DOI:
10.1039/C9RA10079B
(Paper)
RSC Adv., 2020,
10, 8480-8489
Construction of octenyl succinic anhydride modified porous starch for improving bioaccessibility of β-carotene in emulsions†
Received
2nd December 2019
, Accepted 24th January 2020
First published on 27th February 2020
Abstract
Modified porous starch (PS), by introducing octenyl succinic anhydride (OSA) moieties, was synthesized successfully, which was applied as an emulsion of β-carotene for the first time. The pores and channels within porous starch provided more possibilities for OSA to modify starch. The ester linkage of OSA modified PS with different degrees of substitution (DS) were confirmed by both 13C solid-state NMR and Fourier transform-infrared spectroscopy (FT-IR). The hydrophobic octenyl succinic and hydrophilic hydroxyl groups of OSA modified PS showed the good emulsifying capability, which could be utilized to prepare β-carotene emulsions. And the bioaccessibility of β-carotene was also enhanced with increasing DS of OSA modified starch. This study not only paves a new way using porous starches for modification of starch, but also offers an attractive alternative for obtaining emulsion-based delivery systems for bioactive components.
1. Introduction
Over the last decades, great efforts have been devoted to the association between diet and chronic diseases. Based on the convincing evidence from scientific research, the consumption of fruits and vegetables, which are good sources of carotenoids and other bioactive compounds, plays an important role in the prevention of human diseases.1 carotenoids, including β-carotene, lutein and neoxanthin, represent a large family of tetraterpenoid organic pigments, which are widely found in various colorful fruits and vegetables.2,3 As one type of carotenoid, β-carotene showed highly desirable bioactivities such as health-promoting properties, and antioxidant activity, which can maintain human health and prevent chronic diseases including cardiovascular diseases, cancer and other chronic diseases.4,5 Due to the low stability, solubility and bioaccessibility, the supplemented foods of β-carotene were greatly limited.3–6 It was worth noting that utilization of an emulsion-based delivery system is considered as an effective method for overcoming the limitations of β-carotene. The conventional emulsifying agent, including Tween 20, and polyglycerol esters,7–9 showed the excellent ability of emulsification for fabrication of colloidal system. For example, Tween 20 has been applied as an emulsifier to prepare β-carotene nano-dispersions for the first time by Tan et al. However, the chemical emulsifiers may pose a potential threat to human health in the field of foods and beverages, especially for the consumption at high-level.10 With an increasing concern on the safety of the delivery system, there is an increasingly urgent need to search for a safe emulsifier as a candidate. Emulsions from biopolymers of plants and microbial, possessing higher safety in food-grade, are considered as potential alternatives for fabrication of emulsions.3 The application of biopolymer-based emulsifiers was labeled as plant sources surfactants than the synthetic and semi-synthetic one.11 Previous research suggested that biopolymer emulsifiers, including proteins12 and polysaccharides,13 have been applied for the fabrication of the delivery system of carotenoid successfully. Among these food-grade biopolymer emulsifiers, starches chemically modified by different alkenyl succinic anhydrides, possessing the favorable hydrophobicity, are suitable to stabilize emulsions.14 For example, octenyl succinic anhydride modified starch, which is synthesized by esterification between the hydroxyl group of the starch and the carboxyl group of OSA, has also been utilized for preparing β-carotene emulsions due to its strong surface activity and good emulsifying properties.3,14,15 Various studies indicated that hydrophobic octenyl and carboxyl groups, derived from OSA modified starch, can improve the emulsifying capability of starch.16,17 Meanwhile, the degree of substitution has also a great influence on the emulsification effect of OSA modified starch. It has been proved that the emulsions with the larger DS exhibited the less flocculation and coalescence.18,19 It is well known that the DS of OSA modified starch is greatly affected by the process of esterification.20 However, the esterification reaction of OSA modified starch was restricted by the naturally low surface areas of starch, which resulted in the low DS.21 Therefore, exploring the efficient approach with more reactive sites for esterification is a great challenge in this field. It was worth noting that, porous starch is a type of well-known modified starch, which have the high surface areas22,23 to overcome the above limitation of native starches.
Porous starch, obtained by the physical, chemical, and enzyme treatment, is a modified starch with abundant pores, which extended from the surface to the center of starch granules.24 Derived from these micro-sized pores and channels, porous starch possessed the larger specific surface area, which showed the more chemically reactive sites than that of starch.22,23,25 Many studies also proved that the modification of porous starch gives a higher DS than the native one.23 The pores and channels within porous starch provided more possibilities for chemical agents to penetrate easily into the interior of starch for further modifications. Therefore, the higher DS of esterified starch could be obtained using porous starch instead of the native one. Porous starch may be very suitable as a host material for further OSA modification. To the best of our knowledge, few researches have been reported about the fabrication and application of OSA modification using porous starch.
In this study, porous starch modified by introducing OSA moieties was synthesized successfully, which was applied as an emulsion of β-carotene for the first time. As shown in Fig. 1, the schematic illustration was exhibited to illustrate entire process. Porous starch synthesized by enzymatic hydrolysis, was modified by esterification with OSA groups to form OSA modified porous starch (OSA@PS). In comparison with native starch (NS), PS has the larger surface areas, which could offer more reaction sites. Thus, at the same experimental condition, the greater DS of OSA modified starch should be obtained using PS instead of NS. The successful formation of the ester linkage was confirmed by both 13C solid-state NMR spectroscopy and FT-IR. The crystalline and porous structures were characterized by X-ray diffraction analysis (XRD) and scanning electron microscopy (SEM) respectively. Furthermore, OSA modified porous starch was applied as the emulsifier to fabricate β-carotene emulsions. By mimicking digestion process in oral, gastric and intestinal fluid, the digestion test in vitro was carried out to determine the bioaccessibility of β-carotene after emulsions.
