DOI:
10.1039/C9RA07424D
(Paper)
RSC Adv., 2020,
10, 1181-1190
Bi2O3 and g-C3N4 quantum dot modified anatase TiO2 heterojunction system for degradation of dyes under sunlight irradiation
Received
15th September 2019
, Accepted 9th December 2019
First published on 7th January 2020
Abstract
A facile and feasible method was successfully utilized to incorporate Bi2O3 and g-C3N4 quantum dots on TiO2 surface to synthesize a novel composite g-C3N4/TiO2/Bi2O3. The photocatalytic activity of the composite g-C3N4/TiO2/Bi2O3 for degradation of dyes under sunlight and UV light irradiation was evaluated. It possessed the higher photocatalytic performance than that of pristine TiO2 or g-C3N4 under the same conditions. Under sunlight irradiation, the reaction rate constants of the g-C3N4/TiO2/Bi2O3 was about 4.2 times and 3.3 times higher than that of TiO2 and g-C3N4, respectively. The promising photocatalytic performance was attributed to the broader light absorption range and efficient separation of photoinduced carriers. Moreover, based on the TEM, XPS, XRD, UV-vis spectrum, radicals scavenging test and Mott–Schottky analysis systematic mechanism for photodegradation process was proposed. This work provides a promising strategy for the modification of TiO2-based semiconductors by incorporating different quantum dots and promoting the efficiency of the photocatalysts in practical application.
1 Introduction
At present, environmental contamination and energy shortage are the most serious problems on our planet. Hence, there is an urgent need for the development of an environmental, sustainable, economical and energy-saving technology for contamination control. Semiconductor photocatalysis provides an optimal solution for the problems with the potential that the clean solar energy can be harnessed for decomposition of pollutants.1 To date, TiO2 as the photocatalyst has drawn significant attention and been widely studied because of its desirable properties including high reactivity, chemical stability, nontoxicity, and cheap price.2–7 Nevertheless, the application of TiO2-based photocatalysts is hindered due to its inefficient utilization of solar energy, poor charge separation, and low adsorption of organic contaminants on the surface. Besides these intrinsic drawbacks, the complicated process of synthesis is another key factor to impede its practical application.8
To avoid the above limitation, various modification strategies have been carried out, such as dye-sensitization, metal or non-metal doping, and transition metal doping, etc. Particularly, compositing TiO2 with different semiconductor into heterojunction is an efficient method to enhance the photocatalytic activity.8 Since the heterojunction structure would decrease the recombination of electron/hole by separating the charge carriers to different semiconductors surface due to the different potential barriers. These heterojunctions-based semiconductors exhibit advanced efficiency for degrading pollutants.
Presently, considerable attention has been paid to graphitic carbon nitride (g-C3N4), which is a stable kind of polymers with a layered structure like graphene. Particularly, it possesses the strong ability to harvest solar energy due to its narrow band gap (Eg = 2.7 eV). Nevertheless, the quantum efficiency of pure g-C3N4 is low because of the high recombination ratio of photogenerated electron/hole pair.9 Combining the TiO2 with g-C3N4 in heterojunction is considered as an efficient method which can improve the absorption of visible light and the separation of photogenerated pairs. Yu et al.10 synthesized g-C3N4–TiO2 composites by calcination process using P25 and urea, achieving the high photoactivity for the degradation of HCHO. Lei et al.11 reported the synthesis of g-C3N4/TiO2 photocatalyst via simple calcination using cyanamide and anatase TiO2 as precursor. The g-C3N4/TiO2 photocatalyst exhibits a promising progress in degradation of the dye Acid Orange 7 under both visible and UV light. The g-C3N4–TiO2 composites with prominent photoactivity for the degradation of phenol under UV light was synthesized by Colo'n et al.12 by impregnation. Zhang et al.13 found that well-dispersed TiO2 nanocrystals with (001) facets prepared in situ on g-C3N4 through a solvothermal method exhibit higher efficiency for photocatalytic degradation of phenol as compared to pure g-C3N4 and TiO2. Wu et al.14 prepared nanosheets TiO2/g-C3N4 composite by solvothermal method, which exhibits a significant improvement in photodegradation towards methylene blue under visible light irradiation than pristine g-C3N4 and TiO2.
Among strategies of constructing heterojunction, synthesis of p–n junction is considered as an effective method to facilitate the photoactivity by inducing the separation of photogenerated carries due to the existence of an internal electric field. Bismuth oxide (Bi2O3), as a p-type semiconductor, has been extensively studied due to its suitable band gap (Eg = 2.8 eV) and visible light driven catalytic activity. Among the six different polymorphic phases of Bi2O3, β-Bi2O3 has prominent advantages for degradation of pollutants. Since TiO2 is a n-type semiconductor, it is feasible to combine TiO2 with β-Bi2O3, by which catalytic efficiency can be notably enhanced.15–18 Recently, much efforts have been made to synthesize TiO2/Bi2O3 heterojunction. Various methods have been employed such as: pulse electro-deposition,19 hydrothermal, sol–gel, and coprecipitation.20–26 However, the complexity of these methods limits the application of photocatalysis.
Instead of above strategies, researchers have succeeded in sensitizing TiO2 by modifying quantum dots (QDs) of low-band gap materials such as g-C3N4, CdS, CdSe, CdTe, and Bi-based materials, which can absorb light in the visible region. QDs can match the solar spectrum better due to the particle size effect. Additionally, QDs are recently reported to generate multiple excitons, which can improve the photocatalysis efficiency.27–30 Jiao et al.31 firstly prepared Bi2O3 quantum dots decorated TiO2 with exposed {001} facets on graphene sheets. Size-controllable were in situ synthesized on TiO2 nanotube arrays and high activity in synergetic H2 evolution and organics degradation.32
In this study, we firstly use a simple ball-milling/calcination method to synthesize a g-C3N4/TiO2/Bi2O3 heterojunction system which distinctly exhibit a promising synergetic improvement effect. The different properties of the catalyst are evaluated by the degradation of simulated dyeing wastewater under UV light and direct sunlight irradiation. While the different properties are confirmed by TEM, XPS, XRD and UV-vis diffuse reflection spectra. A tentative mechanism for photocatalytic degradation of RhB by the composite g-C3N4/TiO2/Bi2O3 is proposed.
