |
0.73 |
6.68 |
0.38 |
WS5-HoS |
7.28 |
0.68 |
5.97 |
1.31 |
WS5-HoL |
2.20 |
0.84 |
5.48 |
−3.28 |
3.4 Aromaticity
In order to gain a better understanding of the influence of functionalized graphene on the ICT properties, the aromaticities of furan and functionalized graphene in the π-spacer are calculated. A dye with a lower aromaticity will exhibit a better ICT process. The nucleus-independent chemical shift (NICS) is a popular index to measure aromaticity. The NICS(1) value can be calculated at 1 Å above the geometric center of the ring to describe the magnitude of aromaticity.45 A larger NICS(1) value corresponds to a smaller aromaticity, which is shown in Fig. 6. In order to achieve greater electronic movement from the donor (POZ) to the anchor group (CAA), the aromaticity of these aromatic rings should be as small as possible. The NICS(1) values of the furan moiety are in the order WS5-GeS (−20.58) > WS5-HoS (−20.60) > WS5-CS (−20.62) > WS5-SiS (−20.80) > WS5-CL (−21.80) > WS5-SiL (−21.21) > WS5-GeL (−21.33) > WS5-HoL (−22.38) > WS5 (−26.87). It is clear that all the designed dyes exhibit more positive NICS(1) values compared to reference dye WS5, implying that inserting functionalized graphene facilitates electron delocalization and ICT. Additionally, the furan near short functionalized graphene will exhibit a predominant electron transfer character compared to that of furan close to long functionalized graphene. The effect of different atom-doped graphenes on ICT was also studied. The results show that NICS(1) values increase steadily as the electronegativity of the doped atoms (C, Si and Ge) in the graphene increases, indicating that dye with Ge-doped graphene will exhibit better photoelectric performance.
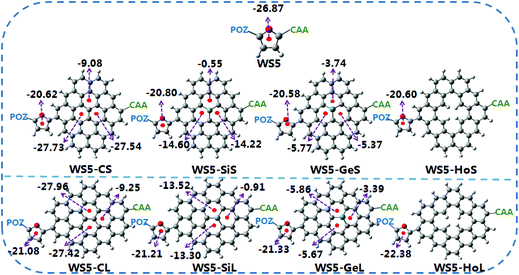 |
| Fig. 6 Schematic diagram of electron transfer routes in molecular π-spacer in which the NICS(1) values of the individual heterocyclic rings are indicated. | |
3.5 Photophysical properties
The light absorption properties of a dye is a vital factor that influences the efficiency of a DSSC, which can be evaluated from UV-vis absorption spectra. A dye with a strong absorption intensity and a wider absorption region usually shows better performance. With the purpose of investigating the effect of functionalized graphene on the light absorption property, the absorption spectra of the studied dyes in dichloromethane which were simulated using the TDDFT/BMK/6-31G(d,p) level are depicted in Fig. 7. Moreover, the corresponding electronic transition properties upon photo-excitation are summarized in Table 3. It is obvious from Fig. 7 that introducing functionalized graphene not only enhances absorption intensity, but also expands the absorption spectrum, which will lead to a better photocurrent response. From Table 3, the maximum absorption peak (λmax) of prototype dye WS5 is 490 nm, which is assigned to the HOMO to LUMO transition. All designed dyes exhibit a blue shift of 60–111 nm with respect to WS5, implying that the introduction of functionalized graphene would lead to a hypsochromic shift of the absorption spectrum. In addition, the λmax of WS5-CS, WS5-SiS, WS5-GeS and WS5-HoS show a red shift compared with WS5-CL, WS5-SiL, WS5-GeL and WS5-HoL, indicating that the introduction of shorter functionalized graphene (A–B direction) is beneficial to the bathochromic shift of the absorption spectrum.