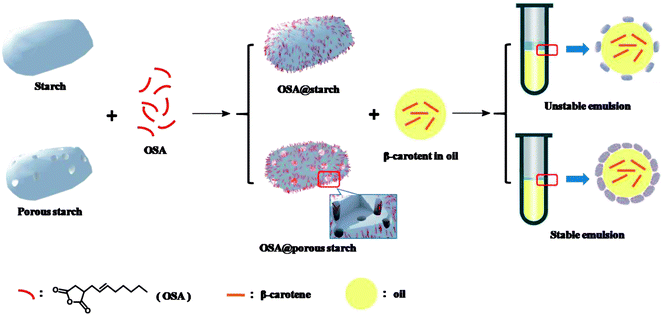 |
| Fig. 1 Schematic representation of synthesis and application of OSA modified porous starch and native starch. | |
2. Materials and methods
2.1 Materials
Corn starch, α-amylase (AM, 50 U mg−1), amyloglucosidase (AMG, 100 U mL−1), octenyl succinic anhydride, were purchased from Shanghai Yuanye Biotechnology Co., Ltd. All the reagents used were of analytical grade unless otherwise stated.
2.2 Methods
2.2.1 Preparation of porous starch. The porous starch was prepared according to the reported procedures24 with a slight modification: native corn starch (10.0 g) was suspended in 60 mL phosphate buffer at pH 6.6 and in 20 mL acetate mixed buffer at pH 4.5, stirred in a water bath at 40 °C for 20 min. The mixed enzyme, whose ratio of α-amylase (50 U mg−1) and amyloglucosidase (100 U mL−1) was 6
:
1, were added into the suspension. The samples were kept in a shaking water bath at 50 °C for 24 h. Then, the pH was adjusted to 10.0 by adding 1 M NaOH solution. The suspension was centrifuged and washed using the distilled water. Finally, the collected precipitate was dried at 50 °C to yield the porous starch. The product was ground through a 90 mesh sieve in a desiccator for further use.
2.2.2 Preparation of OSA modified NS and PS. OSA modified NS or PS samples was prepared according to the previous method of Song et al.26 Corn starch or porous starch (5 g) was suspended in water (30%, w/w). The pH of suspension was adjusted to 8.5 by the addition of 3% NaOH solution. 3% and 5% OSA solution, diluted with ethanol, were added dropwise to the starch slurry within 2 h respectively. The reaction was maintained at 35 °C for 1 h, and then the pH was adjusted to 6.5. The mixture was centrifuged and washed with water and ethanol respectively. The precipitation was dried at 40 °C to yield the modified starches. The products were subsequently referred to as 3% OSA modified native starch (3% OSA@NS) and 5% OSA@NS, 3% OSA modified porous starch (3% OSA@PS) and 5% OSA@PS, respectively.
2.2.3 Determination of degree of substitution. The DS was measured according to the titration method described by Song et al.26 DS was calculated using the following formula: |
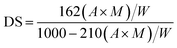 | (1) |
where 162 is the molar mass (g mol−1) of the glucose residue, 210 is the molar mass of OSA, A represents the titration volume (mL) of the NaOH solution, M represents the molar concentration (mol L−1) of the NaOH solution, and W represents the dry weight (g) of sample.Free OSA content of OSA modified starches was measured by 1H NMR experiments referred to the method of Tizzotti et al.27
2.2.4 Structural characterization analysis of modified starch.
2.2.4.1 13C solid-state NMR spectroscopy. Solid-state NMR measurements were carried out on a Bruker WB Avance II 400 MHz spectrometer. The transmitter frequency of 13C NMR is 100.60 MHz. The solid-state 13C cross-polarization magic angle spinning (CP/MAS) NMR spectra were recorded with a 4 mm double-resonance MAS probe and with a spinning rate of 10.0 kHz; a pulse delay of 3 s was applied during data acquisition with a contact time of 2.5 ms (ramp 100). The 13C chemical shifts were referenced to the signal of tetramethylsilane as 0 ppm.
2.2.4.2 Fourier transform-infrared spectroscopy. The FT-IR spectra of NS, PS, OSA@NS and OSA@PS were determined by FT-IR spectroscopy (Nicolet NEXUS 670, American). Scanning was performed from 4000–450 cm−1 at a resolution of 4 cm−1.
2.2.4.3 X-ray diffraction analysis. The crystal structures of NS, PS, OSA@NS and OSA@PS were characterized by X-ray diffract meter (Shimadzu, XRD-6000, Japan). The test conditions were as follows: voltage: 40 kV; current: 40 mA; scanning speed: 2° min−1; scanning step size: 0.06°; scanning method: continuous. The relative crystallinity (%) was quantified as the ratio of the crystalline area to the total area under the diffractogram.
2.2.4.4 Scanning electron microscopy. The morphology of NS, PS, OSA@NS and OSA@PS were observed using an SEM instrument (JSM-6701F, Electron Optics Inc., Japan). The shape and surface characteristics of the samples were observed at an accelerating voltage of 5 kV.