2 Materials and methods
2.1 Catalysts synthesis
2.1.1. Chemicals and reagents. Double distilled water was used throughout the experiment. The catalyst precursor used in this experiment including titanium dioxide (TiO2, anatase, 99.8%; Shanghai Aladdin Biochemical Technology Co., Ltd), melamine (98%; Shanghai Aladdin Biochemical Technology Co., Ltd), bismuth(III) nitrate-pentahydrate (Bi(NO3)3·5H2O, 98%; Shanghai Aladdin Biochemical Technology Co., Ltd). All the reagents utilized were of analytical grade and without further purification.
2.1.2 Methods.
1. Synthesis of pure g-C3N4. 5 g of melamine were added into crucible with a cover then heated at 520 °C under air atmosphere for 2 h with a heating rate of 5 °C min−1. After cooling to the room temperature naturally, the resulting yellow bulks were collected and ball-milled into powders for further evaluation.
2. Synthesis of pure Bi2O3. 3 g Bi(NO3)3·5H2O were added into crucible with a cover then heated at 520 °C under air atmosphere for 2 h with a heating rate of 5 °C min−1. After cooling to the room temperature naturally, the resulting bulks were collected and ball-milled into powders for further evaluation.
3. Synthesis of g-C3N4/TiO2/Bi2O3. The g-C3N4/TiO2/Bi2O3 catalysts were prepared by the processes of ball-milling and calcination. Firstly, 400 mg of anatase TiO2 powders and 600 mg of melamine were added into the agate ball milling tank, followed by the process of ball milling for 2 h with a ball powder ratio of 10
:
1, at a speed of 400 rpm in a planetary ball mill. Then, a certain amount of Bi(NO3)3·5H2O were added into the agate ball milling tank which was filled with TiO2 and melamine. The powders mixed by ball milling for 2 h with a ball powder ratio of 10
:
1, at a speed of 400 rpm in a planetary ball mill. Subsequently, the mixed powder was placed into a crucible with a cover then heated at 520 °C under air atmosphere for 2 h with a heating rate of 5 °C min−1. After cooling to the room temperature naturally, the resulting yellow bulks were collected and ball-milled into powders for further evaluation. The obtained powers was named as TCB-x, where the x refers to the weight percentage of Bi(NO3)3·5H2O to the weight of TiO2 and melamine.
2.2 Characterization
The crystal phases was characterized by X-ray diffraction (XRD, Bruker D8 ADVANCE A25X) with a Cu Kα radiation with a diffraction angle between 10–80°
Transmission electron microscopy (TEM) and high-resolution transmission electron microscopy (HRTEM) were measured by Tecnai G2 F20 S-TWIN (200 kV). The samples were dispersed in ethanol and dropped on copper grids. Chemical composition and valence band (VB) were observed by X-ray photoelectron spectroscopy (XPS, Escalab 250Xi) using a monochromatic micro-focused Al Kα (1486. 6 eV) source. UV-vis diffuse reflectance spectroscopy (UV-vis DRS) absorption spectra of the wavelength between 200 nm and 800 nm were carried out at a Shimadzu UV-3600 spectrophotometer using BaSO4 as a reference.
The electrochemical studies were conducted on an electrochemical workstation (CHI660C, CH Instrument Corp, Shanghai), which used catalyst-deposited FTO glass as working electrode, Pt as the counter electrode and Ag/AgCl as the reference electrode. Meanwhile, 0.5 M Na2SO4 was served as the electrolyte solution. The working electrode was prepared as follows: 20 mg PEG-600 and 10 mg pure TiO2 and Bi2O3 were dispersed in 1 mL ethanol and ultrasonically scattered for 1 h. Then, the suspension above was added onto the FTO glass (1 × 1 cm) and evaporated to dry. The Mott–Schottky measurement was performed at frequency of 1000 Hz.
2.3 Photocatalytic activity test
The aqueous Rhodamine B (RhB), methylene blue (MB) and methyl orange (MO) dyes were used as model organic contaminants to evaluate the photocatalytic activities of the synthesized catalysts. Degradation experiments were carried out under UV and direct solar light irradiation. A high-pressure 300 W mercury lamp with the radiation of 365 nm was used as the UV light source. To study the photocatalytic activity of the samples under visible light, the photoreactor were directly exposed to sunlight. And the illumination intensity was measured by solar power meter (TES-1333R). 50 mg catalyst powders were suspended in 50 mL aqueous organic dye solution and the dyes concentration was 20 mg L−1. To reach adsorption–desorption equilibrium, the solution was stirred for 60 min in the dark. At given time intervals, 4 mL aliquots were taken and centrifuged at 10
000 rpm for 10 min to remove the particles. Then, the concentration of organic dyes in filtrates was analyzed using an Alpha-1506 UV-vis spectroscopy (Shanghai Lab-Spectrum Instruments Co. Ltd, China) at the wavelength of 554 nm, 664 nm, 465 nm for RhB, MB, MO, respectively. The experiments were repeated 3 times.
