DOI:
10.1039/C9RA10388K
(Paper)
RSC Adv., 2020,
10, 12920-12928
Oleanolic acid administration alleviates neuropathic pain after a peripheral nerve injury by regulating microglia polarization-mediated neuroinflammation
Received
11th December 2019
, Accepted 7th March 2020
First published on 1st April 2020
Abstract
Neuropathic pain caused by a peripheral nerve injury constitutes a great challenge in clinical treatments due to the unsatisfactory efficacy of the current strategy. Microglial activation-mediated neuroinflammation is a major characteristic of neuropathic pain. Oleanolic acid is a natural triterpenoid in food and medical plants, and fulfills pleiotropic functions in inflammatory diseases. Nevertheless, its role in neuropathic pain remains poorly elucidated. In the current study, oleanolic acid dose-dependently suppressed LPS-evoked IBA-1 expression (a microglial marker) without cytotoxicity to microglia, suggesting the inhibitory efficacy of oleanolic acid in microglial activation. Moreover, oleanolic acid incubation offset LPS-induced increases in the iNOS transcript and NO releases from microglia, concomitant with the decreases in pro-inflammatory cytokine transcripts and production including IL-6, IL-1β, and TNF-α. Simultaneously, oleanolic acid shifted the microglial polarization from the M1 phenotype to the M2 phenotype upon LPS conditions by suppressing LPS-induced M1 marker CD16, CD86 transcripts, and enhancing the M2 marker Arg-1 mRNA and anti-inflammatory IL-10 levels. In addition, the LPS-induced activation of TLR4-NF-κB signaling was suppressed in the microglia after the oleanolic acid treatment. Restoring this signaling by the TLR4 plasmid transfection overturned the suppressive effects of oleanolic acid on microglial polarization-evoked inflammation. In vivo, oleanolic acid injection alleviated allodynia and hyperalgesia in SNL-induced neuropathic pain mice. Concomitantly, oleanolic acid facilitated microglial polarization to M2, accompanied by inhibition in inflammatory cytokine levels and activation of TLR4-NF-κB signaling. Collectively, these findings confirm that oleanolic acid may ameliorate neuropathic pain by promoting microglial polarization from pro-inflammatory M1 to anti-inflammatory M2 phenotype via the TLR4-NF-κB pathway, thereby indicating its usefulness as therapeutic intervention in neuropathic pain.
Introduction
Neuropathic pain is a common chronic and pathological pain that usually occurs as a direct consequence of lesion or dysfunction of the somatosensory system in peripheral and central nervous systems. Currently, neuropathic pain affects 7–10% of the general population and this prevalence is estimated to increase owing to the ageing global population worldwide.1 Patients with chronic pain often suffer from spontaneous pain that leads to a poor quality of life and heavy economic burden on the individuals and society.2,3 In addition, neuropathic pain commonly occurs in patients with other diseases such as cancer.4 Despite the advances in medicine and systematic introduction of new drugs, the currently available treatment for chronic neuropathic pain is still unsatisfactory due to the unfavorable side effects.5,6
Neuropathic pain is marked by extensive local neuroinflammation in the spinal cord based on the activation of glial cells. Microglia are prominent glial cells in the spinal cord and constitute the major immunological components of the central nervous system. In general, microglial activation is a polarized process that can be classified into two heterogeneous phenotypes: classic pro-inflammatory and cytotoxic M1 phenotype, and anti-inflammatory M2 phenotype. After a peripheral nerve damage, microglia rapidly activates and shifts into the M1 phenotype to release abundant pro-inflammatory cytokines and chemokines to generate an inflammatory environment that enhances the sensitivity of nociceptors and facilitates the initiation and maintenance of neuropathic pain.7,8 During this process, many key pathways are activated to trigger neuroinflammation, such as TLR4 signaling. Accumulating evidence corroborates the key role of aberrant TLR4 activation in the development of neuropathic pain.9,10 Currently, controlling the microglia activation-induced pro-inflammatory response has become a potential therapeutic strategy for neuroinflammatory diseases, including neuropathic pain.11,12
Oleanolic acid is a natural and ubiquitous pentacyclic triterpenoid compound (Fig. 1A) that is widely found in edible fruits, vegetables, and medicinal plants. Over the past decade, oleanolic acid has been proved to fulfill pleiotropic biological functions, including anti-carcinoma, anti-oxidant, and anti-ischemia/reperfusion injury.13,14 Moreover, oleanolic acid can act as a potential therapeutic drug for chronic diseases.15 Recently emerging research has implicated oleanolic acid in inflammation associated diseases.16,17 For instance, administration of oleanolic acid alleviates ovalbumin-induced airway inflammation and allergic asthma.16 Intriguingly, oleanolic acid treatment attenuates mustard oil-induced colonic nociception in mice.18 In addition, oleanolic acid notably ameliorates nociceptive behavior associated with pain in orofacial nociception in zebrafish.19 However, the function of oleanolic acid in chronic neuropathic pain is still undefined.
