DOI:
10.1039/C9RA09328A
(Paper)
RSC Adv., 2020,
10, 6822-6830
The influence of hydrogen concentration in amorphous carbon films on mechanical properties and fluorine penetration: a density functional theory and ab initio molecular dynamics study†
Received
10th November 2019
, Accepted 30th January 2020
First published on 13th February 2020
Abstract
Amorphous carbon (a-C) films have attracted significant attention due to their reliable structures and superior mechanical, chemical and electronic properties, making them a strong candidate as an etch hard mask material for the fabrication of future integrated semiconductor devices. Density functional theory (DFT) calculations and ab initio molecular dynamics (AIMD) simulations were performed to investigate the energetics, structure, and mechanical properties of the a-C films with an increasing sp3 content by adjusting the atomic density or hydrogen content. A drastic increase in the bulk modulus is observed by increasing the atomic density of the a-C films, which suggests that it would be difficult for the films hardened by high atomic density to relieve the stress of the individual layers within the overall stack in integrated semiconductor devices. However, the addition of hydrogen into the a-C films has little effect on increasing the bulk modulus even though the sp3 content increases. For the F blocking nature, the change in the sp3 content by both atomic density and H concentration makes the diffusion barrier against the F atom even higher and suppresses the F diffusion, indicating that the F atom would follow the diffusion path passing through the sp2 carbon and not the sp3 carbon due to the significantly high barrier. For the material design of a-C films with adequate doped characteristics, our results can provide a new straightforward strategy to tailor the a-C films with excellent mechanical and other novel physical and chemical properties.
1. Introduction
Amorphous carbon (a-C) films have attracted significant attention due to their reliable structures and superior mechanical, chemical and electronic properties,1–3 making them a strong candidate as an etch hard mask material for the fabrication of future integrated semiconductor devices. However, for wider applications, it is still necessary to overcome the current limitations such as high internal stresses, low blocking nature of fluorine attack to sub-layers, and low temperature stability.4 In order to eliminate the aforementioned drawbacks and enhance the properties of the a-C films, the influence of hydrogen to modify their structure during the deposition process was studied.
The a-C films are mainly deposited on large areas at relatively low temperatures by plasma-enhanced chemical vapor deposition (PECVD) using hydro-carbon mixed precursor gases such as CH4 or C2H2 diluted in H2.5,6 However, it is well known that the F atoms, which originate from the injection of etchant gases such as CFx during the device fabrication process, generally attack the sub-layers and significantly degrade the device performance due to the extremely high reactive nature of F atoms with other atoms that have an electron-donating nature such as B, Al, and Ga; this results in the bond breaking of sub-layer materials to form F-containing alloys. This problem is a primary obstacle in the development of future integrated semiconductor devices.7,8 To address this problem, a theoretical comprehension of the a-C films is required for the device fabrication process due to the experimentally limited observations on the atomistic scale.
The properties of the a-C films depend on the sp3-to-sp2 hybridization ratio and the H content.9,10 Depending on the composition, these films exhibit different characteristics such as hardness, electrical resistivity, optical transparency, and chemical inertness. The a-C films with high hardness, high sp2 content, and low optical band gaps are referred to as hard graphitic a-C.11 On the other hand, the a-C films with hardness and resistivity similar to that of a diamond are referred to as diamond-like carbon (DLC).12
Briefly, in previous studies, Mikami et al.13 found that an increase in the H2 flow rate leads to an increase in the sp3 fraction and disorder during the deposition of the DLC films by radiofrequency (RF) magnetron sputtering. Vietzke et al.14 investigated the hydrogen-induced chemical erosion of C atoms from the a-C films using mass spectrometry. They reported the formation of CH3 radicals along with a wide range of high hydrocarbons due to the reaction of the thermal H atoms within the a-C films. Other researchers reported that the growth rate, film structure, mechanical properties, optical gaps and field emission were all considerably affected by hydrogen.15,16
Although several experimental studies on the influence of the hydrogen content in an a-C film have been investigated, no guidelines exist for improving the a-C film as an etch hard mask because the relationship between the structure of the a-C film and its etching characteristics remains unclear. Ab initio calculations provide a powerful tool to determine the atomic and electronic details and provide a deeper insight into the stress reduction mechanism of the a-C films.17–19
In this paper, we examine the energetics, structure, and mechanical properties of a-C films with increasing sp3 content by adjusting the atomic density or hydrogen content using density functional theory (DFT) and ab initio molecular dynamics (AIMD) calculations. In our simulation, we generated amorphous carbon structures containing 64 C atoms to focus on studying the influence of the increase in the sp3 content by adjusting the atomic density or hydrogen content on the mechanical properties during the device fabrication processes.
We also theoretically investigated the influence of the increase in the sp3 content by adjusting the atomic density or hydrogen content on the diffusion of F atoms during the dry etch process, generating important findings in the field of materials science.
2. Computational methods
2.1. Generation of the model structure
Ab initio molecular dynamics (AIMD) simulations were carried out to obtain amorphous carbon structures. The cubic supercell of 64 atoms with a fixed lattice constant of 7.61 Å was used, which corresponded to the density of the reference amorphous carbon with 2.9 g cm−3.