2.2.5 Preparation of emulsions. For the preparation of emulsions, the reported method of Lin et al.19 was used and appropriately modified as follows: the OSA modified starch (5%, w/w) was suspended in Milli-Q water and stirred in a boiling water bath for 20 min. Then 1.17 mL corn oil containing 0.1% (w/w) β-carotene was added starch gel to obtain a final mixture with 5% (w/w) OSA modified starch and 10% (w/w) oil. The crude emulsion was passed through an ultrahigh pressure homogenizer (Scientz-207A, China) at a mean value of 130 MPa for 2 min with three cycles to prepare an emulsion. All of devices and beakers were wrapped with aluminum foil to avoid light exposure during preparation.
2.2.6 In vitro digestion of emulsion. Simulated saliva fluid (SSF) was prepared refer to the method of Hur et al.28 with appropriate modifications, containing α-amylase and various salts except mucin. Simulated gastric fluid (SGF) and simulated small intestinal fluid (SIF) were prepared according to the literatures.11,29
2.2.7 Particle size measurements. The particle size distribution of the emulsion was measured using a laser particle size distribution analyzer (Bettersize 2600, China). The result is expressed as the surface area average diameter d32, which is defined as Σnidi3/Σnidi2.
2.2.8 Confocal laser scanning microscopy (CLSM). The microstructure of the emulsion was observed using CLSM (Confocal Laser Scanning Microscope, LSM800, Germany). Samples (1 mL) were dyed with 0.5% Nile Red solution. Then, samples were observed by the 63× oil immersion objective lens, with the excitation light of 561 nm (argon), at the reflection wavelength of 525–618 nm. Images were acquired and processed using digital image processing software Leica LAS AF.
2.2.9 Determination of bioaccessibility of β-carotene. After the simulated digestion process, samples were collected and centrifuged at 8000 rpm to obtain three phases. The upper, middle and bottom layer were attributed to thin oil layer, micelle phase and insoluble precipitate respectively. After taking 5 mL of micelle phase through a 0.45 μm microporous membrane, β-carotene was extracted and measured according to the reported method.30 The filtrate was mixed with dimethyl sulfoxide in equal volumes and destructed the structure of emulsion by vortex oscillator process. Next, dichloromethane/n-hexane (1
:
4, v/v) was added, and centrifuged at 4000 rpm. The bottom yellow layer was analyzed by spectrophotometer at 450 nm. The concentration of β-carotene in the original emulsion and micellar phase were determined from a calibration curve. |
 | (2) |
2.2.10 Statistical analysis. The whole experiments were assayed in duplicate with triplicate measurements being made on each sample, and the results were expressed as the mean and standard deviation. The one-way analysis of variance (ANOVA) test was analyzed using the SPSS 22.0 package. Duncan's multiple range test was used to determine the significant differences of the mean values (p < 0.05).
3. Results and discussion
3.1 DS of OSA modified NS and PS
The OSA@NS and OSA@PS possessing different DS were synthesized according to the methods above. With varying the ratio of OSA to NS and PS from 3% to 5%, the DS of OSA@NS was changed from 0.0195 to 0.0289. The DS was enhanced as the increasing concentration of OSA, which showed a similar trend in OSA-treated ginkgo starch.31 It could be interpreted that greater availability of OSA molecules was in the proximity of starch molecule during esterification.32 In comparison with NS, OSA@PS has a larger DS (Table 1), which was proved by the result from 1H NMR of free OSA (ESI Table S1 and Fig. S1†). It was derived from additional pores and channels within the porous starch structure, which provides a larger specific surface area and more reactive sites with OSA.
Table 1 The DS and relative crystallinity (Xc) of varied OSA modified starchesa
Samples |
DS |
Xc (%) |
Values are given as mean ± standard deviation. Different superscript letters in the columns indicate significant difference (p < 0.05). |
NS |
0 |
31.84 ± 0.1380b |
PS |
0 |
30.18 ± 0.1751b |
3% OSA@NS |
0.0195 ± 0.0195d |
33.28 ± 0.0980a |
5% OSA@NS |
0.0228 ± 0.0162c |
31.95 ± 0.1310a |
3% OSA@PS |
0.0260 ± 0.0249b |
28.83 ± 0.1551c |
5% OSA@PS |
0.0289 ± 0.0205a |
28.22 ± 0.0616c |
3.2 Structural characterization of modified NS and PS
3.2.1 13C solid-state NMR spectra. To confirm the formation of ester linkage between porous starch and OSA during the esterification reaction, solid-state 13C CP/MAS NMR analysis was performed on porous starch and OSA modified one (DS = 0.0289) as shown in Fig. 2a and b. In the high field, the peaks centered at 63.5 ppm, 70.5–81.7 ppm and 101.2 ppm were attributed to the signals of C-6, C-2, 3, 4, 5 and C-1 of starch respectively.33 The spectrum of OSA@PS was almost identical to that of PS in high field, indicating the structural preservation after esterification reaction. It was worth noting that the partially enlarged solid-state 13C spectrum of OSA@PS from 120 to 250 ppm was also embedded in Fig. 2c. The peak centered at 174 ppm was observed obviously, which was derived from 13C chemical shift of ester linkage.34 This result indicated that the atomic-level linkages between OSA and PS were constructed successfully through esterification. 13C CP/MAS NMR spectrum of NS was almost identical to that of PS, indicating that enzymatic hydrolysis has no influence on the skeleton of starch at atomic level. Due to the low DS of OSA modified starch and limited resolution of solid-state NMR, the effect of enzymatic hydrolysis on the efficiency of esterification could not be evaluated between NS and PS accurately. Fortunately, this issue could be solved by FT-IR spectroscopy successfully.