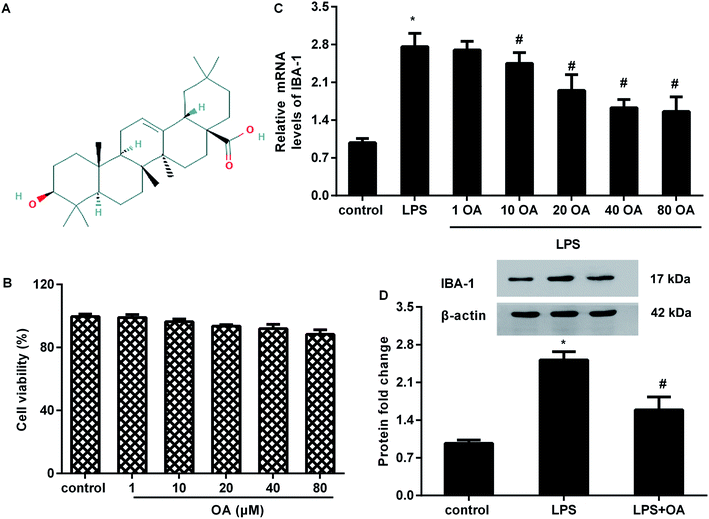 |
| Fig. 1 Effects of oleanolic acid on microglial activation upon LPS exposure. (A) Biochemical structure and spatial conformation of oleanolic acid. (B) BV2 microglia cells were treated with oleanolic acid (OA) ranging from 1 μM to 80 μM for 24 h. Cell viability was then evaluated by MTT assay. (C) Cells were administrated with various doses of oleanolic acid, prior to LPS exposure for 12 h. Then, the mRNA levels of IBA-1 were determined by qRT-PCR. (D) After stimulation with oleanolic acid (40 μM) and/or LPS for 12 h, the corresponding effects on the protein expression of IBA-1 were detected. *P < 0.05 vs. control group, #P < 0.05 vs. LPS group. | |
In the current study, we sought to elucidate the function of oleanolic acid on lipopolysaccharide (LPS)-induced microglial inflammation and spinal nerve ligation (SNL)-mimicked neuropathic pain in mice. In addition, this study also deciphered the underlying molecular mechanism during these processes.
Materials and methods
Animals and ethics statement
Experiments were carried out on male wild type C57BL/6 mice (10–12 weeks old, weight 20–25 g). All mice were bought from the Center of Laboratory Animals, the Fourth Military Medical University of China. Before experiments, the mice were housed at standard laboratory conditions (constant temperature of 22 ± 1 °C with 12 h light–dark cycles) and had ad libitum access to food and water. All animal procedures were conducted in accordance with the National Institutes of Health (NIH) Guide for the Care and Use of Laboratory Animals, and approved by the Institutional Animal Care and Use Committee of China-Japan union hospital of Jilin university. All efforts were made to minimize animal suffering during the experimental procedures.
Neuropathic pain model construction and drug administration
Spinal nerve ligation (SNL) surgery was performed to induce neuropathic pain model as previously reported.20 Briefly, the mice were anesthetized with sodium pentobarbital and barbered along the back with skin sterilization by chlorhexidine. After removal of L6 transverse process to expose the ventral ramus of the L4 and L5 spinal nerves, the L5 spinal nerve was identified and tightly ligated with 6-0 silk threads without damage to the other nerves. Mice were then randomly divided into 5 groups (n = 10 in each group). In the control group, the left L5 spinal nerve was isolated without ligation. Group 2 was conducted with SNL treatment. The other groups served as SNL + oleanolic acid (OA, >97% purity) (Sigma, St. Louis, MO, USA) groups wherein the mice were separately injected (i.p.) with 2 mg kg−1, 5 mg kg−1, and 10 mg kg−1 of oleanolic acid after SNL surgery for 5 consecutive days.
Behavior tests for tactile allodynia and heat hyperalgesia
For the pain behavior test, tactile allodynia was assessed in mice subjected to SNL using a series of von Frey hairs (Stoelting, Kiel, WI, USA).21 Mice were habituated to the testing environment for 2 days before SNL to measure pain thresholds (baseline). Before the experiment, mice were acclimated for 1 h inside a Plexiglas box on a steel mesh floor. Then, a series of von Frey filaments with logarithmically increasing stiffness (0.02–2.56 g) were perpendicularly applied to stimulate the plantar surface of the hindpaw with 3–5 s for each group. The minimal value that caused at least 3 responses of paw withdrawal was recorded as the paw withdrawal threshold (PWT).