3 Result and discussion
3.1 XRD characterization
Fig. 1 shows the XRD patterns of TCB-30%, pristine TiO2, g-C3N4 and Bi2O3. In TCB-30% patterns, the diffraction peaks at 25.3°, 37.9°, 48.0°, 53.9° could be well indexed to the (101), (004), (200) and (105) planes of the anatase phase TiO2.23,33 There are four peaks at 27.9°, 31.8°, 32.8°, 46.3°, corresponding to the (211), (002), (220), and (222) plane of pristine Bi2O3.26,34,35 The characteristic peaks of g-C3N4 at 27.5° (ref. 27 and 36) was also detected in TCB-30% patterns. The diffraction peaks with relatively low intensity indicated the small sized Bi2O3 and TiO2 quantum dots, in consistent with the results of TEM. The well crystallized diffraction peaks detected in TCB-30% patterns suggested the well crystalline structure of anatase TiO2, g-C3N4 and Bi2O3, without the generation of other crystal structure.
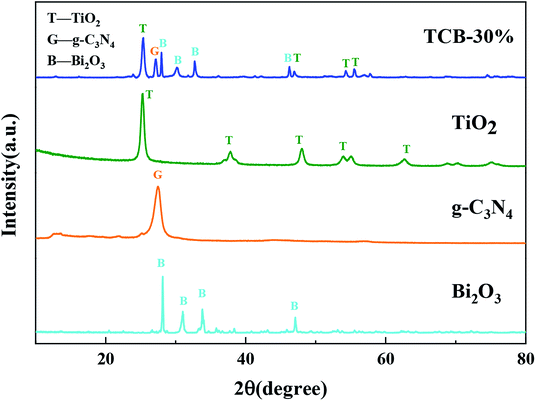 |
| Fig. 1 XRD pattern of TCB-30%, TiO2, g-C3N4 and Bi2O3. | |
3.2 Microstructure analysis
The morphology of the prepared catalysts was characterized by TEM and high-resolution. TEM image Fig. 2b, c and d are enlarged views of Fig. 2a. As shown in Fig. 2a, TiO2 particles have a spherical structure with an average size between 30–60 nm, which was in well accordance with the crystallite size obtained from Scherrer equation. Bi2O3 and g-C3N4 showed a much smaller size below 10 nm, and intimately cover on the surface of TiO2 particles, which intensively increase the specific surface area of TiO2.
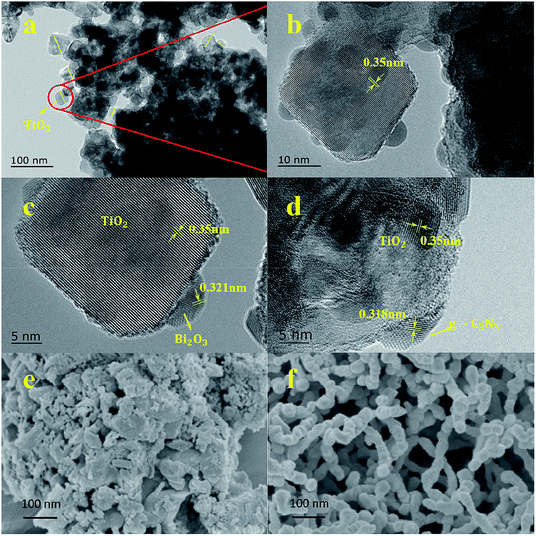 |
| Fig. 2 (a) Selected TEM figure of TCB-30%, (b, c and d) HRTEM figures of TCB-30%, (e) SEM figure of pure g-C3N4, (f) SEM figure of pure Bi2O3. | |
Clear lattice fringes for the identification of crystallographic spacing could be observed in Fig. 2c, and the lattice spacing of 0.350 nm and 0.321 nm corresponded well with (101) phase of TiO2 and (221) phase of Bi2O3,15–17 respectively. In Fig. 2d, the lattice spacing of 0.318 nm matched the (002) crystal phase of g-C3N4.37–39
Thus, these TEM images indicated the interaction of g-C3N4/TiO2/TiO2 heterojunction composite. The g-C3N4 and Bi2O3 located on the surface of TiO2 as quantum dots. In Fig. 2e, the pure g-C3N4 showed aggregated morphologies, which were comprised of block-based flakiness. Fig. 2f displayed a spheroidal structure of Bi2O3. It was obvious that the process of ball-milling played a significant role in synthesizing g-C3N4 and Bi2O3 quantum dots on TiO2 surface.
3.3 Chemical compositions
Fig. 3 showed the full survey and high-resolution spectra for the Ti 2p, O 1s, C 1s, N 1s and Bi 4f region. Fig. 3b depicts the C 1s of the prepared. The peak centered at 284.6 eV could be assigned to the adventitious carbon while the peak at 287.5 eV belongs to the N–C
N group of the g-C3N4.40–42 Fig. 3d showed N 1s spectrum of catalyst. The N 1s peak was fitted into two peaks at 399.3 eV and 400.2 eV, which were the sp2-hybridized nitrogen (C
N–C) and tertiary nitrogen (N-3), respectively.40–42 Furthermore, two fitted peaks of Ti 2p3/2 and Ti 2p1/2 at 458.9 eV and 464.4 eV suggested the presence of Ti(IV).27,36,43 In Fig. 3a, two fitted peaks at 158.9 eV and 164.3 eV belonged to Bi 4f5/2 and Bi 4f7/2, suggesting the existence of Bi2O3 in the catalyst.24,34,35 These results suggested that the composite TCB-30% consisted of g-C3N4, anatase TiO2 and Bi2O3.