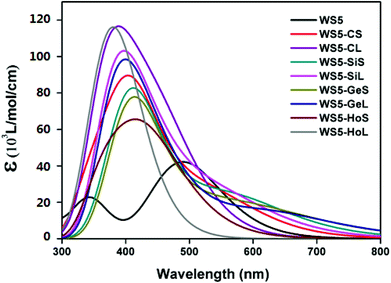 |
| Fig. 7 Simulated absorption spectra of all dyes. | |
Table 3 The absorption characteristics of the dyes calculated by BMK/6-31G(d) level in dichloromethane
Dye |
E
g (eV) |
λ
max (nm) |
f
|
Main configuration |
WS5 |
2.53 |
490 |
1.0420 |
H → L/0.68722 |
WS5-CS |
3.00 |
414 |
0.9565 |
H → L + 1/0.38956 |
WS5-CL |
3.23 |
384 |
0.9801 |
H-2 → L + 1/0.55375 |
WS5-SiS |
3.02 |
410 |
1.1437 |
H-2 → L/0.36583 |
WS5-SiL |
3.18 |
389 |
1.0006 |
H → L + 2/0.43858 |
WS5-GeS |
2.97 |
417 |
0.7909 |
H-4 → L/0.36651 |
WS5-GeL |
3.20 |
388 |
1.4290 |
H → L + 2/0.32353 |
WS5-HoS |
2.88 |
430 |
0.9270 |
H → L + 1/0.50605 |
WS5-HoL |
3.27 |
379 |
1.1218 |
H-1 → L + 2/0.37904 |
To investigate the effect of functionalized graphene on the charge-separation process, selected frontier molecular orbitals (FMOs) of the isolated investigated dyes are plotted in Fig. 8. The transition character of prototype dye WS5 arises from HOMO to LUMO. To achieve an effective charge separation, the HOMO should populate on the donor segment and LUMO should localize on the acceptor moiety. As depicted in Fig. 8, the HOMO orbital of WS5 is centered on the whole framework, while the LUMO is populated over the right part of the donor, furan and acceptor. This distributive character is unfavorable for charge transfer and photoinduced electron injection. For designed dyes, introducing shorter functionalized graphene (A–B direction) will lead to better charge separated states except for WS5-GeS. As we know, the –COOH group is used to graft onto TiO2 and transport electrons. More electrons persisting in the –COOH group for the lowest unoccupied molecular orbitals will lead to a faster electron transfer rate, which is beneficial for improving the performance of DSSCs. The distributive shape of LUMO and LUMO+1 for the dyes with shorter functionalized graphene (A–B direction) is as expected, and the electrons are distributed on the –COOH group. In contrast, the electron density of LUMO+1 and LUMO+2 for the dyes with longer functionalized graphene (C–D direction) are only located at the functionalized graphene group, which will lead to a negative influence on the conversion efficiency of DSSCs. From the FMO distributions of WS5-HoS and WS5-HoL, we can deduce that introducing hole graphene is not favorable to the charge transfer process.
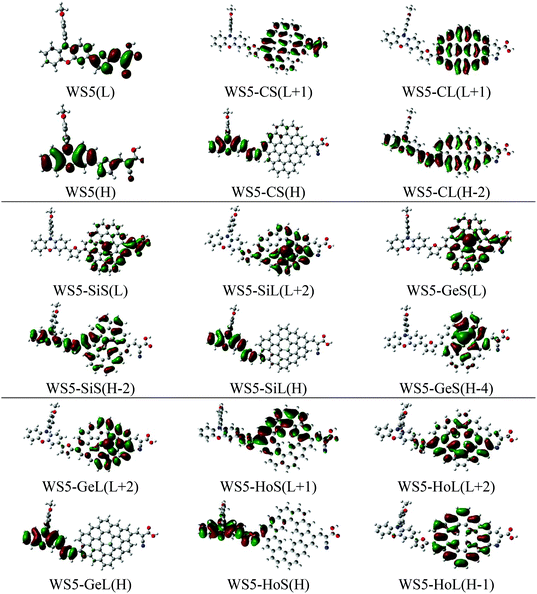 |
| Fig. 8 The selected frontier molecular orbitals (FMOs) of the isolated investigated dyes. | |
In order to achieve a high photoelectric conversion efficiency, a dye should absorb as much energy from sunlight as possible. ηLHE(λ) represents the light harvesting efficiency at a given wavelength λ. A higher ηLHE(λ) is beneficial for improving the performance of a solar cell, which can be calculated as:46,47
where
Γ is the surface loading of dye (mol cm
−2) adsorbed on the semiconductor;
σ(
λ) is the molecular absorption cross-section (cm
2 mol
−1), which can be obtained from the molar absorption coefficient
ε(
λ) by multiplication by 1000.
46,48 The values of
ηLHE(
λstrong) and
LHE(
λ) are calculated and presented in
Fig. 9(a). The
ηLHE(
λstrong) is calculated at the strongest absorption peak. As can be seen from
Fig. 9(a), introducing functionalized graphene into dye WS5 obviously enhances
ηLHE(
λstrong). In addition, we also calculate the average light harvesting efficiency
LHE(
λ). It is found that all the designed dyes exhibit a higher
LHE(
λ) except WS5-CL, WS5-HoS and WS5-HoL.