The melt-quench simulations20,21 were performed by pre-melting for 2 ps at 12
000 K, melting for 10 ps at 5000 K, and quenching to 0 K with a constant cooling rate of −250 K ps−1. In the designed doped amorphous structures, the densities of dense a-C structures were selected as 3.2, 3.5, and 4.0 g cm−3, which corresponded to the lattice constants of 7.36, 7.14, and 6.83 Å. Hydrogen concentrations in H-added a-C structures were selected as 15.6, 23.4, and 31.2 at%, corresponding to 10, 15, and 20 atoms in 64-atom models. The final amorphous carbon structure was obtained by full relaxation of the atomic positions. Finally, the amorphous carbon structure comprised a tetra-coordinated C fraction of 56.3% with a radius of 1.8 Å to define the distances between the nearest neighbours for all amorphous carbon structures. This cut-off radius was obtained by calculating the first minimum value in the radial distribution function (RDF).22
2.2. DFT calculations
In our theoretical results, all DFT calculations were performed using the Vienna Ab initio Simulation Package (VASP) program with the Perdew–Burke–Ernzerhof (PBE) functional in the generalized gradient approximation (GGA).23,24 We used the PBE-D2 functional25 based on the projector augmented wave (PAW) method26 with a conventional Kohn–Sham DFT energy correction to treat the van der Waals interactions for all DFT calculations (see the ESI†27).
Valence orbitals were described by a plane-wave basis set with the cut-off energy of 400 eV. Ultrasoft Vanderbilt-type pseudopotentials28 were used to describe the interactions between ions and electrons. The Brillouin zone for all amorphous structures was sampled with a 3 × 3 × 3 Monkhorst–Pack k-point mesh. Geometry optimization was performed by minimizing the forces of all atoms to less than 0.02 eV Å−1 with the total energy of the system converged within 10−4 eV during self-consistent iterations. We determined the energy barriers (activation energies) through the climbing-image nudged elastic band (CINEB) method29 using a force tolerance of 0.02 eV Å−1. For an accurate calculation of the barriers, the CINEB method with a more precise transition-state search algorithm than the NEB method was used.30,31 This method was made to search one of the various states near the transition-state along the reaction path, converging at the highest saddle point.32–35
3. Results and discussion
3.1. Influence of atomic density and hydrogen concentration on mechanical properties
Fig. 1 shows the final atomic structures of 64 C atom-containing a-C films with an increase in the atomic density (3.2 to 4.0 g cm−3) and hydrogen concentrations (15.6 to 31.2 at%). The dependence of the calculated bulk modulus on the atomic density is illustrated in Fig. 2(a). In the case of the reference a-C film (Ref. a-C), a bulk modulus of about 212 GPa was estimated. With the increase in atomic density, the bulk modulus drastically increased; when the atomic density was 4.0 g cm−3, a maximal bulk modulus of about 617 GPa was obtained, which was around three-fold larger than that of the reference a-C film. On the other hand, on introducing H atoms in the a-C films, as shown in Fig. 2(b), the bulk modulus as a function of the H concentration increased and then slightly increased. When the H concentration was 31.2 at%, a maximal bulk modulus of about 248 GPa was obtained, which slightly increased by 17% compared to that for the reference a-C film.
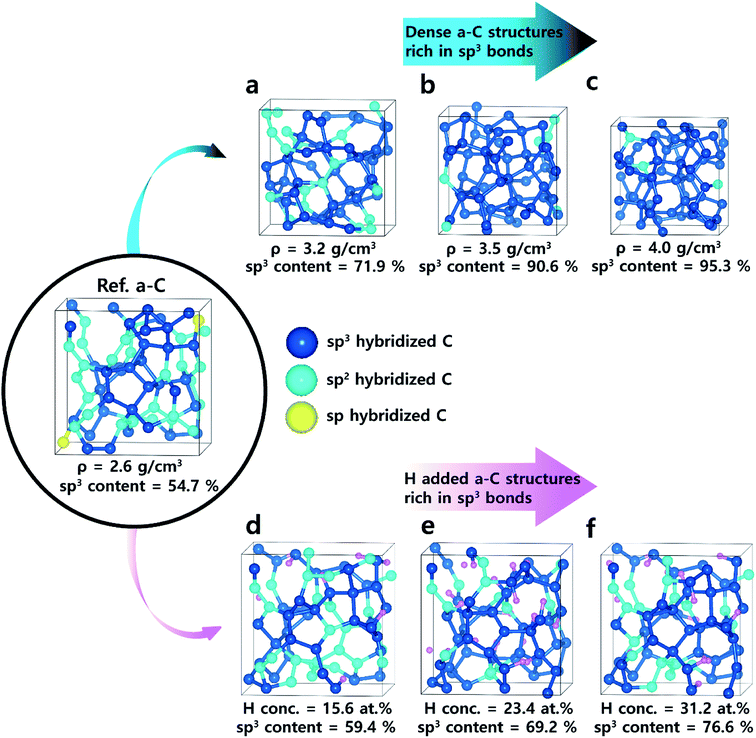 |
| Fig. 1 Optimized atomic structures of 64 C atom-containing amorphous carbon structures with increasing atomic density (3.2 to 4.0 g cm−3) and hydrogen concentrations (15.6 to 31.2 at%). Blue, sky-blue, yellow, and pink colors indicate sp3, sp2, sp hybridized C and H atoms, respectively. | |
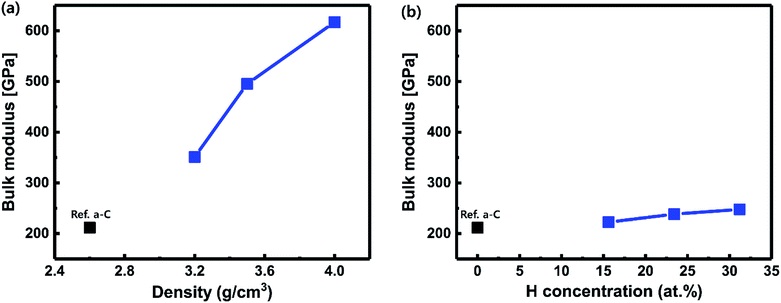 |
| Fig. 2 Dependency of the calculated bulk modulus on (a) atomic density from 3.2 to 4.0 g cm−3 and (b) hydrogen concentrations from 15.6 to 31.2 at%, corresponding to 10 H, 15 H, and 20 H-added a-C. | |
I. N. Remediakis et al.,36 revealed that the bulk modulus of a-C films was enhanced as the film density increased (335 GPa at 2.7 g cm−3, 375 GPa at 3.05 g cm−3, and 465 GPa at 3.55 g cm−3). This experimental result showed a similar trend with our DFT results (212 GPa at 2.6 g cm−3, 351 GPa at 3.2 g cm−3, and 450 GPa at 3.5 g cm−3); however, there is some discrepancy since the amorphous phase is characterized by a short-range order and the atoms are bonded in randomly disordered positions due to a phenomenon that does not allow the formation of a regular arrangement.