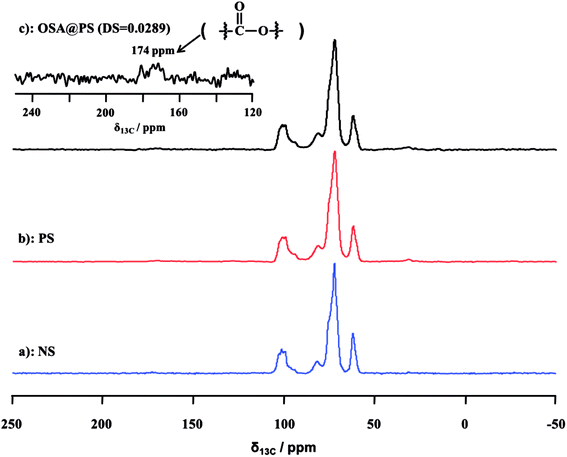 |
| Fig. 2 Solid-state 13C CP/MAS NMR spectra of NS (a), PS (b) and OSA modified PS (c). The peak at 174 ppm was attributed to the ester linkage, indicating that OSA modified PS was obtained successfully. | |
3.2.2 Fourier transform-infrared spectroscopy. Meanwhile, the ester formation was also confirmed in OSA modified PS by FT-IR spectroscopy. The FT-IR spectra of NS, PS, OSA@NS and OSA@PS were shown in Fig. 3. FT-IR spectrum of NS was almost identical to that of PS, indicating that enzymatic hydrolysis has no influence on the skeleton of starch. Compared with the spectra of non-esterified NS and PS, two new absorption bands at 1725 and 1570 cm−1, which were corresponding to the C
O and RCOO– group of the ester, were observed obviously in that of OSA modified NS and PS.35,36 Meanwhile, the signal became increasingly obvious with the raising DS gradually. Compared FT-IR spectra of OSA@NS and OSA@PS, it was worth noting that adsorption bands of C
O (1725 cm−1) and RCOO– (1570 cm−1) of OSA@PS could be observed obviously than that of OSA@NS in FT-IR spectra. This result suggested that enzymatic hydrolysis improved the efficiency of esterification, due to the larger surface areas of PS. This result, which offered the further evidence for the formation of ester linkage, indicated that OSA was successfully introduced into NS and PS by means of esterification reaction.
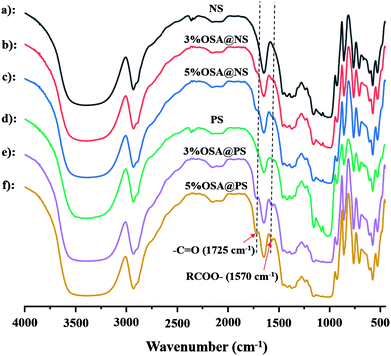 |
| Fig. 3 FT-IR spectra of starch and OSA modified starches ((a) NS; (b) 3% OSA@NS; (c) 5% OSA@NS; (d) PS; (e) 3% OSA@PS; (f) 5% OSA@PS). The bands of OSA modified starch at 1725 cm−1 and 1570 cm−1 correspond to the stretching vibration of C O and the asymmetric stretching vibration of RCOO–, indicating the successful formation of the ester linkage. | |
3.2.3 X-ray diffraction analysis. To verify the crystalline structure of OSA modified starch before and after esterification reaction, the XRD analysis was carried out on NS, PS, OSA@NS and OSA@PS, as shown in Fig. 4. The XRD patterns of all samples, with diffraction peaks at 15°, 17°, 18° and 23°, were attributed to the typical A-type crystalline structure. In comparison with PS, the XRD pattern of OSA@PS was almost identical to that of PS (Fig. 4d–f), indicating that the crystalline structure was still maintained after esterification. A similar phenomenon has also been observed in the case of OSA@NS (as shown in Fig. 4a–c), suggesting that crystalline structure of starch cannot be destroyed by modification of OSA.37 No significant differences were noted between NS and PS of Xc (Table 1), meanwhile, the crystallinities of the porous starches were almost consistent with their esters.38 This indicates that the crystalline patterns of the starches were not changed by OSA esterification.32
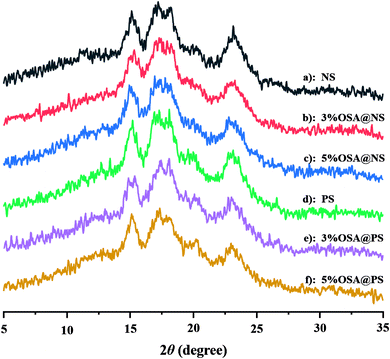 |
| Fig. 4 XRD patterns of OSA modified starches ((a) NS; (b) 3% OSA@NS; (c) 5% OSA@NS; (d) PS; (e) 3% OSA@PS; (f): 5% OSA@PS). The result indicated that the crystalline structure was still maintained after esterification. | |
3.2.4 Scanning electron microscopy. Furthermore, the pore morphology of PS and OSA@PS, with magnification of 2000 times, was eventually verified by SEM analysis before and after esterification. The SEM image of NS (Fig. 5a) showed an irregular and polygonal shape with relatively smooth surface. After esterification, the surface of starch granules becomes rougher (Fig. 5b) than NS.39 As shown in Fig. 5c, many pores and cavities were observed obviously due to the enzymatic hydrolysis. These porous structures offered more chemical reactive sites than NS, due to the larger specific surface area. It was also proved that the porous starch was more suitable for chemical modification than the native one. The SEM image of OSA@PS was displayed in Fig. 5d. Many pores and channels, with slight corrosion, were still maintained, indicating that the porous structure was not destroyed after esterification.22 The high-resolution SEM analysis with magnification of 4000 times was performed on the PS and OSA@PS, as shown in Fig. 5e and f respectively. These images showed the porous structures more clearly before and after esterification, suggesting that pores and channels were still maintained after post-treatment.