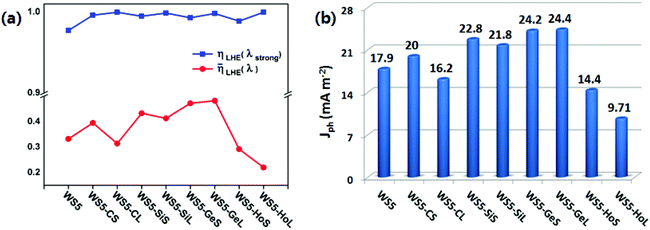 |
| Fig. 9 (a) The calculated ηLHE(λstrong) and LHE(λ) for all dyes; (b) the generated Jph (mA cm−2) for all dyes. | |
For DSSC as a photoelectric conversion device, the more photons the dyes can get from sunlight, the more electrons can be excited and then injected into TiO2, which will increase the short-circuit current density (JSC). JSC is an important parameter affecting the overall efficiency of the DSSCs, which is related to the integral photon flux density Φ(λ). Hence, the exact part of Φ(λ) generated by the dyes per second per unit area at the given wavelength (λ) can be obtained as follows.49 For more details, please refer to these publications.12,47,50
| 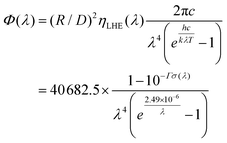 | (4) |
The maximal photon generated current (Jph) can be described by the following equation:
| 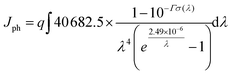 | (5) |
According to eqn (5), the calculated Jph of all the dyes are shown in Fig. 9(b). Among the designed dyes, dyes with Ge-doped graphene (WS5-GeS/WS5-GeL) exhibit the greatest Jph, followed by Si-doped graphene (WS5-SiS/WS5-SiL), pure graphene (WS5-CS/WS5-CL) and hole graphene (WS5-HoS/WS5-HoL). The following features can be observed: (i): Jph increases steadily as the electronegativity of the doped atoms (C, Si and Ge) in graphene increases; (ii) Inserting short functionalized graphene will increase the value of Jph except for WS5-GeS/WS5-GeL; (iii) inserting hole graphene in WS5 has a negative influence on the performance of DSSC due to the lower Jph. The flow of electric charge across a surface per second per unit area over the whole spectrum equals the short circuit density (Jmaxsc).38 Therefore, we can infer that inserting Ge-doped, Si-doped and pure graphene in WS5 is beneficial to improving the performance of DSSCs.
3.6 Electro-optical properties of dye@TiO2 systems
To better understand the mechanism of electron injection from the excited dye into the semiconductor, the photoelectrical properties of dyes adsorbed on the (TiO2)16 cluster were systematically studied. Generally, dyes are adsorbed onto TiO2via three possible adsorption models: monodentate or bidentate bridging and chelating. Previous studies showed that the bidentate binding is the most favorable mode among the three possibilities.51–53 Therefore, we adopt a bidentate bridging configuration for all dyes absorbed onto the TiO2 surface in this work, and the corresponding optimized dye/(TiO2)16 system structures are shown in Fig. S2.† The calculated two Ti–O bonds at all dye/TiO2 interfaces are in the range of 2.02–2.12 Å, which is similar to the Ti–O bond lengths in bulk TiO2 (1.934–1.980 Å), implying that the dye is chemisorbed onto the TiO2 surface. The strong interactions between the dye and TiO2 will promote electron injection into the semiconductor.49 From Fig. S3,† it can clearly be seen that the HOMO levels of all dye/TiO2 complexes show no obvious change compared to their isolated dyes, while the LUMO decreased from 0.26 eV (WS5-GeS) to 0.62 eV (WS5-CL), indicating that the acceptor group has strong electronic coupling with the TiO2 surface. The energy gap of WS5/TiO2 (1.79 eV) is the highest while that of WS5-SiS/TiO2 (1.42 eV) is the lowest, which are lower than those of isolated dyes due to decreases in the LUMO. The transition energies, absorption peaks, oscillator strengths and major transitions of dyes binding on the TiO2 surface are tabulated in Table S2.† It is clear that the λmax of dye/TiO2 systems exhibits a slight red shift in comparison to isolated dyes. The electron density of FMOs of dyes/TiO2 in the major transition is shown in Fig. 10. WS5, WS5-CS, WS5-CL and WS5-SiS have similar electron densities of HOMO, HOMO−1 and HOMO−2, which are mainly localized over the whole molecule. The electronic distributions of the HOMO for WS5-SiL, WS5-GeL and WS5-HoS are mainly from the phenoxazine and furan groups. For WS5-GeS and WS5-HoL, the electronic distributions of HOMO−4 and HOMO−1 are centered on functionalized graphene. However, the lowest unoccupied molecular orbitals of all the dyes are completely located on the TiO2 cluster. This distribution characteristic indicates that all the dyes are promising candidates for DSSCs.