Nevertheless, taking advantage of the benefits of the simulation, it is noted that the drastic increase in the bulk modulus is observed by increasing the atomic density of the a-C films, which suggests that it would be difficult for the films hardened by high atomic density to relieve the stress of the individual layers within the overall stack in integrated semiconductor devices. However, the addition of hydrogen in the a-C films has little effect on increasing the bulk modulus even though the sp3 content increases. These findings suggest that the process design for high-sp3-content a-C films should be carried out by adding hydrogen rather than increasing the atomic density. These requirements become more important and increasingly challenging to meet as the device integrity increases.37,38 To elucidate the mechanism of the increase in bulk modulus caused by atomic density and hydrogen concentrations, more direct evidence for the atomic bond structure including bond length distributions was collected first.
Fig. 3 shows the calculated results of the bond length and bond angle distributions for the dense a-C film with the highest atomic density of 4.0 g cm−3 and those for the H-added a-C film with the highest H concentration of 31.2 at%, in which the highest bulk modulus is observed, as shown in Fig. 2. For comparison, the case for the Ref. a-C film was also considered. The total bond length and bond angle distributions shown in Fig. 3 mainly consist of a C–C bond. Li et al.39 revealed that the distortion of bond lengths (<1.42 Å) and bond angles (>120°) in the carbon network was the key factor for the low level of the bulk modulus. Therefore, the bond lengths and bond angles in these three a-C films were particularly studied in order to gain the fractions of distorted bonds, which are illustrated in Table 1. The equilibrium bond lengths of stable sp2 and sp3 C–C bonds are 1.42 and 1.54 Å, respectively (the black vertical dotted lines in Fig. 3). The fractions of the distorted bond lengths (<1.42 Å) for the dense a-C film with the highest atomic density (4.0 g cm−3, sp3 content = 95.3%) and the H-added a-C film at maximum at% (31.2%, sp3 content = 76.6%) were deduced separately, as shown in Table 1. The calculations for the Ref. a-C film were also obtained for comparison.
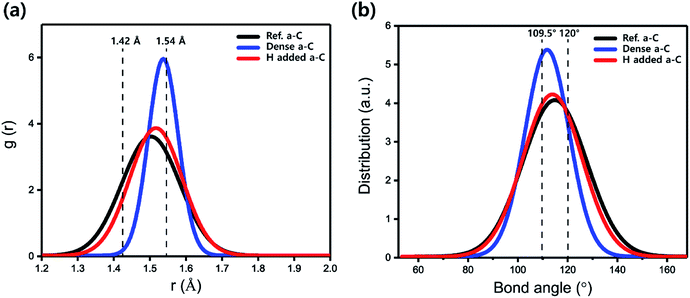 |
| Fig. 3 (a) Bond length distributions and (b) bond angle distributions for Ref. a-C, dense a-C, and H-added a-C films, respectively. | |
Table 1 Comparison of sp3 content, fractions of the distorted bond, and bulk modulus in Ref. a-C, dense a-C, and H-added a-C films
|
Ref. a-C |
Dense a-C |
H-added a-C |
sp3 content (%) |
54.7 |
95.3 |
76.6 |
Fraction of distorted bond length (%) |
17.2 |
1.0 |
14.3 |
Fraction of distorted bond angle (%) |
35.8 |
15.6 |
33.1 |
Bulk modulus (GPa) |
212 |
617 |
248 |
For the Ref. a-C film (Fig. 3), the fractions of the distorted bond length and bond angle are 17.2%, and 35.8%, respectively. For the dense a-C film, the fractions are 1.0% and 15.6%. However, after adding H into the a-C film, the fractions are 14.3% and 33.1%, showing similar values to both the bulk modulus and the fraction of the distorted bond length of the Ref. a-C film. This indicates that even the smaller bulk modulus than that of the dense a-C film is attributed to the high fraction of the distorted C–C bond relaxed by lattice vibrations during stress calculations using VASP.