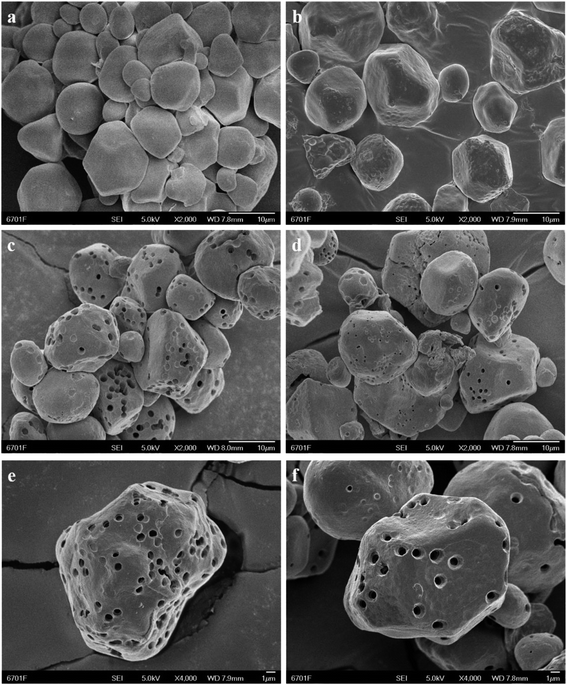 |
| Fig. 5 SEM images of NS (a) and OSA@NS (b), images of PS (c) and OSA@PS (d), images of PS with magnification of 4000 times (e) and OSA@PS with magnification of 4000 times (f). The SEM image of OSA@PS after esterification showed the undamaged pores and channels obviously, indicating that porous structure was still maintained after post-treatment. | |
N2 adsorption analysis was also utilized to explore porous structure of PS before and after esterification. The application of the Brunauer–Emmett–Teller (BET) model resulted in the surface areas of 1.08 and 1.43 m2 g−1 for PS (ESI Fig. S2†) and 5% OSA@PS (ESI Fig. S3†), respectively. In comparison with the case of PS, no difference was observed obviously indicated that pores within PS were still maintained after esterification.
3.3 Effect of modified starch on digestion characteristics of emulsion
3.3.1 Initial emulsion. Particle size distribution analysis was a significant tool for detecting the sizes of emulsions, which was expressed by the key parameter of d32. As shown in Fig. 6 and 7, the mean sizes of emulsion, stabilized by OSA modified starch, during digestion were studied by particle size distribution analysis. The particle sizes of initial emulsions showed a narrow monomodal distribution centered at about 0.75 μm (Fig. 7). Displayed in black histogram of Fig. 6, compared with the particle sizes of two OSA@NS samples, the emulsion prepared by 3% OSA@PS, with the DS of 0.026 showed the slightly smaller particle size. However, when the DS of OSA@PS was raised up to 0.0289, the particle size of emulsion became larger than that of 3% OSA@PS. This result may be derived from the greater loading amount of OSA groups would result in the larger steric hindrance of the interfacial layer.40 The confocal images (Fig. 8) also offered the evidence that the initial emulsions of OSA@NS and OSA@PS possessed the small particle sizes. Furthermore, oil droplets of initial emulsions showed the uniform distribution, with only a few amounts of large oil droplets, which is consistent with the particle size measurement above.
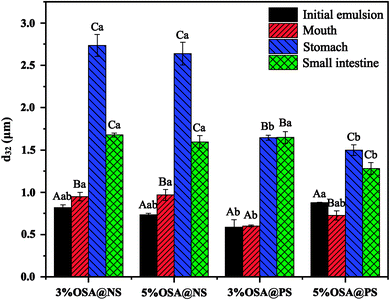 |
| Fig. 6 Particle size (d32) of emulsions containing β-carotene during in vitro digestion. Different capital letters indicate significant differences (p < 0.05) in the droplet diameter of an emulsion between digestion phases. Different lowercase letters indicate significant differences (p < 0.05) in the droplet diameter between emulsions within the same digestion phase. | |
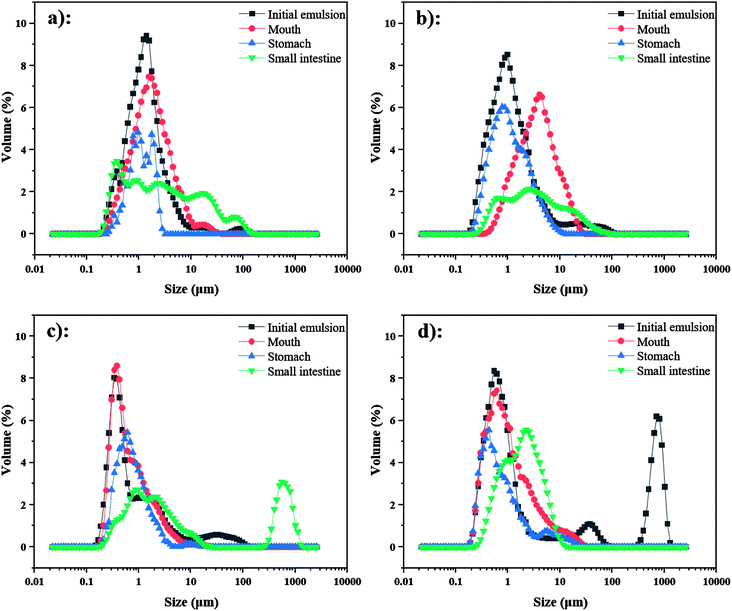 |
| Fig. 7 Particle size distribution of emulsions containing β-carotene during in vitro digestion ((a) 3% OSA@NS; (b) 5% OSA@NS; (c) 3% OSA@PS; (d) 5% OSA@PS). | |
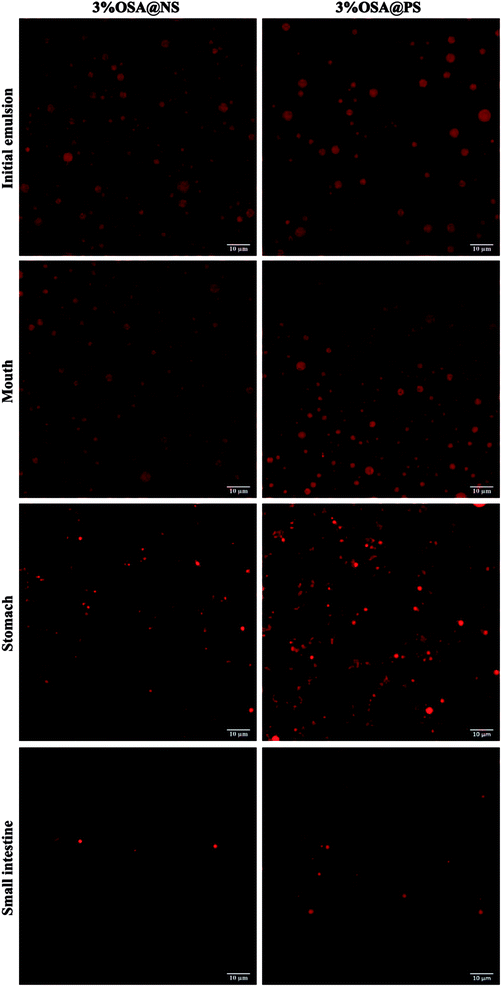 |
| Fig. 8 Changes in the microstructure (determined by CLSM) of emulsions containing β-carotene during in vitro digestion. | |