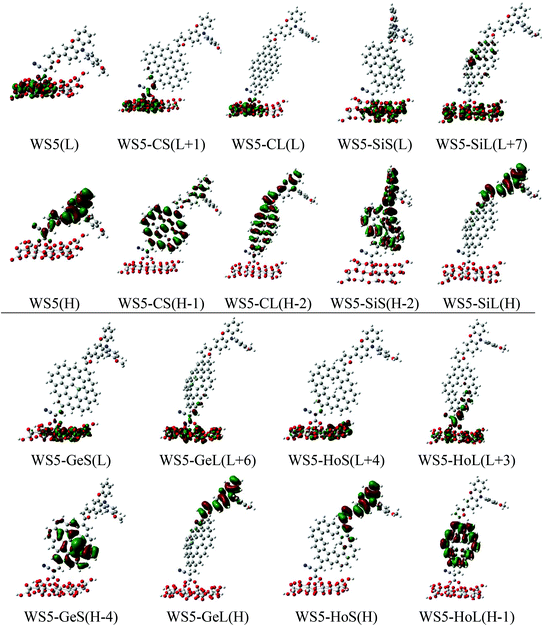 |
| Fig. 10 Distribution patterns of the FMOs for dye/TiO2 clusters in the major transition. | |
The collected electrons in the TiO2 conduction band can recombine with the electrolyte, which is the main charge recombination process and leads to a negative effect for DSSCs. The high concentration of iodine electrolyte close to the TiO2 surface would reduce the electron lifetime in TiO2 and accelerate the charge recombination. Previous simulations showed that using I2 to study electron recombination for dye-electrolyte is more realistic.54,55 Therefore, we consider only I2 as the electron acceptor in the charge recombination process. The optimized molecular structures of the dye/TiO2-I2 complex and corresponding binding energies are exhibited in Fig. 11. A more negative binding energy corresponds to a stronger interaction, which will lead to a higher iodine concentration around the TiO2 surface. WS5-HoS has the most negative binding energy, and other designed dyes possess smaller binding energies than that of WS5, indicating that using functionalized graphene to decorate the dye WS5 might result in an inhibitory effect on charge recombination except for WS5-HoS, possibly increasing VOC.
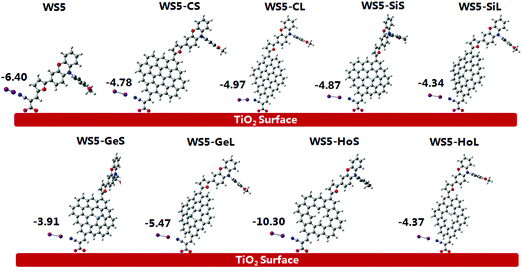 |
| Fig. 11 The optimized structures of the dye/TiO2-I2 complex and corresponding binding energies (unit in kcal mol−1). | |
4. Conclusion
To sum up, we performed a comprehensive theoretical investigation of how functionalized graphene affects the photovoltaic performance of a dye sensitizer in DSSCs. The ground- and excited-state properties of all the dyes were calculated via DFT and TD-DFT methodologies. Based on an analysis of electronic structures, intramolecular as well as interface charge transfer processes, and absorption properties, the main conclusions can be itemized as follows:
• The introduction of functionalized graphene in dye WS5 could decrease ionization potentials (IP) as well as chemical hardness (h), and increase electron affinities (EA), electron-accepting power (ω+) and electrophilicity index (ω), meaning that all the designed dyes will exhibit better photoelectrical properties. Among all the designed dyes, the prominent optical and electrical properties of dyes with short functionalized graphene over those of long functionalized graphene are due to smaller IP and h, and larger EA, ω+ and ω.
• Using graphene to decorate the dye WS5 could adjust the energy levels to narrow the energy gap. Dyes with short functionalized graphene exhibit the smallest HOMO–LUMO gaps, followed by long functionalized graphene and dye WS5. In addition, the energy gap decreases steadily with an enhancement in the electronegativity of doped atoms (C, Si and Ge) (such as WS5-CS/L > WS5-SiS/L > WS5-GeS/L).