So-Yeon Lee et al.,40 reported that the RDF of the a-C films can be extracted from diffraction pattern measurements (mean bond length = 1.50 Å for the dense a-C film with sp3 content = 78.5%; mean bond length = 1.45 Å for the normal a-C film with sp3 content = 52.6%). These experimental RDF values show a similar trend with our DFT calculation results (mean bond length = 1.53 Å for the dense a-C film with sp3 content = 95.3%; mean bond length = 1.50 Å for the Ref. a-C film with sp3 content = 54.7%); however, there is slight discrepancy because the atoms in amorphous materials are bonded in randomly disordered positions due to the tendency that does not allow the formation of a regular atomic arrangement.
3.2. Influence of the atomic density and hydrogen concentration on diffusion profile of F atom in the point of energetics
During the dry etch process, the injection of etchant molecules such as CFx leads to the penetration of F atoms through the a-C film up to the sub-layers. To enhance the performance of the a-C film as an etch hard mask, the a-C film has to block the F atoms on the top surface to diffuse downward up to the sub-layers, which are very important layers in highly integrated devices. For F diffusion to proceed, the F atom can pass though the dopant atom in the a-C films.
The optimized structures of the first, second, third, and fourth states for F penetration in the a-C films are shown in Fig. 4: (a) Ref. a-C film, (b) dense a-C film (at the highest density), (c) H-added a-C film (at the highest H concentration). Fig. 5 shows the minimum energy path of F diffusion, corresponding to the 1st to 4th state in Fig. 4. The 1st state shows the energy profile of the downward diffusion of F atoms initially adsorbed on the top layer of the a-C films. In Fig. 4(a–c), Ref. and H-added a-C films show that the F atom diffuses downward and is bound to the sp2 carbon atom.
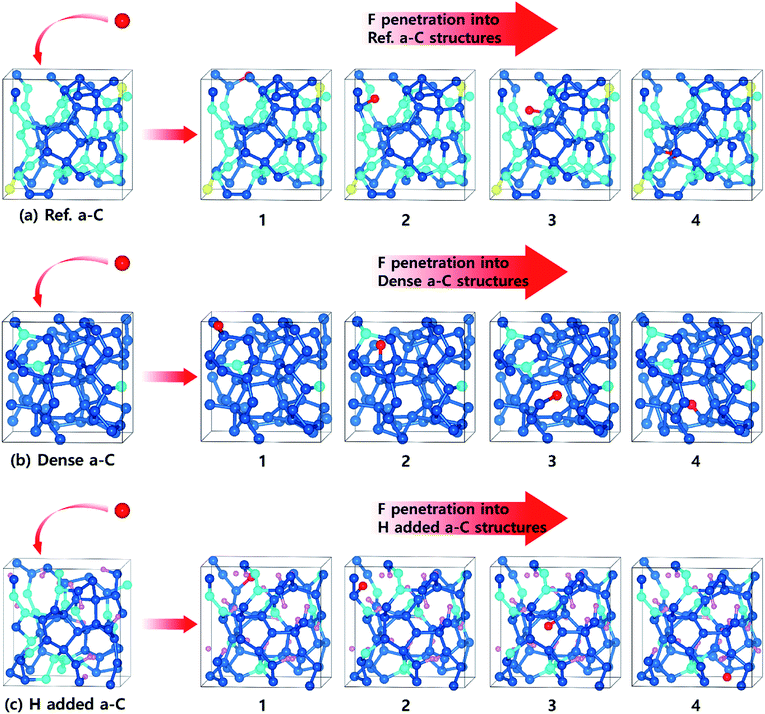 |
| Fig. 4 The optimized structures of the first, second, third, and fourth states for the F penetration into the a-C films; (a) Ref. a-C, (b) dense a-C, and (c) H-added a-C films. | |
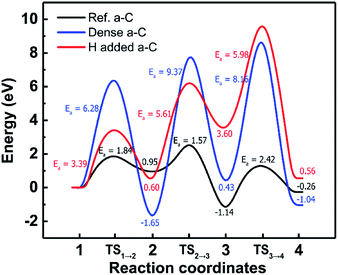 |
| Fig. 5 Minimum energy path of F diffusion, corresponding to the initial, transition, and final states; Ref. a-C (black), dense a-C (blue), and H-added a-C (red) films. Ea means activation energy obtained from NEB calculations. | |
Within the dense a-C film, the F atom diffuses downward and is bound to the surrounding carbon atom by breaking the C–C bond. The interesting point is that the F atom surrounded by sp3 C atoms has much higher barriers compared to that for the Ref. a-C and H-added a-C films. This implies that the sp2 C atoms play an important role in determining the diffusion path of the F atom because of the higher reactive nature of sp2 C than that of sp3 C.19 Furthermore, in our previous study, we reported that the energy barrier for the diffusion of an F atom through the sp3 bonding path is much higher than that for an F atom through the sp2 boding path in a-C films.40
The energy diagram in Fig. 5 clearly shows that the dense a-C film has the highest energy barrier, which can be explained in two perspectives, that is, sp3 content and atomic density. In the perspective of the sp3 content, an increase in the sp3 content causes the penetration of the F atom to weaken due to the decrease in the sp2 carbon sites, enabling the formation of the C–F bonds and strengthening the blocking nature for F penetration. From the perspective of atomic density, an increase in the density leads to an increase in the sp3 content and then hinders the diffusion of F atoms due to the surrounding dense sp3 C atoms. Thus, the change in the sp3 content by both atomic density and H concentration significantly impacts the blocking characteristic against the F atom.