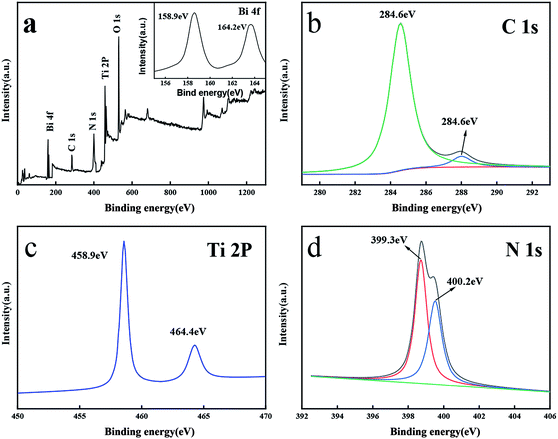 |
| Fig. 3 XPS spectrum of (a) TCB-30%, (b) C 1s, (c) Ti 2P, (d) N 1s. | |
3.4 UV-vis DRS analyses
The UV-vis diffuse reflectance spectra of different composites were exhibited in Fig. 4. It is obvious that the composite of TCB-30% showed a significant red shift of band edge to 456.5 nm compared with the initial TiO2, Bi2O3 and g-C3N4. There is also an intensively long tailing absorption in visible region, which can be explained by the synergistic effects of Bi2O3 and g-C3N4 quantum dots with a narrower band gap.
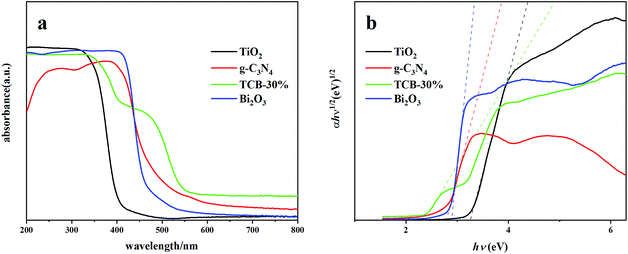 |
| Fig. 4 (a) UV-vis DRS spectra and (b) optical bandgap of the TCB-30%, TiO2, g-C3N4 and Bi2O3. | |
The band gap energies were determined using the equation below:
where
A,
h,
v,
Eg and
α are the absorption coefficient, Planck's constant, frequency, band gap energy and a constant, respectively. For the composites in this study,
n is 4 because of the indirect transition. Therefore, Tauc's plots of (
αhν)
1/2 versus photon energy (
hν) are obtained. As shown in
Fig. 4b, the band gap energies of TiO
2, TCB-30%, Bi
2O
3 and g-C
3N
4 are 3.20 eV, 2.24 eV, 2.80 eV and 2.7 eV, respectively. The TCB-30% has a lower band gap energy compared to TiO
2, which may be due to the heterojunction structure of the composite.
In order to further explain the photocatalytic activity of the ternary composite, the band structure of Bi2O3 was explored according to the following empirical equation.
where
EVB is the valence band edge potentials, E
CB is the conduction band energy,
X is the electronegativity of semiconductor,
Ee is the energy value of free electrons on the hydrogen scale,
Eg is gap energy of semiconductor. The value of
X for Bi
2O
3 is
ca. 5.99 eV. The calculated conduction band and valence band of Bi
2O
3 are 0.33 eV and 3.13 eV, respectively. The band structure of TiO
2 and g-C
3N
4 was calculated in above-mentioned method as well. And the results were showed in
Table 1.
Table 1 Band structure of Bi2O3, TiO2 and g-C3N4
Catalyst |
Band gap (eV) |
Valence band (VB) |
Conduction band (CB) |
TiO2 |
3.2 |
2.7 |
−0.5 |
g-C3N4 |
2.7 |
1.4 |
−1.3 |
TCB-30% |
2.2 |
|
|
Bi2O3 |
2.8 |
3.13 |
0.33 |
3.5 Photocatalytic activity
Fig. 5 displays the change of RhB concentration versus irradiation time with the pure TiO2, g-C3N4 or TCB-x under UV irradiation and sunlight irradiation. From Fig. 5a, pure TiO2 and pure g-C3N4 exhibited limited degradation rate of RhB under the UV light, which were only 66.9% and 62.1% after 50 min irradiation respectively. With the incorporation of Bi2O3 and construction of heterojunction, the photocatalytic activity substantially enhanced. In particular, the TCB-30% displayed the highest degradation rate of 96.5%. Moreover, TCB-30% showed the best photodegradation ability on RhB (completely degraded within 120 min) under sunlight irradiation (about 640 W m−2).
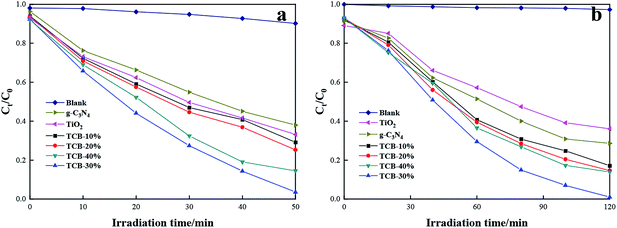 |
| Fig. 5 Photocatalytic activity of TCB-30%, TiO2 and g-C3N4 for degradation of RhB under (a) UV light irradiation (b) sunlight irradiation. | |
The degradation of RhB followed the first-order kinetics, which can be expressed by the equation: ln(C0/Ct) = ka−1t, where C0 and Ct is RhB concentration at time 0 and time t, and ka−1 represents the first-order reaction rate constant. As shown in Fig. 6, TCB-30% displayed a maximum value of rate constant under UV, which was about 2.2 times as high as that of TiO2 and 2.6 times as that of g-C3N4. When under the solar irradiation (640 W m−2), the ka−1 of TCB-30% was 4.2 times and 3.3 times higher than that of TiO2 and g-C3N4 respectively. Therefore, the improvement of photocatalytic activity under solar irradiation is more remarkable than that in the condition of UV light.