• According to analysis of the intermolecular interaction between dye and I2, all the designed dyes possess a slow electron recombination rate except WS5-HoS, possibly increasing VOC.
• Jph increases steadily with an increase in the electronegativity of doped atoms (C, Si and Ge) in graphene. Moreover, the dyes with insertion of short functionalized graphene exhibit a superior performance in Jph to that of long functionalized graphene.
• All the designed dyes exhibit lower aromaticities compared to dye WS5, especially for dyes with short functionalized graphene, implying that inserting functionalized graphene facilitates electron ICT properties. Additionally, the aromaticities around the doped atoms decrease steadily with an increase in the electronegativity of doped atoms (C, Si and Ge) in graphene.
• For the absorption property, introducing functionalized graphene not only enhances absorption intensity, but also expands the absorption spectrum, which will lead to a better photocurrent response. The absorption spectrum of dyes with short functionalized graphene exhibits a bathochromic shift compared to long functionalized graphene.
Conflicts of interest
There are no conflicts to declare.
Acknowledgements
We are grateful for the financial support of this research from the National Natural Science Foundation of China (51779065 and 51579057) and the State Key Laboratory of Urban Water Resource and Environment, Harbin Institute of Technology (2019DX11).
References
- K. Kakiage, Y. Aoyama, T. Yano, K. Oya, J.-I. Fujisawa and M. Hanaya, Highly-efficient dye-sensitized solar cells with collaborative sensitization by silyl-anchor and carboxy-anchor dyes, Chem. Commun., 2015, 51(88), 15894–15897 RSC.
- I. Mihalache, A. Radoi, M. Mihaila, C. Munteanu, A. Marin and M. Danila,
et al., Charge and energy transfer interplay in hybrid sensitized solar cells mediated by graphene quantum dots, Electrochim. Acta, 2015, 153, 306–315 CrossRef CAS.
- C.-R. Zhang, Y. Zhang, X.-Y. Li, W. Wang, J.-J. Gong and Z.-J. Liu,
et al., The bis-dimethylfluoreneaniline organic dye sensitizers for solar cells: A theoretical study and design, J. Mol. Graphics Modell., 2019, 88, 23–31 CrossRef CAS.
- T. Umeyama, T. Hanaoka, H. Yamada, Y. Namura, S. Mizuno and T. Ohara,
et al., Exclusive occurrence of photoinduced energy transfer and switching of its direction by rectangular π-extension of nanographenes, Chem. Sci., 2019, 10(27), 6642–6650 RSC.
- G. Wang, P. He, A. Xu, Q. Guo, J. Li and Z. Wang,
et al., Promising Fast Energy Transfer System Between Graphene Quantum Dots and the Application in Fluorescent Bioimaging, Langmuir, 2019, 35(3), 760–766 CrossRef CAS.
- L. S. Panchakarla, K. S. Subrahmanyam, S. K. Saha, A. Govindaraj, H. R. Krishnamurthy and U. V. Waghmare,
et al., Synthesis, Structure, and Properties of Boron- and Nitrogen-Doped Graphene, Adv. Mater., 2009, 21(46), 4726–4730 CAS.
- J. Liu, X. Kang, X. He, P. Wei, Y. Wen and X. Li, Temperature-directed synthesis of N-doped carbon-based nanotubes and nanosheets decorated with Fe (Fe3O4, Fe3C) nanomaterials, Nanoscale, 2019, 11(18), 9155–9162 RSC.
- J. Liu, F. Li, W. Liu and X. Li, Effect of calcination temperature on the microstructure of vanadium nitride/nitrogen-doped graphene nanocomposites as anode materials in electrochemical capacitors, Inorg. Chem. Front., 2019, 6(1), 164–171 RSC.
- J. Liu, X. Ren, X. Kang, X. He, P. Wei and Y. Wen,
et al., Fabrication of nitrogen-rich three-dimensional porous carbon composites with nanosheets and hollow spheres for efficient supercapacitors, Inorg. Chem. Front., 2019, 6(8), 2082–2089 RSC.
- J. Ebrahimi, M. G. Ahangari and M. Jahanshahi, Computational studies at the density functional theory (DFT) level about the surface functionalization of hexagonal monolayers by chitosan monomer, Appl. Surf. Sci., 2018, 440, 778–789 CrossRef CAS.
- Y. Dai, Z. Li and J. Yang, Distinct molecule adsorption behaviors on warped nanographene C80H30: A theoretical study, Carbon, 2016, 100, 428–434 CrossRef CAS.