3.3. Dynamic behaviors at finite temperatures
AIMD simulations were performed to examine the dynamic behavior of these three a-C structures. A simulation temperature of 1000 K was chosen. To better understand the dynamic properties, AIMD simulations were also performed to estimate the F mobility within these three a-C structures at 1000 K. The MD duration of 10 ps was used to calculate the mean-square displacements (MSD). Fig. 6 shows the variations in MSD as the simulation time progresses. The diffusion coefficients of F atoms can be calculated based on the Einstein relation:
here, Ri is the atomic position; broken brackets denote thermal averages and t is the time.
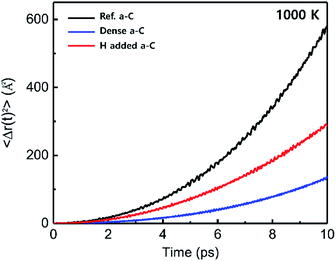 |
| Fig. 6 Variations in the diffusivities of the F atom within the a-C films; Ref. a-C (black), dense a-C (blue), and H-added a-C (red) films. | |
For the Ref. a-C structure, the diffusion coefficient of F was predicted to be DF = 9.66 × 10−4 cm2 s−1. The diffusivities of the dense and H-added a-C structures were estimated to be 2.22 × 10−4 and 4.83 × 10−4 cm2 s−1, respectively. These calculations clearly show that F mobility tends to be hindered by both the atomic density and H concentration. The drastic reduction in F diffusivities within the dense and H-added a-C structures can be explained by the enhancement in the characteristic barrier of F diffusion, as aforementioned in Section 3.2.
Table 2 shows the comparison of the sp3 contents, activation energies (Ea, eV), and diffusion coefficients (DF, cm2 s−1) for F diffusion for the Ref., dense, and H-added a-C films. This table clearly shows that the change in the sp3 content by both atomic density and H concentration makes the diffusion barrier of the F atom even higher and suppresses the F diffusion, meaning that the F atom would follow the diffusion path passing through the sp2 carbon and not the sp3 carbon due to the significantly high barriers of 3.88 eV and 7.04 eV, respectively.
Table 2 Comparison of sp3 content, activation energies (Ea, eV), and diffusion coefficients (DF, cm2 s−1) for F diffusion on Ref., dense, and H-added a-C films
|
Ref. a-C |
Dense a-C |
H-added a-C |
sp3 content (%) |
54.7 |
95.3 |
76.6 |
Ea,min (eV) |
1.57 |
3.39 |
6.28 |
Ea,max (eV) |
2.42 |
5.98 |
9.37 |
DF (cm2 s−1) |
9.66 |
2.22 |
4.83 |
3.4. Discussion
According to several studies on the etch process,41–43 during the initial etch process (within 10 s), etching is very fast because it has an unstable atomistic structure by dangling bonds of the top-surface atoms. However, after the initial etch of on the surface, the bulk part of the thin film starts to get slowly etched due to its characteristics of stable atomic bonds and condensed atomic arrangement. Due to this slow etching, the bulk etch process determines the overall etch reaction rate. Therefore, the reason we only considered the “bulk” part is that during the real etch process, the “surface” part takes place in a very short time and most of the time is required for the “bulk” part. In fact, many other researchers in the fields of etch processes44–46 have controlled the etch profile by tuning the “density” of thin films, which is a very important “bulk” property that can be easily tuned by the etch chemistry ratio, temperature, and plasma power.
Our DFT calculation shows that increasing the atomic density of the a-C films dramatically increases the bulk modulus. Similarly, several researchers reported experimentally that the bulk modulus of a-C is proportional to density.12,47–49 Ito A. M. et al.49 explained the dependence of the bulk modulus on density by counting the covalent bonds in a-C materials. They revealed that the number of covalent bonds increased in proportion to the film density as well as the bulk modulus. Other researchers50,51 stated that an increase in the modulus is attributed to the change from sp2 to sp3 bonds with an increase in the density. The combination of their results and our DFT calculated results for the relation between density and bulk modulus in actual thin films provides deep insights for analyzing the thin film chemistry and physics from this approach.
All our DFT calculated results suggest that the dense a-C film would be difficult to apply for highly integrated devices due to the high bulk modulus even though this film has a superior F blocking nature. On the other hand, the H-added a-C film would have an advantage for highly integrated devices due to the excellent F blocking nature by keeping the bulk modulus from rising steeply.
Even though a-C films with increasing atomic density and H concentration have not been reported as an etch hard mask, our DFT calculated results suggest that the H-added a-C films would have outstanding characteristics such as mechanical properties and blocking nature of F atoms for an etch hard mask in ultra-high integrated semiconductor devices. In our DFT calculated results, the combinational study of atomic density and H concentration in the a-C films would provide a deep insight into improving the mechanical properties and etch selectivity of the films, enhancing the performance of the future memory devices.
