DOI:
10.1039/C9RA08721D
(Paper)
RSC Adv., 2020,
10, 9126-9132
Biomimetic enzyme barrier for preventing intestine-derived LPS induced diseases†
Received
24th October 2019
, Accepted 3rd February 2020
First published on 3rd March 2020
Abstract
Biomimetic enzyme barrier (BEB) encapsulated microcapsules with alginate shells were in situ fabricated with a microfluidic electrospray approach for preventing intestine-derived LPS induced diseases. As the alginate shells could protect the contents in gastric juice and release them in the intestine, the inner BEB could form a consecutive immune barrier on the surface of the intestine during the release. Through combining BEB with alkaline phosphatase, the immune barrier could degrade and prevent the permeation of lipopolysaccharide, which enhanced the intestinal barrier function. Thus, the BEB microcapsules were imparted with outstanding ability in preventing intestine-derived LPS induced diseases. Based on an in vivo study, we demonstrated that this BEB microcapsule could effectively protect organ function, restore intestinal barrier integrity, prevent the permeation of LPS and alleviate inflammation. Therefore, the generated microcapsules have potential for clinical applications.
Introduction
Most of the microbiota in the colon are tightly attached to the outer intestinal barrier, and the inner layer, which is composed of mucus, intestinal cells and tight junctions, forms a physical barrier that limits the permeation of pathogens.1–3 These 100 trillion microorganisms provide important functions which benefit the host. However, gut derived lipopolysaccharide (LPS), which is a component of bacteria in the gut, has been regarded as pathogenic or a lethal factor in many diseases, including sepsis, septic shock and metabolic syndrome (MS).4–7 To prevent the permeation of LPS, the gut barrier could secrete antimicrobial peptides, mucins and enzymes, which forms an important part of the immune barrier.8–10 However, the digestive system suffers from the modern high fat and high sugar (HFHS) diet, which seriously damaged this first line of defence and leads to LPS induced diseases.11–13 So far, nonbiological and nonpharmacological strategies have been evolved in this regard to protect and enhance the barrier function, including antibiotics, antibodies, dialysis, biomacromolecules and so on. Of particular concern is the use of alkaline phosphatase (ALP) as they play an important role in gut barrier's function which contribute to the degradation of excessive LPS.14–16 Although multiple researches of intravenous application of ALP in sepsis and septic shock has showed their value in preventing organ dysfunction, there is still no approach for enhancing barrier function and solving the pathogeny.
As an important part of apical brush border enzyme, ALP exerts its function through dephosphorylation of LPS, adenosine triphosphate etc., which maintained the homeostasis of intestine.17–19 The impaired expression of ALP in intestine lead to the dysfunction of gut barrier and several gastroenterology diseases. Orally delivered ALP could regulate the intestinal inflammation and permeability, which resulting in a protective effect against inflammatory diseases such as inflammatory bowel diseases.19–21 Hence, we believe a biomimetic enzyme barrier (BEB) based on ALP that could enhance the intestinal barrier function and clean the pathogens is ideal for gut-derived-LPS induced diseases. However, directly supplement of protein drugs lead to loss of deactivation of enzyme owing to complex environment in digestive tract. To overcome the encountered dilemma, microcapsules are fabricated with the form of particles or droplets which are surrounded by coatings in various fields. The microcapsulation process build a barrier between the contents and outer environment, which protects sensitive substances from the surroundings and controls the release of contents. With the application of microcapsule in oral delivery system, drug efficiency and biocompatibility could be improved.22–24
Thus, we herein employed electrostatically driven microfluidics with a biomimetic barrier system to encapsulate the ALP through core–shell microcapsule to imitate the mucus barrier with immune function on degrading LPS (Fig. 1). Core–shell microcapsules hold great potential for scenes involving drug delivery, encapsulation and functionalization without interfering contents' function.25–27 The alginate shell of the microcapsules formed through quick crosslinking with calcium ion (Ca2+), which could protect the contents from gastric juice and digestive enzymes. After degradation in the intestine, the encapsulated enzyme-mucosal adhesives were released to form a successive barrier on the surface of intestine, which could degrade LPS and protect the intestinal barrier. It was demonstrated that this BEB could reduce the permeation of LPS, relieving inflammation, restoring barrier function and prevent LPS induced diseases. Thus, this BEB microcapsule provides an ideal approach for treating gut-derived-LPS induced diseases.