- L.-J. He, J. Wang, J. Chen, R. Jia and H.-X. Zhang, The effect of relative position of the π-spacer center between donor and acceptor on the overall performance of D-π-A dye: a theoretical study with organic dye, Electrochim. Acta, 2017, 241, 440–448 CrossRef CAS.
- X. Xie, Z.-H. Liu, F.-Q. Bai and H.-X. Zhang, Performance Regulation of Thieno[3,2-b]benzothiophene π-Spacer-Based D-π-A Organic Dyes for Dye-Sensitized Solar Cell Applications: Insights From Computational Study, Front. Chem., 2019, 6, 676 CrossRef.
- Y. Fu, T. Lu, Y. Xu, M. Li, Z. Wei and H. Liu,
et al. Theoretical screening and design of SM315-based porphyrin dyes for highly efficient dye-sensitized solar cells with near-IR light harvesting, Dyes Pigm., 2018, 155, 292–299 CrossRef CAS.
- S. Jiang, X. Lu, G. Zhou and Z.-S. Wang, Charge transfer in cross conjugated 4,8-dithienylbenzo[1,2-b:4,5-b′]dithiophene based organic sensitizers, Chem. Commun., 2013, 49(37), 3899–3901 RSC.
- Y. Li, J. Liu, D. Liu, X. Li and Y. Xu, D-A-π-A based organic dyes for efficient DSSCs: A theoretical study on the role of π-spacer, Comput. Mater. Sci., 2019, 161, 163–176 CrossRef CAS.
- J. H. Bae, S. J. Lim, J. Choi, S. B. Yuk, J. W. Namgoong and J. H. Ko,
et al. Effects of introducing functional groups on the performance of phenoxazine-based dye-sensitized solar cells, Dyes Pigm., 2019, 162, 905–915 CrossRef CAS.
- C. Moreno, M. Vilas-Varela, B. Kretz, A. Garcia-Lekue, M. V. Costache and M. Paradinas,
et al. Bottom-up synthesis of multifunctional nanoporous graphene, Science, 2018, 360(6385), 199 CrossRef CAS.
- M. Tripathi, A. Markevich, R. Böttger, S. Facsko, E. Besley and J. Kotakoski,
et al., Implanting Germanium into Graphene, ACS Nano, 2018, 12(5), 4641–4647 CrossRef CAS.
- S. S. Lin, Light-Emitting Two-Dimensional Ultrathin Silicon Carbide, J. Phys. Chem. C, 2012, 116(6), 3951–3955 CrossRef CAS.
- S. Lin, S. Zhang, X. Li, W. Xu, X. Pi and X. Liu,
et al., Quasi-Two-Dimensional SiC and SiC2: Interaction of Silicon and Carbon at Atomic Thin Lattice Plane, J. Phys. Chem. C, 2015, 119(34), 19772–19779 CrossRef CAS.
- J. Hu, C. Ouyang, S. A. Yang and H. Y. Yang, Germagraphene as a promising anode material for lithium-ion batteries predicted from first-principles calculations, Nanoscale Horiz., 2019, 4(2), 457–463 RSC.
- Z. Shi, Z. Zhang, A. Kutana and B. I. Yakobson, Predicting Two-Dimensional Silicon Carbide Monolayers, ACS Nano, 2015, 9(10), 9802–9809 CrossRef CAS.
- G. Gao, N. W. Ashcroft and R. Hoffmann, The Unusual and the Expected in the Si/C Phase Diagram, J. Am. Chem. Soc., 2013, 135(31), 11651–11656 CrossRef CAS.
- J. Tirado-Rives and W. L. Jorgensen, Performance of B3LYP Density Functional Methods for a Large Set of Organic Molecules, J. Chem. Theory Comput., 2008, 4(2), 297–306 CrossRef CAS.
- M. N. Glukhovtsev, R. D. Bach and C. J. Nagel, Performance of the B3LYP/ECP DFT Calculations of Iron-Containing Compounds, J. Phys. Chem. A, 1997, 101(3), 316–323 CrossRef CAS.
- J. E. Del Bene, W. B. Person and K. Szczepaniak, Properties of Hydrogen-Bonded Complexes Obtained from the B3LYP Functional with 6-31G(d,p) and 6-31+G(d,p) Basis Sets: Comparison with MP2/6-31+G(d,p) Results and Experimental Data, J. Phys. Chem., 1995, 99(27), 10705–10707 CrossRef CAS.
- J.-D. Chai and M. Head-Gordon, Systematic optimization of long-range corrected hybrid density functionals, J. Chem. Phys., 2008, 128(8), 084106 CrossRef.