3.3.2 Mouth phase. No significant change of average particle size distributions was observed during all emulsions moved through the SSF, as shown in Fig. 6 and 7. Meanwhile, confocal microscopy images also showed that the sizes of oil droplets in the emulsion were still maintained after incubation in the mouth. The results were attributed to effect of protection, which oil droplets embedded with OSA modified starches could avoid the attack of mineral ions and α-amylase in SSF.29 OSA modified starches have the spatial repulsion generated by its branched structure, which could resist the attack of ionic strength and α-amylase.3 Moreover, the short residence time and low consumption of starch in the oral cavity would not result in a significant change in the structure of emulsions.
3.3.3 Stomach phase. The particle size distribution of the emulsions was measured after treatment of SGF, as shown in the blue histogram of Fig. 6. Compared with the SSF (seen the red histogram of Fig. 6), the particle sizes of emulsions were increased significantly after 1 h in SGF. This observation, which was consistent with the result of research,19 may result from the flocculation and coalescence occurred in the SGF.41 The variation of particle size distribution showed narrower extent in OSA@PS than that of OSA@NS. Meanwhile, as shown in the blue triangle-label of Fig. 7, in comparison to the bimodal distributions of OSA@NS (Fig. 7a and b) with the scope of 0.1–10 μm, the narrow monomodal distributions of OSA@PS was observed obviously (Fig. 7c and d) with the scope of 0.1–1 μm. This result indicated that the OSA@PS exhibited the excellent stability of the emulsions than that of NS.42As shown in ESI Fig. S4,† by means of CLSM analysis, the variation of microstructure was observed obviously in all emulsions after SGF. In the emulsions stabilized by OSA@NS, many flocs appeared along with the disappearance of small oil droplets, which indicated that severe flocculation and coalescence occurred in these emulsions.18 However, only a few flocs and large particles were observed in OSA@PS, which showed the greater stability than that of OSA@NS. This phenomenon may be explained that OSA@PS with higher DS provided a large amount of OSA groups, which not only had the larger steric hindrance, but also formed the more rigid and dense interface layer.18,43
3.3.4 Small intestine phase. The particle size distribution of the emulsions was measured after treatment of SIF for two hours. As shown in the green histogram of Fig. 6, the particle sizes of emulsions decreased slightly, in the confocal microscopy images, the sizes and amount of oil droplets in all the samples were obviously reduced, compared to that of SGF. Previous studies have already offered the evidence that the sizes and amount of oil droplets would decrease after digestion in vitro.29
3.4 Determination of bioaccessibility of β-carotene
For better absorption by intestinal epithelial cells, the fat-soluble nutrients, embedded in the carriers, need to be released from carriers firstly, and penetrated into mixed micelles or vesicles.3 Seen from the digestive pathways in the body, it was found that the amount of fat-soluble nutrients transferred from food to the micellar phase was the key parameter, which determines absorption levels of nutrients by small intestine.44 Therefore, the degree of micellization was a key parameter to characterize the bioaccessibility of fat-soluble nutrients in vitro digestion.