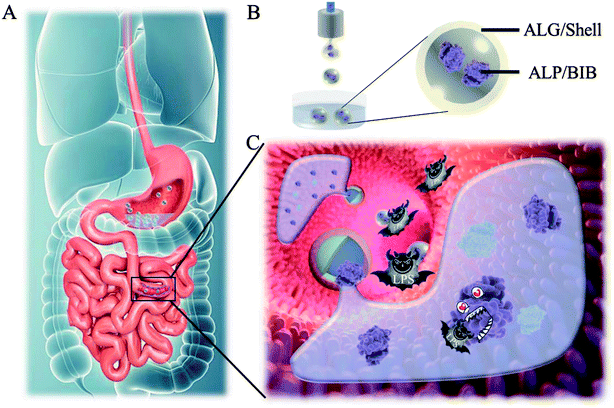 |
| Fig. 1 Design and fabrication of the BEB microcapsule. (A) Schematic illustration of the behaviour of microcapsule in digestive tract. (B) The fabrication process of microcapsule. (C) Forming BEB in intestine and degrading LPS. | |
Results and discussion
Characterizing the BEB microcapsule
In a typical experiment, core–shell microcapsules were fabricated with a coaxial capillary microfluidic chip to form a biomimetic enzyme barrier with the encapsulated contents through electrospray. The coaxial chip was prepared with an inner capillary and an outer capillary with centres aligned. During the process of electrospray, the ALP-carboxymethyl cellulose sodium (CMC)-sucralfate solution flowed inside the inner capillary and the outer phase of ALP flowed along the outer capillary. Due to the low Reynolds numbers, laminar flows were formed with inner and outer phase at the merging point. Within the electric field, the coflowing broke into droplets and gelled with calcium chloride (CaCl2) in the gelling bath. The fast gelation of alginate through crosslinking with Ca2+ solidified the shell structure of the microcapsules. Within this process, the inner ALP-CMC-sucralfate was encapsulated in alginate shells with a core–shell structure. Finally, the generated microcapsules were collected from the gelling bath for further characterization (Fig. 2). Through regulating voltage (U), collecting distance (d), flow rate of outer phase (Fouter) and inner phase (Finner), the size of the microcapsules could be precisely controlled (Fig. S1†). The dissected microcapsule showed different surficial and cross-sectional structure in details under SEM which showed a smooth surface of alginate shell and a rather tough morphology of the inner section (Fig. 2D and E) indicating the seperation between core and shell. This result also implied the successful generation of core–shell microcapsules.