4. Conclusions
We investigated the energetics, structure, and mechanical properties of a-C films with an increase in the sp3 content by adjusting the atomic density or hydrogen content based on DFT and AIMD calculations. Drastic increase in bulk modulus was observed by increasing the atomic density of the a-C films, which suggested that it would be difficult for the films hardened by high atomic density to relieve the stress of the individual layers within the overall stack in integrated semiconductor devices. However, the addition of hydrogen into the a-C films had little effect on increasing the bulk modulus even though the sp3 content increased. These findings suggest that the process design for high-sp3-content a-C films should be carried out by adding hydrogen rather than increasing atomic density. These requirements become more important and increasingly challenging to meet as the device integrity increases. In the perspective of the F blocking nature, a change in the sp3 content by both atomic density and H concentration makes the diffusion barrier of the F atom even higher and suppresses the F diffusion, meaning that the F atom would follow the diffusion path that passes the sp2 carbon and not the sp3 carbon due to the significantly high barrier. For the material design of a-C films with adequate doped characteristics, our results can provide a new straightforward strategy to tailor the a-C films with excellent mechanical and other novel physical and chemical properties.
Conflicts of interest
There are no conflicts to declare.
Acknowledgements
Euijoon Yoon and Gun-Do Lee acknowledge support from the Supercomputing Center/Korea Institute of Science and Technology Information with supercomputing resources (KSC-2017-C3-0020), from the joint program for Samsung Electronics Co., Ltd. (SEC), from BK21PLUS SNU Materials Division for Educating Creative Global Leaders (21A20131912052), and from the National Research Foundation of Korea (NRF) grant funded by the Korea government (No. RIAM 2019R1A2C2005098) and the Ministry of Science, ICT and Future Planning (2017M3D1A1040688). Hwanyeol Park acknowledge support from the Supercomputing Center/Korea Institute of Science and Technology Information with supercomputing resources (KSC-2019-CRE-0148). The computation data to support the findings of this paper is available from the corresponding author on reasonable request. The code to analyze ab initio molecular dynamics simulation is available at: https://github.com/mogroupumd/aimd.
References
- J. Robertson, Diamond-like amorphous carbon, Mater. Sci. Eng. R Rep., 2002, 37(4–6), 129–281 CrossRef.
- L. Zhang, X. Wei, Y. Lin and F. Wang, A ternary phase diagram for amorphous carbon, Carbon, 2015, 94, 202–213 CrossRef CAS.
- L. Wang, R. Zhang, U. Jansson and N. Nedfors, A near-wearless and extremely long lifetime amorphous carbon film under high vacuum, Sci. Rep., 2015, 5, 11119 CrossRef PubMed.
- X. Li, P. Guo, L. Sun, A. Wang and P. Ke, Ab Initio Investigation on Cu/Cr Codoped Amorphous Carbon Nanocomposite Films with Giant Residual Stress Reduction, ACS Appl. Mater. Interfaces, 2015, 7, 27878–27884 CrossRef CAS PubMed.
- G. Cicala, P. Bruno and A. M. Losacco, PECVD of hydrogenated diamond-like carbon films from CH4–Ar mixtures: growth chemistry and material characteristics, Diamond Relat. Mater., 2004, 13, 1361–1365 CrossRef CAS.
- Y. T. Kim, S. M. Cho, W. S. Choi, B. Hong and D. H. Yoon, Dependence of the bonding structure of DLC thin films on the deposition conditions of PECVD method, Surf. Coat. Technol., 2003, 169–170, 291–294 CrossRef CAS.
- H. Yang, G. A. Brown, J. C. Hu, J. P. Lu, R. Kraft, A. L. P. Rotondaro, S. V. Hattangady, I. Chen, J. D. Luttmer, R. A. Chapman, P. J. Chen, H. L. Tsai, B. Amirhekmat and L. K. Magel, A comparison of TiN processes for CVD W/TiN gate electrode on 3 nm gate oxide, in International Electron Devices Meeting, IEDM Technical Digest, 1997, pp. 459–462 Search PubMed.
- M. Dammann, M. Chertouk, W. Jantz, K. Köhler and G. Weimann, Reliability of InAlAs/InGaAs HEMTs grown on GaAs substrate with metamorphic buffer, Microelectron. Reliab., 2000, 40, 1709–1713 CrossRef.
- J. Robertson, Electronic and atomic structure of diamond-like carbon, Semicond. Sci. Technol., 2003, 18, S12–S19 CrossRef CAS.
- N. Maître, S. Camelio, A. Barranco, T. Girardeau and E. Breelle, Physical and chemical properties of amorphous hydrogenated carbon films deposited by PECVD in a low self-bias range, J. Non-Cryst. Solids, 2005, 351, 877–884 CrossRef.
- N. Fourches and G. Turban, Plasma deposition of hydrogenated amorphous carbon: growth rates, properties and structures, Thin Solid Films, 1994, 240, 28–38 CrossRef CAS.
- J. Robertson, Diamond-like amorphous carbon, Mater. Sci. Eng., R, 2002, 37, 129–281 CrossRef.
- T. Mikami, H. Nakazawa, M. Kudo and M. Mashita, Effects of hydrogen on film properties of diamond-like carbon films prepared by reactive radio-frequency magnetron sputtering using hydrogen gas, Thin Solid Films, 2005, 488, 87–92 CrossRef CAS.