- T. Yanai, D. P. Tew and N. C. Handy, A new hybrid exchange–correlation functional using the Coulomb-attenuating method (CAM-B3LYP), Chem. Phys. Lett., 2004, 393(1), 51–57 CrossRef CAS.
- A. D. Boese and J. M. L. Martin, Development of density functionals for thermochemical kinetics, J. Chem. Phys., 2004, 121(8), 3405–3416 CrossRef CAS.
- C. Adamo and V. Barone, Exchange functionals with improved long-range behavior and adiabatic connection methods without adjustable parameters: The mPW and mPW1PW models, J. Chem. Phys., 1998, 108(2), 664–675 CrossRef CAS.
- C. Lee, W. Yang and R. G. Parr, Development of the Colle-Salvetti correlation-energy formula into a functional of the electron density, Phys. Rev. B: Condens. Matter Mater. Phys., 1988, 37(2), 785–789 CrossRef CAS.
- J. Tomasi, B. Mennucci and R. Cammi, Quantum Mechanical Continuum Solvation Models, Chem. Rev., 2005, 105(8), 2999–3094 CrossRef CAS.
- T. Lu and F. Chen, Multiwfn: A multifunctional wavefunction analyzer, J. Comput. Chem., 2012, 33(5), 580–592 CrossRef CAS.
- P. Persson, J. C. M. Gebhardt and S. Lunell, The Smallest Possible Nanocrystals of Semiionic Oxides, J. Phys.
Chem. B, 2003, 107(15), 3336–3339 CrossRef CAS.
- J. K. Roy, S. Kar and J. Leszczynski, Electronic Structure and Optical Properties of Designed Photo-Efficient Indoline-Based Dye-Sensitizers with D-A-π-A Framework, J. Phys. Chem. C, 2019, 123(6), 3309–3320 CrossRef CAS.
- M. Guo, R. He, Y. Dai, W. Shen, M. Li and C. Zhu,
et al. Electron-Deficient Pyrimidine Adopted in Porphyrin Sensitizers: A Theoretical Interpretation of π-Spacers Leading to Highly Efficient Photo-to-Electric Conversion Performances in Dye-Sensitized Solar Cells, J. Phys. Chem. C, 2012, 116(16), 9166–9179 CrossRef CAS.
- P. Li, Y. Cui, C. Song and H. Zhang, A systematic study of phenoxazine-based organic sensitizers for solar cells, Dyes Pigm., 2017, 137, 12–23 CrossRef CAS.
- P. Li, Z. Wang, C. Song and H. Zhang, Rigid fused π-spacers in D-π-A type molecules for dye-sensitized solar cells: a computational investigation, J. Mater. Chem. C, 2017, 5(44), 11454–11465 RSC.
- P. J. Hay and W. R. Wadt, Ab initio effective core potentials for molecular calculations. Potentials for K to Au including the outermost core orbitals, J. Chem. Phys., 1985, 82(1), 299–310 CrossRef CAS.
-
M. J. Frisch, G. W. Trucks, H. B. Schlegel, G. E. Scuseria, M. A. Robb, J. R. Cheeseman, G. Scalmani, V. Barone, B. Mennucci, G. A. Petersson, H. Nakatsuji, M. Caricato, X. Li, H. P. Hratchian, A. F. Izmaylov, J. Bloino, G. Zheng, J. L. Sonnenberg, M. Hada, M. Ehara, K. Toyota, R. Fukuda, J. Hasegawa, M. Ishida, T. Nakajima, Y. Honda, O. Kitao, H. Nakai, T. Vreven, J. A. Montgomery Jr., J. E. Peralta, F. Ogliaro, M. Bearpark, J. J. Heyd, E. Brothers, K. N. Kudin, V. N. Staroverov, T. Keith, R. Kobayashi, J. Normand, K. Raghavachari, A. Rendell, J. C. Burant, S. S. Iyengar, J. Tomasi, M. Cossi, N. Rega, J. M. Millam, M. Klene, J. E. Knox, J. B. Cross, V. Bakken, C. Adamo, J. Jaramillo, R. Gomperts, R. E. Stratmann, O. Yazyev, A. J. Austin, R. Cammi, C. Pomelli, J. W. Ochterski, R. L. Martin, K. Morokuma, V. G. Zakrzewski, G. A. Voth, P. Salvador, J. J. Dannenberg, S. Dapprich, A. D. Daniels, O. Farkas, J. B. Foresman, J. V. Ortiz, J. Cioslowski and D. J. Fox, Gaussian 09, Revision D.01, Gaussian, Inc., Wallingford, CT, 2013 Search PubMed.