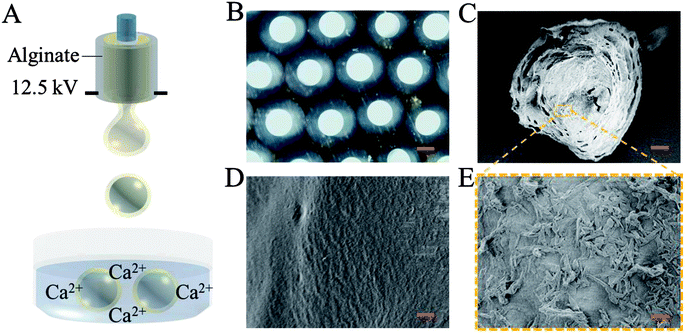 |
| Fig. 2 Fabrication of the microcapsule. (A) The fabrication of microfluidic electrospray process. (B) Bright field microscopic images of BEB microcapsules. Scale bar is 100 μm. (C) Image of dissected microcapsule under SEM. Scale bar is 50 μm. (D) The SEM image of the outer surface of the microcapsule. Scale bar is 1 μm. (E) The SEM image of the inner surface of the microcapsule. Scale bar is 1 μm. | |
The damage of gut barrier integrity has been regarded as a fundamental factor in the pathogenesis of many gut-derived diseases, such as inflammatory bowel diseases and MS.28 Researches have demonstrated the importance of a consecutive and functional barrier in maintaining intestinal homeostasis and preventing diseases.29–31 Thus, a BEB that could spread on the intestine with functional properties against pathogens could enhance the barrier function and contribute to many diseases. This microcapsule could restrain the efflux of contents in gastric environment (pH 1.5) and release contents in pH 7.4 (Fig. S2†). We believe this ability contributed to the well-regulated drug release in digestive tract. To investigate the effect of microcapsules, in vivo CT imaging and IVIS were utilized to analyse the distribution and continuity of the BEB in intestine (Fig. 3). Before gavage, there was no signal in IVIS and CT, which indicated no disperse of contents. After 12 h of gavage, the stomach, duodenum and lower gastrointestinal tract showed significant signal in IVIS. The continuous signal in IVIS showed successful formation of the BEB in vivo, which could establish a relatively complete artificial barrier to enhance the natural barrier's function. CT showed the distribution of BEB on sections of digestive tract, which implied the well-distributed spread of BEB. These results demonstrated the effect of microcapsules in building BEB, which could degrade LPS and compensate the immune barrier function, and indicated the well generating process of BEB. Owing to the complex environment in intestine and the microcapsule took time to release the contents, the upper intestine was not fully covered but we think the resultant BEB was enough for therapy.
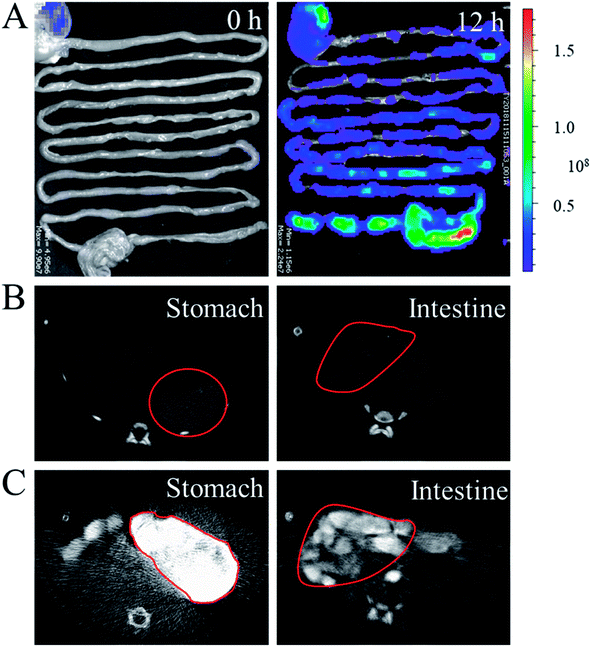 |
| Fig. 3 Characterization of the formation of BEB in vivo. (A) In vivo imaging system (IVIS) images of BEB at 0 and 12 h. (B) Computed tomography (CT) images of rats before gavage with microcapsules. (C) CT images of rats after gavage with microcapsules for 12 h. The positions of stomach and intestine were pointed out with red marker. | |
The therapeutic effect of BEB
To investigate the biomedical value of the BEB microcapsule, their effect in degrading LPS and preventing LPS-induced damage was evaluated through cell viability. PBS, LPS, LPS + microcapsule and LPS + BEB microcapsule were coculture for 1 h. The resultants were added to Caco-2 cells respectively and cocultured for another 24 h. The cells in LPS treated groups showed significantly decrease after coculture. The microcapsule + LPS group showed no difference with the LPS treated group which indicated no effect of the carrier. In comparison with other groups, BEB microcapsule group could decrease LPS-induced cell death (Fig. 4). The Caco-2 cells died massively in contact with LPS while the presence of BEB could reverse the condition. On one side, the result indicated the effect of BEB on degrading LPS and prevent LPS induced damage. On the other side, it also implied the beneficial aspect of the BEB microcapsule in dealing with LPS induced diseases.