- E. Vietzke, K. Flaskamp, V. Philipps, G. Esser, P. Wienhold and J. Winter, Chemical erosion of amorphous hydrogenated carbon films by atomic and energetic hydrogen, J. Nucl. Mater., 1987, 145–147, 443–447 CrossRef CAS.
- C. Wei, K.-S. Peng and M.-S. Hung, The effect of hydrogen and acetylene mixing ratios on the surface, mechanical and biocompatible properties of diamond-like carbon films, Diamond Relat. Mater., 2016, 63, 108–114 CrossRef CAS.
- M. Silinskas, A. Grigonis, V. Kulikauskas and I. Manika, Hydrogen influence on the structure and properties of amorphous hydrogenated carbon films deposited by direct ion beam, Thin Solid Films, 2008, 516, 1683–1692 CrossRef CAS.
- R.-h. Zhang, L.-p. Wang and Z.-b. Lu, Probing the intrinsic failure mechanism of fluorinated amorphous carbon film based on the first-principles calculations, Sci. Rep., 2015, 5, 9419 CrossRef PubMed.
- X. Li, P. Ke and A. Wang, Probing the Stress Reduction Mechanism of Diamond-Like Carbon Films by Incorporating Ti, Cr, or W Carbide-Forming Metals: Ab Initio Molecular Dynamics Simulation, J. Phys. Chem. C, 2015, 119, 6086–6093 CrossRef CAS.
- H. Park, S. Lee, H. J. Kim, D. Woo, S. J. Park, J. M. Lee, E. Yoon and G.-D. Lee, Effects of nitrogen doping in amorphous carbon layers on the diffusion of fluorine atoms: a first-principles study, J. Appl. Phys., 2019, 125, 155701 CrossRef.
- R. Haerle, A. Pasquarello and A. Baldereschi, First-principle study of C 1s core-level shifts in amorphous carbon, Comput. Mater. Sci., 2001, 22, 67–72 CrossRef CAS.
- R. Haerle, E. Riedo, A. Pasquarello and A. Baldereschi, sp2/sp3 hybridization ratio in amorphous carbon from C 1s core-level shifts: X-ray photoelectron spectroscopy and first-principles calculation, Phys. Rev. B: Condens. Matter Mater. Phys., 2001, 65, 045101 CrossRef.
- H. Park, D. Woo, J. M. Lee, S. J. Park, S. Lee, H. J. Kim, E. Yoon and G.-D. Lee, First principles investigation on energetics, structure, and mechanical properties of amorphous carbon films doped with B, N, and Cl, Sci. Rep., 2019, 9, 18961 CrossRef CAS PubMed.
- J. P. Perdew, K. Burke and M. Ernzerhof, Generalized gradient approximation made simple, Phys. Rev. Lett., 1996, 77, 3865–3868 CrossRef CAS PubMed.
- G. Kresse and J. Furthmuller, Efficient iterative schemes for ab initio total-energy calculations using a plane-wave basis set, Phys. Rev. B: Condens. Matter Mater. Phys., 1996, 54, 11169–11186 CrossRef CAS PubMed.
- S. Grimme, Semiempirical GGA-type density functional constructed with a long-range dispersion correction, J. Comput. Chem., 2006, 27, 1787–1799 CrossRef CAS PubMed.
- P. E. Blöchl, Projector augmented-wave method, Phys. Rev. B: Condens. Matter Mater. Phys., 1994, 50, 17953–17979 CrossRef PubMed.
- W. Zhai, X. Song, T. Li, B. Yu, W. Lu and K. Zeng, Ti Reactive Sintering of Electrically Conductive Al2O3–TiN Composite: Influence of Ti Particle Size and Morphology on Electrical and Mechanical Properties, Materials, 2017, 10, 1348 CrossRef.
- D. Vanderbilt, Soft self-consistent pseudopotentials in a generalized eigenvalue formalism, Phys. Rev. B: Condens. Matter Mater. Phys., 1990, 41, 7892–7895 CrossRef PubMed.
- G. Henkelman, B. P. Uberuaga and H. Jónsson, A climbing image nudged elastic band method for finding saddle points and minimum energy paths, J. Chem. Phys., 2000, 113, 9901–9904 CrossRef CAS.
- G. Mills and H. Jónsson, Quantum and thermal effects in ${\mathrm{H}}_{2}$ dissociative adsorption: evaluation of free energy barriers in multidimensional quantum systems, Phys. Rev. Lett., 1994, 72, 1124–1127 CrossRef CAS PubMed.
- D. R. Alfonso and K. D. Jordan, A flexible nudged elastic band program for optimization of minimum energy pathways using ab initio electronic structure methods, J. Comput. Chem., 2003, 24, 990–996 CrossRef CAS PubMed.
- H. Park, S. Lee, H. J. Kim, E. Yoon and G.-D. Lee, Dissociation reaction of B2H6 on TiN surfaces during atomic layer deposition: first-principles study, RSC Adv., 2017, 7, 55750–55755 RSC.
- H. Park, S. Lee, H. J. Kim, D. Woo, S. J. Park, K. Kim, E. Yoon and G.-D. Lee, Effects of H2 and N2 treatment for B2H6 dosing process on TiN surfaces during atomic layer deposition: an ab initio study, RSC Adv., 2018, 8, 21164–21173 RSC.