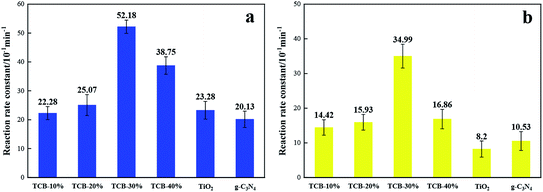 |
| Fig. 6 Reaction rate constant for degradation RhB of TCB-30%, TiO2, g-C3N4 and Bi2O3 under (a) UV light irradiation (b) sunlight irradiation. | |
Moreover, TCB-30% was also used to degrade different kinds of dyes with the same initial concentration under sunlight irradiation, including methylene blue (MB) and methyl orange (MO). As it shown in Fig. 7a, TCB-30% displayed a limited degradation rate for methylene blue and methyl orange compared with RhB. Fig. 8 showed the dark adsorption of three dyes over TCB-30%. It was supposed that the promising reaction rate could be contributed by the strong adsorption of the prepared heterojunction materials towards organic dyes with hydroxy groups. Thus, it is worth further research on how the surface groups of this composite affects the reaction with organic pollutants.
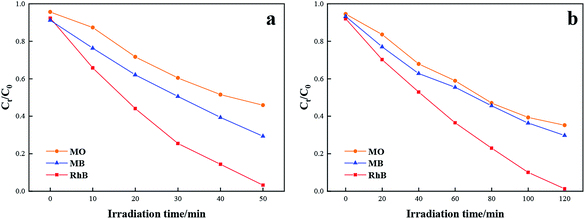 |
| Fig. 7 Comparison of the photocatalytic activity towards degradation of RhB, MB and MO under (a) UV light irradiation (b) sunlight irradiation. | |
 |
| Fig. 8 Dark adsorption of three dyes over TCB-30%. | |
To figure out the contribution of photo-induced active radical in the reaction process, benzoquinone (BQ), isopropanol (IPA) and ammonium oxalate (AO) were applied as scavengers of superoxide radical (·O2−), hydroxyl radical (·OH) and hole (h+), respectively. The comparison experiments were carried out in the presence of the same molar concentration of scavengers (BQ, IPA and AO) for visible light degradation of RhB with TCB-30%. As displayed in Fig. 9, the degradation rate without any scavengers is 99.6%. By contrast, by adding BQ and IPA, the degradation rate sharply suppressed. While with the addition of AO scavengers, there is only a slight decrease of degradation rate. Therefore, it can be concluded that superoxide radical (·O2−) and hydroxyl radical (·OH) play key roles in degradation towards RhB under visible light.
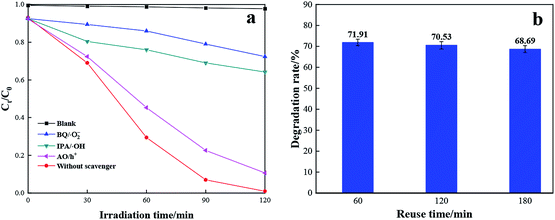 |
| Fig. 9 Radical capture test (a) and stability test of TCB-30% for degradation of RhB (b) under sunlight irradiation. | |
During the practical application of catalysts, the by-products tend to be adsorbed on the active sites of photocatalysts' surface, leading to the dramatic decrease of the photocatalytic activity. To evaluate the stability of the prepared TCB-30%, recycle experiments were conducted under solar irradiation. As shown in the Fig. 9, the TCB-30% still presents the excellent photocatalytic performance after three cycles.
3.6 Mechanism discussion
In order to investigate the electrochemical properties of TiO2 and Bi2O3, Mott–Schottky plots were obtained. In Fig. 10a, TiO2 exhibit a positive slope indicating that it’s n-type semiconductor. On the contrary, BiO2 shows a negative slope, which indicts a p-type semiconductor.
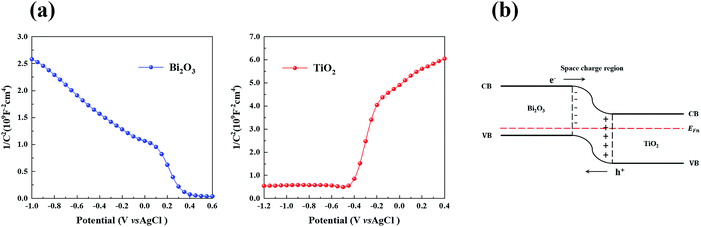 |
| Fig. 10 (a) Mott–Schottky plots of Bi2O3 and TiO2 in 0.5 M Na2SO4 at a frequency of 1 kHz, and (b) energy band structure of p-type Bi2O3/n-type TiO2. | |
Based on the results of XRD, XPS, TEM and UV-vis DRS analysis and Mott–Schottky, a tentative heterojunction system proposed and depicted in Scheme 1. TiO2 is placed as a support and connector of Bi2O3 and g-C3N4 quantum dots. The promising photocatalytic performance of TCB-30% under visible light is significantly related to the extended light adsorption spectrum and enhanced electron–hole separation.