- N. Wazzan and A. Irfan, Theoretical study of triphenylamine-based organic dyes with mono-, di-, and tri-anchoring groups for dye-sensitized solar cells, Org. Electron., 2018, 63, 328–342 CrossRef CAS.
- G. Zhang, Y. Bai, R. Li, D. Shi, S. Wenger and S. M. Zakeeruddin,
et al. Employ a bisthienothiophene linker to construct an organic chromophore for efficient and stable dye-sensitized solar cells, Energy Environ. Sci., 2009, 2(1), 92–95 RSC.
- M. Grätzel, Photoelectrochemical cells, Nature, 2001, 414(6861), 338–344 CrossRef.
- H. Fallah-Bagher-Shaidaei, C. S. Wannere, C. Corminboeuf, R. Puchta and P. Schleyer, Which NICS Aromaticity Index for Planar π Rings Is Best?, Org.
Lett., 2006, 8(5), 863–866 CrossRef CAS.
- M. K. Nazeeruddin, A. Kay, I. Rodicio, R. Humphry-Baker, E. Mueller and P. Liska,
et al. Conversion of light to electricity by cis-X2bis(2,2′-bipyridyl-4,4′-dicarboxylate)ruthenium(II) charge-transfer sensitizers (X = Cl-, Br-, I-, CN-, and SCN-) on nanocrystalline titanium dioxide electrodes, J. Am. Chem. Soc., 1993, 115(14), 6382–6390 CrossRef CAS.
- L.-J. He, J. Chen, F.-Q. Bai, R. Jia, J. Wang and H.-X. Zhang, Fine-tuning π-spacer for high efficiency performance DSSC: A theoretical exploration with D-π-A based organic dye, Dyes Pigm., 2017, 141, 251–261 CrossRef CAS.
- M. Grätzel, Solar Energy Conversion by Dye-Sensitized Photovoltaic Cells, Inorg. Chem., 2005, 44(20), 6841–6851 CrossRef.
- L.-J. He, Y. Sun, W. Li, J. Wang, M.-X. Song and H.-X. Zhang, Highly-efficient sensitizer with zinc porphyrin as building block: Insights from DFT calculations, Sol. Energy, 2018, 173, 283–290 CrossRef CAS.
- L.-J. He, J. Chen, F.-Q. Bai, R. Jia, J. Wang and H.-X. Zhang, The influence of a dye–TiO2 interface on DSSC performance: a theoretical exploration with a ruthenium dye, RSC Adv., 2016, 6(85), 81976–81982 RSC.
- M. Khoudiakov, A. R. Parise and B. S. Brunschwig, Interfacial Electron Transfer in FeII(CN)64-−Sensitized TiO2 Nanoparticles: A Study of Direct Charge Injection by Electroabsorption Spectroscopy, J. Am. Chem. Soc., 2003, 125(15), 4637–4642 CrossRef CAS.
- X.-Q. Gong, A. Selloni and A. Vittadini, Density Functional Theory Study of Formic Acid Adsorption on Anatase TiO2(001): Geometries, Energetics, and Effects of Coverage, Hydration, and Reconstruction, J. Phys. Chem. B, 2006, 110(6), 2804–2811 CrossRef CAS.
- S. Feng, Z.-Z. Sun and Q. Li, How to screen a promising anchoring group from heterocyclic components in dye sensitized solar cell: A theoretical investigation, Electrochim. Acta, 2019, 296, 545–554 CrossRef CAS.
- A. N. M. Green, R. E. Chandler, S. A. Haque, J. Nelson and J. R. Durrant, Transient Absorption Studies and Numerical Modeling of Iodine Photoreduction by Nanocrystalline TiO2 Films, J. Phys. Chem. B, 2005, 109(1), 142–150 CrossRef CAS PubMed.
- M. Xie, J. Wang, F.-Q. Bai, L. Hao and H.-X. Zhang, Discovering the intermediate of dye regeneration in dye-sensitized solar cells: Theoretical investigations on the interaction between organic dye with different donors and X−/X3− (X = I, Br), Dyes Pigm., 2015, 120, 74–84 CrossRef CAS.
Footnote |
† Electronic supplementary information (ESI) available. See DOI: 10.1039/c9qi01264h |
|
This journal is © the Partner Organisations 2020 |
Click here to see how this site uses Cookies. View our privacy policy here.