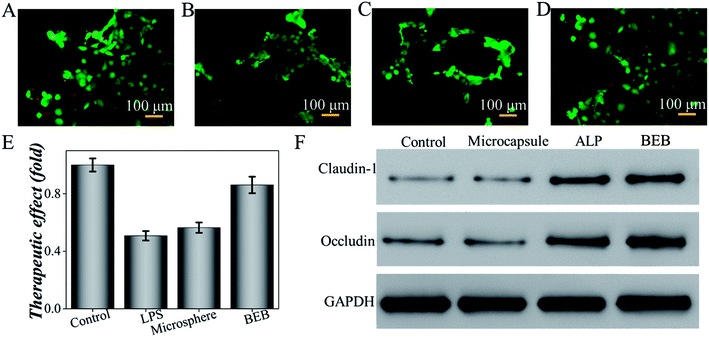 |
| Fig. 4 The detoxication tests of microcapsules with different interventions. (A–D) The confocal laser scanning images of the Live/Dead staining of Caco-2 cells treated with (A) PBS, (B) LPS, (C) microcapsule + LPS and (D) BEB microcapsule + LPS. (E) The statistical graph of cell viability in different groups. (F) The expression of tight junction proteins in intestine of different groups. | |
Through translocation of gut-derived LPS, a series of disorders could be induced.8,32,33 Restoring barrier integrity and reducing the absorption of LPS could improve these disorders.34,35 Thus, a mice model based on HFHS induced MS was finally conducted to investigate the practical value of the BEB in LPS-induced diseases. The mice were divided into four groups including control group, microcapsule treated group, ALP treated group and BEB microcapsule treated group. The capability in restoring gut barrier function and therapeutic effect on organs were evaluated through the expression level of tight junctions and fat deposition in liver. WB showed the expression of claudin-1 and occludin in intestine after interventions (Fig. 4F). Both of ALP and BEB could increase the expression of tight junctions but BEB could greatly improve the distribution of these proteins in apical cells and exhibited more potential in restoring the barrier function (Fig. 5). We think the damage of gut barrier is the initial factor of MetS. Through restoring barrier function, the MetS could, thus, be alleviated. Additionally, BEB showed less fat disposition than other groups. These results indicated the therapeutic effect of the BEB microcapsule in preventing MS. To investigate the underlying mechanism, organs were fetched out for analysing the permeation of LPS and inflammation of organs (Fig. S3 and S4†). The concentrations of LPS of organs in different groups were detected. There were differences in the levels of LPS in intestine which indicated that the permeation of LPS could be reduced through the application of BEB. The average levels of LPS in spleen of BEB treated groups were also lower than HFHS induced control group (Fig. S3†). This result indicated the transfusion of LPS into blood, which bring burden to organs. Spleen showed stress towards the change, while BEB could reduce the stress. Thus, the intestines and spleens were further analysed with inflammatory levels of IL-1β, TNF-α and IL-6 (Fig. S4†). These inflammatory factors all decreased in BEB treated group. Hence, we expected that the permeation of excessive LPS lead to high level of inflammation of intestine and spleen, which result in fat deposition in liver later. Additionally, through utilization of BEB could reduce the permeation of LPS and restore the barrier function, which contribute to alleviating the LPS induced diseases.