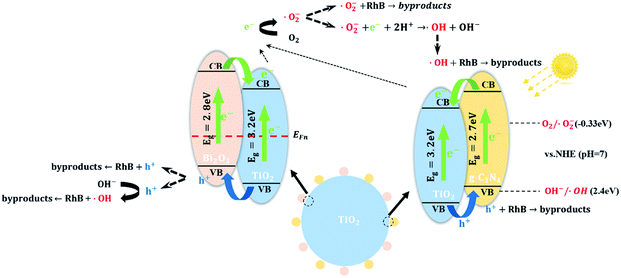 |
| Scheme 1 Photocatalytic degradation mechanism from the generation of photoinduced electron–hole to the decomposition of dyes. | |
On the one hand, the generation of g-C3N4 and Bi2O3 quantum dots with a narrow band gap facilitated the improvement of visible light harvesting ability, which motivates the generation of photoinduced carriers under visible light. On the other hand, the Fermi energy level of n-type semiconductors located nearer to the VB while the Fermi energy of p-type semiconductors located nearer to the VB.23,27,35 Therefore, after p-type Bi2O3 and n-type TiO2 were in contact, the electrons near the interface diffused from TiO2 to Bi2O3 while the holes diffused from Bi2O3 to TiO2. When the electrons migrated from TiO2, leaving a positively charged donor ion, and a positive charge region was formed on the side of the n region. In the same way, a negative charge region was formed on the side of p region. Therefore, there was no electrical neutrality on both sides of the interface of the p–n junction, and a positively and negatively charged region appeared, becoming a space charge region, which can force photoinduced electrons and holes to transfer in the opposite direction (Fig. 10b). The photogenerated electrons can only transfer from the CB of Bi2O3 to CB of TiO2, in contrast the holes can only transfer from the VB of TiO2 to the VB of Bi2O3, leading to a notably efficient separation of photoinduced pairs between Bi2O3 and TiO2. As for the heterojunction between g-C3N4 quantum dots and TiO2 particles, since the g-C3N4 has a more positive conduction band edge potential than that of TiO2 (−0.28 eV), the photoinduced electrons under visible irradiation tend to transfer from the CB of g-C3N4 to CB of TiO2.
Based on above calculation and analysis, an energy band structure has been proposed in Scheme 1. The photogenerated electrons and holes are unable to migrate from g-C3N4 to Bi2O3 through TiO2. Besides, the Bi2O3 and g-CN4 quantum dots don't interact and they are located in different position of TiO2 surface. Consequently, it's believed that there are just two heterojunctions (TiO2/g-C3N4, TiO2/Bi2O3) instead of a ternary heterojunction.
According to the previous researches, there are three types of radical degradation mechanism, including superoxide radical (·O2−), hydroxyl radical (·OH) and hole (h+).3,7,8,44,45 Steps of generation of the three radicals can be explained as follows:
H2O2 +·O−2 → ·OH + OH− + O2 |
In this work, there was a minor change in degradation performance after eliminating the holes through the scavenging test. Thus, it was concluded that the majority dye was degraded by superoxide radical (·O2−) or hydroxyl radical (·OH) induced by photo catalysis. Meanwhile, a slight number of dyes is degraded by hydroxyl radical (·OH) induced by photoinduced holes or directly oxidation by the holes.
Eventually, the systematic mechanism, including the generation and transfer of carriers, generation of radicals and reaction between radicals and dyes, was proposed in Scheme 1.
4 Conclusion
In summary, a facile ball-milling/calcination was successively utilized to prepared a Bi2O3 and g-C3N4 quantum dots modified anatase TiO2 heterojunction system. It was found that TCB-30% exhibited the highest removal rate for degradation of dyes under UV light irradiation and sunlight irradiation. Under sunlight irradiation, there was remarkable improvement of TCB-30%, the reaction rate constant was about 4.2 times and 3.3 times higher than that of TiO2 and g-C3N4 towards degradation of RhB. The notable enhancement of photocatalytic activity is mainly due to the ability of visible light harvesting and efficient separation of the carriers, which was induced by the modification of Bi2O3 and g-C3N4 quantum dots.
Conflicts of interest
There are no conflicts to declare.
Acknowledgements
This work was supported by National Key Research and Development Program (2018YFC1802605), Nature Science Foundation of Sichuan Province (2017GZ0383, 2017SZ0181), Science Foundation of China (31100374), the Key Research and Development Project of Sichuan Province (2018GZ0455), and the Sci-tech innovation seedling project from Sichuan Institute of Computer Science (18-YCG041).
References
- K. Nakata and A. Fujishima, TiO2 photocatalysis: design and applications, J. Photochem. Photobiol., C, 2012, 13(3), 169–189 CrossRef CAS.
- A. Fujishima and K. Honda, Electrochemical Photolysis of Water at a Semiconductor Electrode, Nature, 1972, 238(5358), 37–38 CrossRef CAS.
- H. Dong, G. Zeng, L. Tang, C. Fan, C. Zhang, X. He and Y. He, An overview on limitations of TiO2-based particles for photocatalytic degradation of organic pollutants and the corresponding countermeasures, Water Res., 2015, 79, 128–146 CrossRef CAS PubMed.
- V. Etacheri, C. Di Valentin, J. Schneider, D. Bahnemann and S. C. Pillai, Visible-light activation of TiO2 photocatalysts: advances in theory and experiments, J. Photochem. Photobiol., C, 2015, 25, 1–29 CrossRef CAS.
- A. Fujishima, X. Zhang and D. A. Tryk, TiO2 photocatalysis and related surface phenomena, Surf. Sci. Rep., 2008, 63(12), 515–582 CrossRef CAS.
- Y. Ren, Y. Dong, Y. Feng and J. Xu, Compositing Two-Dimensional Materials with TiO2 for Photocatalysis, Catalysts, 2018, 8(12), 590 CrossRef.
- J. Schneider, M. Matsuoka, M. Takeuchi, J. Zhang, Y. Horiuchi, M. Anpo and D. W. Bahnemann, Understanding TiO2 Photocatalysis: Mechanisms and Materials, Chem. Rev., 2014, 114(19), 9919–9986 CrossRef CAS PubMed.
- S. G. Kumar and L. G. Devi, Review on Modified TiO2 Photocatalysis under UV/Visible Light: Selected Results and Related Mechanisms on Interfacial Charge Carrier Transfer Dynamics, J. Phys. Chem. A, 2011, 115(46), 13211–13241 CrossRef CAS PubMed.