Many studies have proved that the OSA modified starch emulsions showed the great benefits on bioaccessibility of β-carotene. When the lipid layers were hydrolyzed gradually, more and more β-carotenes were released from the oil droplets. Meanwhile, many free fatty acids are produced during the hydrolysis of lipid, which could penetrate the mixed micelles. This could give rise to the higher solubility of β-carotenes in the mixed micelles.17,18 Besides, the higher DS of OSA modified starch may be beneficial to the stability of the emulsion in the digestive tract, which resulted in the more β-carotenes were released from oil droplets.30 Inspired by the better bioaccessibility caused by OSA modified starch, the bioaccessibility of β-carotene in the OSA@NS and OSA@PS emulsions with different DS were measured in detail, as shown in Fig. 9. It was found that availability of β-carotene enhanced along with the increasing DS of OSA modified starches, which was consistent with previous studies.18,30,45 For example, 5% OSA@PS sample have the excellent bioaccessibility, due to the DS up to 0.0289. Compared with OSA@NS samples, the bioaccessibility of OSA@PS emulsions showed almost twice than that of OSA@NS. As far as we know, there is still no report on the OSA modified porous starch. Moreover, OSA modified starch exhibited well-stabilizing effect for β-carotene. Therefore, OSA modified PS, which can obtain the higher DS than the native one, indeed was an excellent alternative for stabilize β-carotene emulsions.
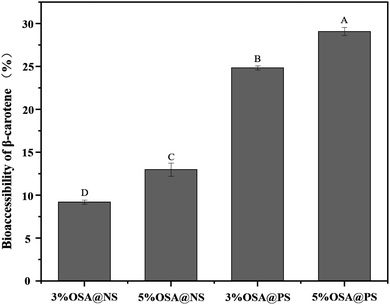 |
| Fig. 9 Bioaccessibility of β-carotene embedded in the emulsions after digestion in vitro. Different capital letters indicate a statistically significant difference (p < 0.01). | |
In summary, OSA modified porous starches with different DS were synthesized successfully by esterification reaction between porous starch and OSA groups. The spectra of 13C solid-state NMR and FT-IR spectroscopy confirmed that ester linkage of OSA modified PS was successfully formed. The XRD patterns and SEM images suggested that the ordered crystalline configuration and porous structure of starch granules were not destroyed during the OSA modified process. Using the lower consumptions of PS as materials, the higher DS of OSA@PS with the DS of 0.0289 was obtained. Furthermore, OSA@PS possessing the excellent ability of emulsification was applied as an emulsion of β-carotene for the first time. In the simulated gastric juice, we found that the emulsions with the larger DS exhibited the less flocculation and coalescence, and the stability of the emulsion was positively correlated with the DS of OSA modified starch. It was also demonstrated that different DS of samples showed the great influences on the bioaccessibility of β-carotene in the emulsion, due to the variation of stability and particle sizes of the emulsions. Therefore, as a delivery system of food-grade, OSA@PS with higher DS could be applied as emulsions for stabilizing bioactive components.
Conflicts of interest
The authors declare no competing financial interest.
Acknowledgements
This work was financially supported by the National Natural Science Foundation of China (No. 21965002), the Scientific Research Start-up Funds of Gansu Agricultural University (No. 2017RCZX-46) and Modern Agricultural Industry Technology System of Gansu Province (No. GARS-TSZ-4). The authors thank Prof. Wei Wang (Lanzhou University) for the assistance with 13C solid state NMR analysis and beneficial discussion.
References
- A. V. Rao and L. G. Rao, Pharmacol. Res., 2007, 55, 207–216 CrossRef CAS PubMed.
- Y. Yonekura and A. Nagao, Mol. Nutr. Food Res., 2007, 51, 107–115 CrossRef PubMed.
- R. Liang, C. F. Shoemaker, X. Yang, F. Zhong and Q. Huang, J. Agric. Food Chem., 2013, 61, 1249–1257 CrossRef CAS PubMed.
- C. S. Boon, D. J. McClements, J. Weiss and E. A. Decker, Crit. Rev. Food Sci. Nutr., 2010, 50, 515–532 CrossRef CAS PubMed.
- E. Meroni and V. Raikos, Food Funct., 2018, 9, 320–330 RSC.
- Q. Lin, R. Liang, F. Zhong, A. Ye, Y. Hemar, Z. Yang and H. Singh, J. Agric. Food Chem., 2019, 67, 6614–6624 CrossRef CAS PubMed.
- C. P. Tan and M. Nakajima, Food Chem., 2005, 92, 661–671 CrossRef CAS.
- Y. Yuan, Y.-X. Gao, L. Mao and J. Zhao, Food Chem., 2008, 107, 1300–1306 CrossRef CAS.
- Y. Yuan, Y.-X. Gao, J. Zhao and L. Mao, Food Res. Int., 2008, 41, 61–68 CrossRef CAS.
- D. J. McClements and J. Rao, Crit. Rev. Food Sci. Nutr., 2011, 51, 285–330 CrossRef CAS PubMed.
- B. Ozturk and D. J. McClements, Curr. Opin. Food Sci., 2016, 7, 1–6 CrossRef.
- J. Yi, Y. Li, F. Zhong and W. Yokoyama, Food Hydrocolloids, 2014, 35, 19–27 CrossRef CAS.
- T. A. J. Verrijssen, L. G. Balduyck, S. Christiaens, A. M. Van Loey, S. Van Buggenhout and M. E. Hendrickx, Food Res. Int., 2014, 57, 71–78 CrossRef CAS.
- M. C. Sweedman, M. J. Tizzotti, C. Schafer and R. G. Gilbert, Carbohydr. Polym., 2013, 92, 905–920 CrossRef CAS PubMed.