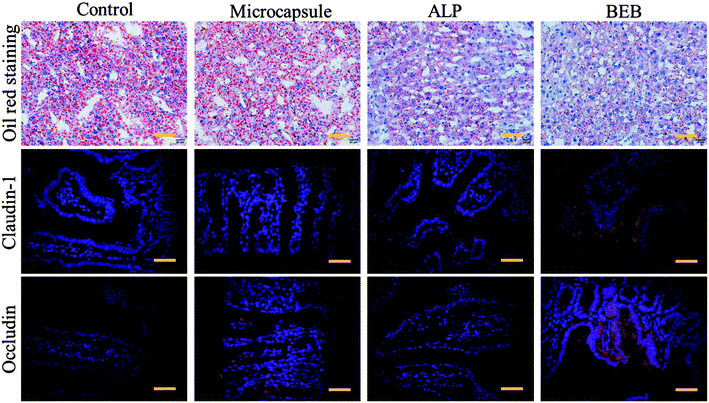 |
| Fig. 5 Therapeutic effect of the BEB microcapsule on MS, including liver oil red O staining and the immunofluorescence staining of distribution of tight junction proteins in different groups. Scale bars are 50 μm. | |
Conclusion
In conclusion, we have developed BEB microcapsules for preventing gut-derived-LPS induced diseases. Owing to the core–shell structure and muco-adhesive solution, the resultant microcapsule could release the BEB along the intestine and form a consecutive barrier with capability in degrading LPS. This microcapsule could protect the cells from LPS induced damage and show biomedical potential in restoring barrier function. The practical value of BEB existed in preventing the permeation of LPS and, thus, alleviating the inflammation of organs. Improved inflammation level relieved organ function in return. These features manifested that this BEB microcapsule were efficient for gut-derived-LPS diseases.
Experimental section
Materials, cell lines, and animals
Alginate, CaCl2, sucralfate, CMC, ALP, cy5, BSA-FITC, calcein-AM/PI (CA-PI) and phosphate buffer saline (PBS) were bought from Aladdin. A high voltage power supply was purchased from Dong Wen High Voltage. Diodone was purchased from Jinkelong Biotech Company. Caco-2 cells, the 8–12 week male mice and 200 ± 10 g rats were from Jinling Hospital. All animals were treated in strict accordance with the recommendations in the Guide for the Care and Use of Laboratory Animals of the National Institutes of Health, USA. All animals' experimental protocols and care were reviewed and approved by Animal Investigation Ethics Committee of the Jinling Hospital.
Experimental design
Fabrication of microfluidic device. A glass slide and capillaries were used to fabricate the microfluidic device. The outer capillary was sanded to reach a diameter of 350 μm, and the inner capillary was pulled after heated to form spindle tips with a diameter of 150 μm. The outer capillary and the inner capillary were then coaxially assembled. Needles were used to connect the inner and outer capillary. Transparent epoxy resin was used to fix the device.
Fabrication of the microcapsules. 4% alginate was used for outer phase and CMC-ALP-sucralfate was used for inner phase. A voltage power supply was used to generate electric field for electrospray. The coflowing formed laminar flows stretched to Taylor cone entirely within the electric field. The flows broke into droplets and gelled in CaCl2. The microcapsules were generated and collected for characterization. The variables were regulated to analyse the size of microcapsules.
Characterization. Bright-field images of microcapsules were recorded by microscopy (OLYMPUS IX71) equipped with CCD camera. The microstructures were characterized by a scanning electron microscope (SEM). Before SEM, microcapsules were dehydrated and fixed with a double-sided adhesive tape. The samples were then covered with gold with SEM-ion sputter. After fabrication of BEB microcapsule, we utilized CT imaging and IVIS to visualize the distribution of the BEB in intestine. In CT imaging, the inner phase was composed with diodone and encapsulated in microcapsules which were used for gavage later. In IVIS, cy5 was composed with inner phase for microcapsule. Data were collected before gavage and 12 h after gavage. Images were normalized with the control group. To study the drug release ability of the core–shell microcapsules, BSA-FITC were encapsulated and cultured in HCl buffer at pH 1.5 and in phosphate buffer at pH 7.4. The intensity of fluorescence in solvents were collected and analysed then.