- S. C. Yan, Z. S. Li and Z. G. Zou, Photodegradation Performance of g-C3N4 Fabricated by Directly Heating Melamine, Langmuir, 2009, 25(17), 10397–10401 CrossRef CAS PubMed.
- J. G. Yu, S. H. Wang, J. X. Low and W. Xiao, Enhanced photocatalytic performance of direct Z-scheme g-C3N4-TiO2 photocatalysts for the decomposition of formaldehyde in air, Phys. Chem. Chem. Phys., 2013, 15(39), 16883–16890 RSC.
- J. Y. Lei, Y. Chen, F. Shen, L. Z. Wang, Y. D. Liu and J. L. Zhang, Surface modification of TiO2 with g-C3N4 for enhanced UV and visible photocatalytic activity, J. Alloys Compd., 2015, 631, 328–334 CrossRef CAS.
- C. Miranda, H. Mansilla, J. Yanez, S. Obregon and G. Colon, Improved photocatalytic activity of g-C3N4/TiO2 composites prepared by a simple impregnation method, J. Photochem. Photobiol., A, 2013, 253, 16–21 CrossRef CAS.
- H. Li, L. Zhou, L. Wang, Y. Liu, J. Lei and J. Zhang, In situ growth of TiO2 nanocrystals on g-C3N4 for enhanced photocatalytic performance, Phys. Chem. Chem. Phys., 2015, 17(26), 17406–17412 RSC.
- Y. M. Wu, L. Tao, J. Zhao, X. Yue, W. Y. Deng, Y. X. Li and C. Y. Wang, TiO2/g-C3N4 nanosheets hybrid photocatalyst with enhanced photocatalytic activity under visible light irradiation, Res. Chem. Intermed., 2016, 42(4), 3609–3624 CrossRef CAS.
- S. Bagwasi, Y. Niu, M. Nasir, B. Tian and J. Zhang, The study of visible light active bismuth modified nitrogen doped titanium dioxide photocatlysts: role of bismuth, Appl. Surf. Sci., 2013, 264, 139–147 CrossRef CAS.
- V. L. Chandraboss, J. Kamalakkannan and S. Senthilvelan, Synthesis of activated charcoal supported Bi-doped TiO2 nanocomposite under solar light irradiation for enhanced photocatalytic activity, Appl. Surf. Sci., 2016, 387, 944–956 CrossRef CAS.
- Y. Wang, Y. Wang, Y. Meng, H. Ding, Y. Shan, X. Zhao and X. Tang, A highly efficient visible-light-activated photocatalyst based on bismuth- and sulfur-codoped TiO2, J. Phys. Chem. C, 2008, 112(17), 6620–6626 CrossRef CAS.
- Y. Wu, G. Lu and S. Li, The Doping Effect of Bi on TiO2 for Photocatalytic Hydrogen Generation and Photodecolorization of Rhodamine B, J. Phys. Chem. C, 2009, 113(22), 9950–9955 CrossRef CAS.
- D. Y. Li, Y. G. Zhang, Y. L. Zhang, X. F. Zhou and S. J. Guo, Fabrication of bidirectionally doped beta-Bi2O3/TiO2-NTs with enhanced photocatalysis under visible light irradiation, J. Hazard. Mater., 2013, 258, 42–49 CrossRef PubMed.
- Y. Bessekhouad, D. Robert and J. V. Weber, Photocatalytic activity of CU2O/TiO2, Bi2O3/TiO2 and ZnMn2O4/TiO2 heterojunctions, Catal. Today, 2005, 101(3–4), 315–321 CrossRef CAS.
- Z. F. Bian, J. Zhu, S. H. Wang, Y. Cao, X. F. Qian and H. X. Li, Self-assembly of active Bi2O3/TiO2 visible photocatalyst with ordered mesoporous structure and highly crystallized anatase, J. Phys. Chem. C, 2008, 112(16), 6258–6262 CrossRef CAS.
- Y. D. Liu, F. Xin, F. M. Wang, S. X. Luo and X. H. Yin, Synthesis, characterization, and activities of visible light-driven Bi2O3-TiO2 composite photocatalysts, J. Alloys Compd., 2010, 498(2), 179–184 CrossRef CAS.
- J. Hou, C. Yang, Z. Wang, S. Jiao and H. Zhu, Bi2O3 quantum dots decorated anatase TiO2 nanocrystals with exposed {001} facets on graphene sheets for enhanced visible-light photocatalytic performance, Appl. Catal., B, 2013, 129, 333–341 CrossRef CAS.
- Y. Liu, F. Xin, F. Wang, S. Luo and X. Yin, Synthesis, characterization, and activities of visible light-driven Bi2O3-TiO2 composite photocatalysts, J. Alloys Compd., 2010, 498(2), 179–184 CrossRef CAS.
- S. Sood, S. K. Mehta, A. S. K. Sinha and S. K. Kansal, Bi2O3/TiO2 heterostructures: synthesis, characterization and their application in solar light mediated photocatalyzed degradation of an antibiotic, ofloxacin, Chem. Eng. J., 2016, 290, 45–52 CrossRef CAS.
- J. Xu, Y. Ao, D. Fu and C. Yuan, Synthesis of Bi2O3-TiO2 composite film with high-photocatalytic activity under sunlight irradiation, Appl. Surf. Sci., 2008, 255(5), 2365–2369 CrossRef CAS.
- Y. Zhang, J. Lu, M. R. Hoffmann, Q. Wang, Y. Cong, Q. Wang and H. Jin, Synthesis of g-C3N4/Bi2O3/TiO2 composite nanotubes: enhanced activity under visible light irradiation and improved photoelectrochemical activity, RSC Adv., 2015, 5(60), 48983–48991 RSC.