- H. Park, S. Lee, H. J. Kim, D. Woo, J. M. Lee, E. Yoon and G.-D. Lee, Overall reaction mechanism for a full atomic layer deposition cycle of W films on TiN surfaces: first-principles study, RSC Adv., 2018, 8, 39039–39046 RSC.
- H. Park, E. Yoon, G.-D. Lee and H. J. Kim, Analysis of surface adsorption kinetics of SiH4 and Si2H6 for deposition of a hydrogenated silicon thin film using intermediate pressure SiH4 plasmas, Appl. Surf. Sci., 2019, 496, 143728 CrossRef CAS.
- I. N. Remediakis, M. G. Fyta, C. Mathioudakis, G. Kopidakis and P. C. Kelires, Structure, elastic properties and strength of amorphous and nanocomposite carbon, Diamond Relat. Mater., 2007, 16, 1835–1840 CrossRef CAS.
- H. Ito, M. Kuwahara, R. Ohta and M. Usui, Behavior of stress generated in semiconductor chips with high-temperature joints: influence of mechanical properties of joint materials, J. Appl. Phys., 2018, 123, 145109 CrossRef.
- M. Koganemaru, K. Yoshida, T. Ikeda, N. Miyazaki and H. Tomokage, Device simulation for evaluating effects of mechanical stress on semiconductor devices: impact of stress-induced variation of electron effective mass, in 3rd Electronics System Integration Technology Conference ESTC, 2010, pp. 1–6 Search PubMed.
- X. Li, P. Ke, H. Zheng and A. Wang, Structural properties and growth evolution of diamond-like carbon films with different incident energies: a molecular dynamics study, Appl. Surf. Sci., 2013, 273, 670–675 CrossRef CAS.
- S.-Y. Lee, K.-T. Jang, M.-W. Jeong, S. Kim, H. Park, K. Kim, G.-D. Lee, M. Kim and Y.-C. Joo, Bonding structure and etching characteristics of amorphous carbon for a hardmask deposited by DC sputtering, Carbon, 2019, 154, 277–284 CrossRef CAS.
- H. Zhu, J. Hüpkes, E. Bunte, J. Owen and S. M. Huang, Novel etching method on high rate ZnO:Al thin films reactively sputtered from dual tube metallic targets for silicon-based solar cells, Sol. Energy Mater. Sol. Cells, 2011, 95, 964–968 CrossRef CAS.
- D. H. van Dorp, S. Arnauts, F. Holsteyns and S. De Gendt, Wet-Chemical Approaches for Atomic Layer Etching of Semiconductors: Surface Chemistry, Oxide Removal and Reoxidation of InAs (100), ECS J. Solid State Sci. Technol., 2015, 4, N5061–N5066 CrossRef CAS.
- J. Provine, P. Schindler, Y. Kim, S. P. Walch, H. J. Kim, K.-H. Kim and F. B. Prinz, Correlation of film density and wet etch rate in hydrofluoric acid of plasma enhanced atomic layer deposited silicon nitride, AIP Adv., 2016, 6, 065012–0650128 CrossRef.
- Z. Ming, X. De Yuan, P. S. Min, H. Z. Shan and S. Y. Xie, Experimental study of multilayer SiCN barrier film in 45/40 nm technological node and beyond, Microelectron. Reliab., 2016, 57, 86–92 CrossRef CAS.
- T.-S. Choi and D. W. Hess, Chemical Etching and Patterning of Copper, Silver, and Gold Films at Low Temperatures, ECS J. Solid State Sci. Technol., 2014, 4, N3084–N3093 CrossRef.
- N. Kuboi, T. Tatsumi, H. Minari, M. Fukasawa, Y. Zaizen, J. Komachi and T. Kawamura, Influence of hydrogen in silicon nitride films on the surface reactions during hydrofluorocarbon plasma etching, J. Vac. Sci. Technol., A, 2017, 35, 061306 CrossRef.
- J. Robertson, Deposition mechanisms for promoting sp3 bonding in diamond-like carbon, Diamond Relat. Mater., 1993, 2, 984–989 CrossRef CAS.
- P. J. Fallon, V. S. Veerasamy, C. A. Davis, J. Robertson, G. A. J. Amaratunga, W. I. Milne and J. Koskinen, Properties of filtered-ion-beam-deposited diamondlike carbon as a function of ion energy, Phys. Rev. B: Condens. Matter Mater. Phys., 1993, 48, 4777–4782 CrossRef CAS PubMed.
- A. M. Ito, A. Takayama, Y. Oda and H. Nakamura, The first principle calculation of bulk modulus and Young's modulus for amorphous carbon material, J. Phys.: Conf. Ser., 2014, 518, 012011 CrossRef.
- A. C. Ferrari, B. Kleinsorge, N. A. Morrison, A. Hart, V. Stolojan and J. Robertson, Stress reduction and bond stability during thermal annealing of tetrahedral amorphous carbon, J. Appl. Phys., 1999, 85, 7191–7197 CrossRef CAS.
- J. Robertson, Mechanical properties and coordinations of amorphous carbons, Phys. Rev. Lett., 1992, 68, 220–223 CrossRef CAS PubMed.
Footnote |
† Electronic supplementary information (ESI) available. See DOI: 10.1039/c9ra09328a |
|
This journal is © The Royal Society of Chemistry 2020 |
Click here to see how this site uses Cookies. View our privacy policy here.