Detoxication effect of the microcapsules. Before the experiment, 0.1 μg mL−1 LPS was cocultured with PBS, microcapsule and BEB microcapsule for 1 h. Then, the resultants were extracted and cocultured with 104 Caco-2 cells. Normal cells without interventions were set as control group. After 24 h, cells in control group, LPS group, microcapsule + LPS group and BEB microcapsule + LPS group were stained with CA-PI to analyse the cell viability. The images were recorded with a fluorescent microscope.
Protection of microcapsules on mice. HFHS diet induced MS model was set to evaluate the effect of BEB microcapsules on preventing LPS-induced diseases. 20 C57/BL mice were fed a HFHS diet for 8 weeks and divided into four groups, including PBS treated group (control group), microcapsule group (alginate-CMC), ALP group and BEB microcapsule group (alginate-CMC-ALP-sucralfate). All mice were sacrificed for collecting intestine, liver, heart, spleen, lung and kidney at 8th week. The organs were further analysed with ELISA for the levels of LPS and inflammatory factors including IL-1 β, TNF-α and IL-6 following the manufacturer's instructions. The resultants were measured according to standard curves. Results were expressed with respect to control.
Oil red O staining and immunofluorescence analysis. Before the experiment, tissues were immersed in 4% neutral formaldehyde for 24 h. Frozen liver tissues were stained with oil red analyse the fat deposition. Intestines were dehydrated and embedded in paraffin. Sections was conducted with a microtome. To conduct the immunofluorescence staining, the sections were incubated with ZO-1 or occludin antibody at 4 °C for 12 h. Afterwards, the sections were washed and the secondary antibody (1% BSA) was further applied at 25 °C for 1 h. Images were recorded with a fluorescence microscope.
Author contributions
J. A. R. and X. W. W. conceived the study and participated in its design. C. Z. and X. X. W. participated in the fabrication of the microcapsules. C. Z., Q. J. L., Q. Y. H. and J. W. finished the remaining experiments, drew all figures in this article and wrote this manuscript. Z. W. W. and X. M. K. helped write the paper. All authors read and approved the final manuscript.
Ethics approval and consent to participate
Animal experiments were performed in accordance with the Guide for the Care and Use of Laboratory Animals of the Institute for Laboratory Animal Research, and approval to conduct the study was obtained from the Animal Investigation Ethics Committee of Jinling Hospital. The methods were carried out according to the approved guidelines.
Conflicts of interest
The authors declare no competing financial interests.
Acknowledgements
This work was supported by National Major Scientific and Technological Special Project for “Significant New Drugs Development” (2018ZX09J18111-04), the grants from Projects of Jiangsu Social Development (BE2017722), General Project of Military Logistics Research (CLB19J025), Distinguished Scholars Foundation of Jiangsu Province (JCRCB2016006), Key Project of Science Foundation of the 12th Five-Year Plan (BNJ13J002) and The China Postdoctoral Science Foundation Grant (2019M663108).
References
- K. Dabke, G. Hendrick and S. Devkota, The gut microbiome and metabolic syndrome, J. Clin. Invest., 2019, 129, 4050–4057, DOI:10.1172/jci129194.
- Y. Goto, Epithelial Cells as a Transmitter of Signals From Commensal Bacteria and Host Immune Cells, Front. Immunol., 2019, 10, 2057, DOI:10.3389/fimmu.2019.02057.
- A. Nicoletti, et al., Intestinal permeability in the pathogenesis of liver damage: from non-alcoholic fatty liver disease to liver transplantation, World J. Gastroenterol., 2019, 25, 4814–4834, DOI:10.3748/wjg.v25.i33.4814.
- L. G. Hersoug, P. Moller and S. Loft, Gut microbiota-derived lipopolysaccharide uptake and trafficking to adipose tissue: implications for inflammation and obesity, Obes. Rev., 2016, 17, 297–312, DOI:10.1111/obr.12370.
- L. G. Hersoug, P. Moller and S. Loft, Role of microbiota-derived lipopolysaccharide in adipose tissue inflammation, adipocyte size and pyroptosis during obesity, Nutr. Res. Rev., 2018, 31, 153–163, DOI:10.1017/s0954422417000269.