- Z. Zhao, J. Tian, D. Wang, X. Kang, Y. Sang, H. Liu, J. Wang, S. Chen, R. I. Boughton and H. Jiang, UV-visible-light-activated photocatalysts based on Bi2O3/Bi4Ti3O12/TiO2 double-heterostructured TiO2 nanobelts, J. Mater. Chem., 2012, 22(44), 23395–23403 RSC.
- J. Guo, J. Liang, X. Yuan, L. Jiang, G. Zeng, H. Yu and J. Zhang, Efficient Visible-Light Driven Photocatalyst, Silver (meta)vanadate: Synthesis, Morphology and Modification, Chem. Eng. J., 2018, 352, 782–802 CrossRef CAS.
- Mi-H. Jung and M. Gu Kang, Enhanced photo-conversion efficiency of CdSe–ZnS core–shell quantum dots with Au nanoparticles on TiO2 electrodes, J. Mater. Chem., 2011, 21, 2694 RSC.
- J. Hou, C. Yang, Z. Wang, S. Jiao and H. Zhu, Bi2O3 quantum dots decorated anatase TiO2 nanocrystals with exposed {001} facets on graphene sheets for enhanced visible-light photocatalytic performance, Appl. Catal., B, 2013, 129, 333–341 CrossRef CAS.
- G. Lia, Z. Liana, W. Wanga, D. Zhanga and H. Lia, Nanotube-confinement induced size-controllable g-C3N4 quantum dots modified single-crystalline TiO2 nanotube arrays for stable synergetic photoelectrocatalysis, Nano Energy, 2016, 19, 446–454 CrossRef.
- W.-K. Jo, T. Adinaveen, J. J. Vijaya and N. C. S. Selvam, Synthesis of MoS2 nanosheet supported Z-scheme TiO2/g-C3N4 photocatalysts for the enhanced photocatalytic degradation of organic water pollutants, RSC Adv., 2016, 6(13), 10487–10497 RSC.
- B. Naik, S. Martha and K. M. Parida, Facile fabrication of Bi2O3/TiO2-xNx nanocomposites for excellent visible light driven photocatalytic hydrogen evolution, Int. J. Hydrogen Energy, 2011, 36(4), 2794–2802 CrossRef CAS.
- M. SGe, C. Cao, S. Li, S. Zhang, S. Deng, J. Huang, Q. Li, K. Zhang, S. S. Al-Deyab and Y. Lai, Enhanced photocatalytic performances of n-TiO2 nanotubes by uniform creation of p–n heterojunctions with p-Bi2O3 quantum dots, Nanoscale, 2015, 7(27), 11552–11560 RSC.
- Z. Lu, L. Zeng, W. Song, Z. Qin, D. Zeng and C. Xie, In situ synthesis of C-TiO2/g-C3N4 heterojunction nanocomposite as highly visible light active photocatalyst originated from effective interfacial charge transfer, Appl. Catal., B, 2017, 202, 489–499 CrossRef CAS.
- G. Dong, Y. Zhang, Q. Pan and J. Qiu, A fantastic graphitic carbon nitride (g-C3N4) material: electronic structure, photocatalytic and photoelectronic properties, J. Photochem. Photobiol., C, 2014, 20, 33–50 CrossRef CAS.
- J. Fu, J. Yu, C. Jiang and B. Cheng, g-C3N4-Based Heterostructured Photocatalysts, Adv. Energy Mater., 2018, 8(3), 1701503 CrossRef.
- W.-J. Ong, 2D/2D Graphitic Carbon Nitride (g-C3N4) Heterojunction Nanocomposites for Photocatalysis: Why Does Face-to-Face Interface Matter?, Front. Mater. Sci., 2017, 4 DOI:10.3389/fmats.2017.00011.
- Y. Wu, L. Tao, J. Zhao, X. Yue, W. Deng, Y. Li and C. Wang, TiO2/g-C3N4 nanosheets hybrid photocatalyst with enhanced photocatalytic activity under visible light irradiation, Res. Chem. Intermed., 2016, 42(4), 3609–3624 CrossRef CAS.
- H. Yan and H. Yang, TiO2-g-C3N4 composite materials for photocatalytic H-2 evolution under visible light irradiation, J. Alloys Compd., 2011, 509(4), L26–L29 CrossRef CAS.
- N. Yang, G. Li, W. Wang, X. Yang and W. F. Zhang, Photophysical and enhanced daylight photocatalytic properties of N-doped TiO2/g-C3N4 composites, J. Phys. Chem. Solids, 2011, 72(11), 1319–1324 CrossRef CAS.
- K. Li, S. Gao, Q. Wang, H. Xu, Z. Wang, B. Huang, Y. Dai and J. Lu, In-Situ-Reduced Synthesis of Ti3+ Self-Doped TiO2/g-C3N4 Heterojunctions with High Photocatalytic Performance under LED Light Irradiation, ACS Appl. Mater. Interfaces, 2015, 7(17), 9023–9030 CrossRef CAS PubMed.
- M. A. M. Adnan, N. M. Julkapli, M. N. I. Amir and A. Maamor, Effect on different TiO2 photocatalyst supports on photodecolorization of synthetic dyes: a review, Int. J. Environ. Sci. Technol., 2019, 16(1), 547–566 CrossRef.
- P. Huo, P. Kumar and B. Liu, The Mechanism of Adsorption, Diffusion, and Photocatalytic Reaction of Organic Molecules on TiO2 Revealed by Means of On-Site Scanning Tunneling Microscopy Observations, Catalysts, 2018, 8(12), 616 CrossRef.
|
This journal is © The Royal Society of Chemistry 2020 |
Click here to see how this site uses Cookies. View our privacy policy here.