To evaluate heat hyperalgesia, mice from various groups were put in glass boxes and allowed to adapt for 1 h before the tests. Afterwards, heat sensitivity of mice hind paws was measured using a radiant heat apparatus (390G Plantar Test Apparatus, IITC Life Science Inc., Woodland Hills, CA, USA) through the detection of paw withdrawal latency (PWL). During this process, the radiant heat intensity was adjusted to approximately 12 s with a cutoff of 20 s to avert tissue damage.
Cell culture
Murine BV2 microglial cell line was bought from the Institute of Cell Biology, Chinese Academy of Sciences (Shanghai, China). For culture, the cells were incubated in Dulbecco's modified Eagle medium (DMEM; Invitrogen, CA, USA) supplemented with 10% fetal bovine serum (Thermo Fisher Scientific, Waltham, MA, USA) and 1% penicillin/streptomycin. All the cells were maintained at 37 °C with an atmosphere of 5% CO2.
Cell treatment
In vitro, BV2 cells were pre-administrated with various concentrations of oleanolic acid (OA, >97% purity) (1, 10, 20, 40, 80 μM) for 10 h. Subsequently, 1 μg mL−1 of LPS was supplemented for further incubation for 12 h.
MTT assay for cell viability
Cell viability was evaluated by the MTT assay as previously described.22–24 Cells were seeded in 96-well plates and were then exposed to the indicated doses of oleanolic acid for 24 h. Then, the cells were maintained in DMEM medium containing 100 μL of MTT reagent (5 mg mL−1, Sigma, St. Louis, MO, USA). Approximately 4 h later, 100 μL of dimethyl sulfoxide solution was supplemented to dissolve the crystal formazan. The absorbance at 570 nm was captured using a microplate reader (Bio-Rad, Hercules, CA, USA) to analyze the cell viability.
Recombinant vector construction and transfection
To overexpress TLR4 in BV2 cells, mice TLR4 cDNA was amplified using PCR. Then, the prepared TLR4 cDNA was subcloned into the pcDNA3.1(+) plasmid (Invitrogen) to synthesize the recombinant pcDNA3.1-TLR4 plasmids. Subsequently, transfection was conducted as previously reported.25 Briefly, the BV2 cells were transfected with the pcDNA3.1-TLR4 vectors using Lipofectamine 2000 reagent (Invitrogen). The cells that transfected with the empty vectors were defined as the negative control. The transfection efficacy was then evaluated by western blotting.
Quantitative RT-PCR
L4-5 spinal segmental tissues were collected from mice after deep anesthetization with sodium pentobarbital (100 mg kg−1 i.p.). Then, total RNA isolation from the tissues and cells was prepared using the TRIzol reagent (Invitrogen). Subsequently, reverse transcription was performed to synthesize cDNA using the High-Capacity cDNA Reverse Transcription Kits (Applied Biosystems, Foster City, CA, USA). Then, qRT-PCR was conducted as reported previously25 to determine the transcripts of iNOS, IBA-1, IL-6, IL-1β, TNF-α, CD16, CD86, and arginase-1 (Arg-1) using a SYBR® Premix Ex Taq™ II Kit (Takara, Otsu, Japan). All the procedures were performed according to the manufacturers' protocols and reacted on an Applied Biosystems 7300 Real-Time PCR System (Applied Biosystems; Foster City, CA, USA). The specific primers for these genes are shown in Table 1. β-Actin served as an internal reference to quantify the target gene expression; all the results were calculated by using 2−ΔΔCt.