- Y. Ji, Y. Yin, Z. Li and W. Zhang, Gut Microbiota-Derived Components and Metabolites in the Progression of Non-Alcoholic Fatty Liver Disease (NAFLD), Nutrients, 2019, 11(8) DOI:10.3390/nu11081712.
- Z. Wang and Y. Zhao, Gut microbiota derived metabolites in cardiovascular health and disease, Protein Cell, 2018, 9, 416–431, DOI:10.1007/s13238-018-0549-0.
- M. Guerville and G. Boudry, Gastrointestinal and hepatic mechanisms limiting entry and dissemination of lipopolysaccharide into the systemic circulation, Am. J. Physiol.: Gastrointest. Liver Physiol., 2016, 311, G1–G15, DOI:10.1152/ajpgi.00098.2016.
- A. L. Neves, J. Coelho, L. Couto, A. Leite-Moreira and R. Roncon-Albuquerque Jr, Metabolic endotoxemia: a molecular link between obesity and cardiovascular risk, J. Mol. Endocrinol., 2013, 51, R51–R64, DOI:10.1530/jme-13-0079.
- R. Wiest, M. Lawson and M. Geuking, Pathological bacterial translocation in liver cirrhosis, J. Hepatol., 2014, 60, 197–209, DOI:10.1016/j.jhep.2013.07.044.
- S. S. Ghosh, et al., Dietary Supplementation with Galactooligosaccharides Attenuates High-Fat, High-Cholesterol Diet-Induced Glucose Intolerance and Disruption of Colonic Mucin Layer in C57BL/6 Mice and Reduces Atherosclerosis in Ldlr-/- Mice, J. Nutr., 2020, 150(2), 285–293, DOI:10.1093/jn/nxz233.
- M. Hussain, et al., Meat proteins in a high-fat diet have a substantial impact on intestinal barriers through mucus layer and tight junction protein suppression in C57BL/6J mice, Food Funct., 2019, 10, 6903–6914, 10.1039/c9fo01760g.
- H. Xu, et al., The ameliorative effect of the Pyracantha fortuneana (Maxim.) H. L. Li extract on intestinal barrier dysfunction through modulating glycolipid digestion and gut microbiota in high fat diet-fed rats, Food Funct., 2019, 10(10), 6517–6532, 10.1039/c9fo01599j.
- J. Bilski, et al., The Role of Intestinal Alkaline Phosphatase in Inflammatory Disorders of Gastrointestinal Tract, Mediat. Inflamm., 2017, 2017, 9074601, DOI:10.1155/2017/9074601.
- F. Hummeke-Oppers, P. Hemelaar and P. Pickkers, Innovative Drugs to Target Renal Inflammation in Sepsis: Alkaline Phosphatase, Front. Pharmacol., 2019, 10, 919, DOI:10.3389/fphar.2019.00919.
- B. A. Rader, Alkaline Phosphatase, an Unconventional Immune Protein, Front. Immunol., 2017, 8, 897, DOI:10.3389/fimmu.2017.00897.
- K. Geddes and D. J. Philpott, A new role for intestinal alkaline phosphatase in gut barrier maintenance, Gastroenterology, 2008, 135, 8–12, DOI:10.1053/j.gastro.2008.06.006.
- J. P. Lalles, Intestinal alkaline phosphatase: novel functions and protective effects, Nutr. Rev., 2014, 72, 82–94, DOI:10.1111/nure.12082.
- W. M. Miner-Williams and P. J. Moughan, Intestinal barrier dysfunction: implications for chronic inflammatory conditions of the bowel, Nutr. Res. Rev., 2016, 29, 40–59, DOI:10.1017/s0954422416000019.
- M. Estaki, D. DeCoffe and D. L. Gibson, Interplay between intestinal alkaline phosphatase, diet, gut microbes and immunity, World J. Gastroenterol., 2014, 20, 15650–15656, DOI:10.3748/wjg.v20.i42.15650.