- O. Torres, B. Murray and D. A. Sarkar, Trends Food Sci. Technol., 2016, 55, 98–108 CrossRef CAS.
- J. He, J. Liu and G. Zhang, Biomacromolecules, 2008, 9, 175–184 CrossRef CAS PubMed.
- S. Zhao, G. Tian, C. Zhao, C. Lu, Y. Bao, X. Liu and J. Zheng, Food Hydrocolloids, 2018, 85, 248–256 CrossRef CAS.
- Q. Lin, R. Liang and F. Zhong, Food Hydrocolloids, 2017, 73, 184–193 CrossRef CAS.
- Q. Lin, R. Liang, F. Zhong, A. Ye and H. Singh, Food Hydrocolloids, 2018, 84, 303–312 CrossRef CAS.
- H. Ruan, Q. Chen, M. Fu, Q. Xu and G. He, Food Chem., 2009, 114, 81–86 CrossRef.
- F. Gao, D. Li, C. Bi, Z. Mao and B. Adhikari, Carbohydr. Polym., 2014, 103, 310–318 CrossRef CAS PubMed.
- X. Ma, X. Liu, D. P. Anderson and P. R. Chang, Food Chem., 2015, 181, 133–139 CrossRef CAS PubMed.
- P. R. Chang, D. Qian, D. P. Anderson and X. Ma, Carbohydr. Polym., 2012, 88, 604–608 CrossRef CAS.
- A. Dura, W. Blaszczak and C. M. Rosell, Carbohydr. Polym., 2014, 101, 837–845 CrossRef CAS PubMed.
- B. Zhang, D. Cui, M. Liu, H. Gong, Y. Huang and F. Han, Int. J. Biol. Macromol., 2012, 50, 250–256 CrossRef CAS PubMed.
- X. Song, G. He, H. Ruan and Q. Chen, Starch, 2006, 58, 109–117 CrossRef CAS.
- M. J. Tizzotti, M. C. Sweedman, D. Tang, C. Schaefer and R. G. Gilbert, J. Agric. Food Chem., 2011, 59, 6913–6919 CrossRef CAS PubMed.
- S. J. Hur, E. A. Decker and D. J. McClements, Food Chem., 2009, 114, 253–262 CrossRef CAS.
- A. Sarkar, K. Goh, R. P. Singh and H. Singh, Food Hydrocolloids, 2009, 23, 1563–1569 CrossRef CAS.
- L. Salvia-Trujillo, C. Qian, O. Martín-Belloso and D. J. McClements, Food Chem., 2013, 141, 1472–1480 CrossRef CAS PubMed.
- Y. Zheng, L. L. Hu, N. Ding, P. Liu, C. Yao and H. X. Zhang, Int. J. Biol. Macromol., 2017, 94, 566–570 CrossRef CAS PubMed.
- R. Bhosale and R. Singhal, Carbohydr. Polym., 2007, 68, 447–456 CrossRef CAS.
- F. Ye, M. Miao, C. Huang, K. Lu, B. Jiang and T. Zhang, J. Agric. Food Chem., 2014, 62, 11696–11705 CrossRef CAS PubMed.
- Y. J. Bai, Y. C. Shi, A. Herrera and O. Prakash, Carbohydr. Polym., 2011, 83, 407–413 CrossRef CAS.
- Z. Liu, Y. Li, F. Cui, L. Ping, J. Song, Y. Ravee, L. Jin, Y. Xue, J. Xu, G. Li, Y. Wang and Y. Zheng, J. Agric. Food Chem., 2008, 56, 11499–11506 CrossRef CAS PubMed.
- S. Tian, Z. Wang, X. Wang and R. Zhao, RSC Adv., 2016, 6, 96182–96189 RSC.
- W. Liu, Y. Li, M. Chen, F. Xu and F. Zhong, J. Agric. Food Chem., 2018, 66, 9301–9308 CrossRef CAS PubMed.
- G. He, X. Song, H. Ruan and F. Chen, J. Agric. Food Chem., 2006, 54, 2775–2779 CrossRef CAS PubMed.
- M. Lopez-Silvaa, L. A. Bello-Pereza, E. Agama-Acevedoa and J. Alvarez-Ramirezb, Food Hydrocolloids, 2019, 97, 105–212 CrossRef.
- J. Wang, L. Su and S. Wang, J. Sci. Food Agric., 2009, 90, 424–429 Search PubMed.
- D. J. McClements and H. Xiao, Food Funct., 2012, 3, 202–220 RSC.
- K. Królikowska, S. Pietrzyk, T. Fortuna, P. Pająk and M. Witczak, Food Chem., 2019, 278, 284–293 CrossRef PubMed.
- H. Zhang, C. Schäfer, P. Wu, B. Deng, G. Yang, E. Li, R. G. Gilbert and C. Li, Food Hydrocolloids, 2018, 74, 168–175 CrossRef CAS.
- P. Wang, H. J. Liu, X. Y. Mei, M. Nakajima and L. J. Yin, Food Hydrocolloids, 2012, 26, 427–433 CrossRef CAS.
- S. Kokubun, I. Ratcliffe and P. A. Williams, Carbohydr. Polym., 2018, 194, 18–23 CrossRef CAS PubMed.
Footnotes |
† Electronic supplementary information (ESI) available. See DOI: 10.1039/c9ra10079b |
‡ Haiyan Li and Yunxiang Ma contributed equally to this work. |
|
This journal is © The Royal Society of Chemistry 2020 |
Click here to see how this site uses Cookies. View our privacy policy here.