- S. Mozaffari, S. Nikfar and M. Abdollahi, Inflammatory bowel disease therapies discontinued
between 2009 and 2014, Expet Opin. Invest. Drugs, 2015, 24, 949–956, DOI:10.1517/13543784.2015.1035432.
- L. J. De Cock, et al., Polymeric Multilayer Capsules in Drug Delivery, Angew. Chem., Int. Ed., 2010, 49, 6954–6973, DOI:10.1002/anie.200906266.
- C. Zhang, X. Li, Y.-N. Liu and F. Zhang, Utilization of Microcapsule Technology in Foods, J. Nanosci. Nanotechnol., 2015, 15, 9330–9340, DOI:10.1166/jnn.2015.9226.
- C. Zhao, et al., Alginate and Probiotics Synergistically Reversed Dextran Sulfate Sodium Salt (DSS)-Induced Gut Barrier Damage, Macromol. Res., 2019, 27, 888–894, DOI:10.1007/s13233-019-7122-4.
- Y. Liu, X. Zhao, C. Zhao, H. Zhang and Y. Zhao, Responsive Porous Microcarriers With Controllable Oxygen Delivery for Wound Healing, Small, 2019, 15(21), e1901254, DOI:10.1002/smll.201901254.
- D. Wu, et al., NK-Cell-Encapsulated Porous Microspheres via Microfluidic Electrospray for Tumor Immunotherapy, ACS Appl. Mater. Interfaces, 2019, 11, 33716–33724, DOI:10.1021/acsami.9b12816.
- C. Zhao, et al., Biomimetic intestinal barrier based on microfluidic encapsulated sucralfate microcapsules, Sci. Bull., 2019, 64, 1418–1425, DOI:10.1016/j.scib.2019.07.020.
- A. J. Forgie, J. M. Fouhse and B. P. Willing, Diet-Microbe-Host Interactions That Affect Gut Mucosal Integrity and Infection Resistance, Front. Immunol., 2019, 10, 1802, DOI:10.3389/fimmu.2019.01802.
- M. Antonini, M. Lo Conte, C. Sorini and M. Falcone, How the Interplay Between the Commensal Microbiota, Gut Barrier Integrity, and Mucosal Immunity Regulates Brain Autoimmunity, Front. Immunol., 2019, 10, 1937, DOI:10.3389/fimmu.2019.01937.
- S. Iacob and D. G. Iacob, Infectious Threats, the Intestinal Barrier, and Its Trojan Horse: Dysbiosis, Front. Microbiol., 2019, 10, 1676, DOI:10.3389/fmicb.2019.01676.
- C. Wang, Q. Li and J. Ren, Microbiota-Immune Interaction in the Pathogenesis of Gut-Derived Infection, Front. Immunol., 2019, 10, 1873, DOI:10.3389/fimmu.2019.01873.
- N. Enomoto, et al., Burn injury sensitizes rat Kupffer cells via mechanisms dependent on gut-derived endotoxin, J. Gastroenterol., 2004, 39, 1175–1181, DOI:10.1007/s00535-004-1468-9.
- F. Laugerette, et al., Oil composition of high-fat diet affects metabolic inflammation differently in connection with endotoxin receptors in mice, Am. J. Physiol.: Endocrinol. Metab., 2012, 302, E374–E386, DOI:10.1152/ajpendo.00314.2011.
- P. D. Cani and N. M. Delzenne, The gut microbiome as therapeutic target, Pharmacol. Therapeut., 2011, 130, 202–212, DOI:10.1016/j.pharmthera.2011.01.012.
- G. Reid, et al., Microbiota restoration: natural and supplemented recovery of human microbial communities, Nat. Rev. Microbiol., 2011, 9, 27–38, DOI:10.1038/nrmicro2473.
Footnote |
† Electronic supplementary information (ESI) available. See DOI: 10.1039/c9ra08721d |
|
This journal is © The Royal Society of Chemistry 2020 |
Click here to see how this site uses Cookies. View our privacy policy here.