Table 1 Primer sequences of qRT-PCR
Name |
Primer sequences (5′–3′) |
iNOS |
Sense, 5′-GGAATCTTGGAGCGAGTTGT-3′ |
Anti-sense, 5′-CCTCTTGTCTTTGACCCAGTAG-3′ |
IBA-1 |
Sense, 5′-GACGTTCAGCTACTCTGACTTT-3′ |
Anti-sense, 5′-GTTGGCCTCTTGTGTTCTTTG-3′ |
IL-6 |
Sense, 5′-ATTCCTCTGTGCCACCTTTAC-3′ |
Anti-sense, 5′-GGTCAGCACCACCATCTTATT-3′ |
IL-1β |
Sense, 5′-ATGGGCAACCACTTACCTATTT-3′ |
Anti-sense, 5′-GTTCTAGAGAGTGCTGCCTAATG-3′ |
TNF-α |
Sense, 5′-TTGCTCTGTGAAGGGAATGG-3′ |
Anti-sense, 5′-GGCTCTGAGGAGTAGACAATAAAG-3′ |
CD16 |
Sense, 5′-TGGACACGGGCCTTTATTTC-3′ |
Anti-sense, 5′-GAGCCTGGTGCTTTCTGATT-3′ |
CD86 |
Sense, 5′-CCTGGAAAGGTCTGGAGAATG-3′ |
Anti-sense, 5′-GGCAGATCAGTCCTTCCATAAA-3′ |
Arg-1 |
Sense, 5′-ACAGCAAAGCAGACAGAACTA-3′ |
Anti-sense, 5′-GAAAGGAACTGCTGGGATACA-3′ |
β-Actin |
Sense, 5′-TCTAGACTTCGAGCAGGAGATG-3′ |
Anti-sense, 5′-GAACCGCTCGTTGCCAATA-3′ |
Measurement of nitric oxide (NO) concentration
Microglia were treated with the indicated doses of oleanolic acid prior to LPS exposure. Then, the cells were reacted with Griess reagent (Nanjing Jiancheng Bioengineering Institute, Nanjing, China) for 10 min. The absorbance at 550 nm was captured to evaluate the contents of NO from the cells by referring to a freshly prepared nitrite standard curve.
ELISA assay for inflammatory cytokine levels
ELISA assay was performed to determine the cytokine levels as previously described.23,26 The concentrations of IL-6, IL-1β, TNF-α, and IL-10 in the supernatant from BV2 cells and spinal cord tissue homogenate were analyzed according to the instructions of the commercially available ELISA kits (Invitrogen).
Western blotting
Western blotting was performed as previously described.24,26 Briefly, total protein concentration from the cells and L4–L5 spinal segments was extracted by homogenizing in a RIPA buffer (Thermo Fisher Scientific). The BCA protein assay kit (Beyotime, Shanghai, China) was applied to quantify the protein concentration. Then, equal amounts of protein were loaded in each lane and separated by 12% SDS-PAGE. After transfer to the PVDF membrane, 5% non-fat milk was added to interdict non-specific binding for 2 h. For immunoblotting, the membrane was incubated with primary antibodies against mice IBA-1 (1
:
1500), TLR4 (1
:
1000), p-p65 NF-κB (1
:
5000), and p65 NF-κB (1
:
1000) (all from Abcam, Cambridge, MA, USA) overnight at 4 °C. After incubation with horseradish peroxidase-conjugated secondary antibody, the immunoreactive proteins were visualized by ECL reagent (Beyotime) and quantified by ImageJ software (Bethesda, MD, USA).
Statistical analysis
All the data were obtained from at least three separate experiments and analyzed by the SPSS19.0 software. The values are given as mean ± SD. Differences between the groups were compared using Student's t-test for two groups and ANOVA, followed by the SNK post hoc test for three or more groups. The criterion for statistical significance was P < 0.05.
Results
Treatment with oleanolic acid restrains microglial activation upon LPS conditions without cytotoxicity
To evaluate the potential cytotoxicity of oleanolic acid, microglial cells were exposed to various doses of oleanolic acid for 24 h. MTT assay confirmed that oleanolic acid ranging from 1 to 80 μM concentration showed little cytotoxicity to microglia (Fig. 1B). Moreover, oleanolic acid dose-dependently suppressed the mRNA levels of microglia marker IBA-1 in microglia exposed to LPS and reached its climax at 40 μM-treated groups; however, there was no difference between the 40 μM- and 80 μM-treated groups (Fig. 1C). Simultaneously, administration with 40 μM of oleanolic acid inhibited LPS-induced protein expression of IBA-1 in the microglia (Fig. 1D).
Oleanolic acid suppresses inflammatory response in LPS-activated BV2 cells
Accumulating evidence supports the critical role of microglial activation-mediated neuroinflammation in neuropathic pain. We, therefore, investigated the function of oleanolic acid in microglial inflammation in vitro and the results corroborated that LPS exposure increased the transcript of iNOS in BV2 microglial cells, which was inhibited by oleanolic acid in a dose-dependent manner (Fig. 2A). Concomitantly, oleanolic acid also restrained LPS-induced release of inflammatory mediator NO (Fig. 2B). Furthermore, LPS-activated microglia presented high mRNA levels of pro-inflammatory cytokine IL-6 (Fig. 2C), IL-1β (Fig. 2D), and TNF-α (Fig. 2E), and these increases were suppressed following pretreatment with 40 μM of oleanolic acid. Importantly, oleanolic acid reduced LPS-induced production of IL-6 (Fig. 2F), IL-1β (Fig. 2G), and TNF-α (Fig. 2H) from the microglia.
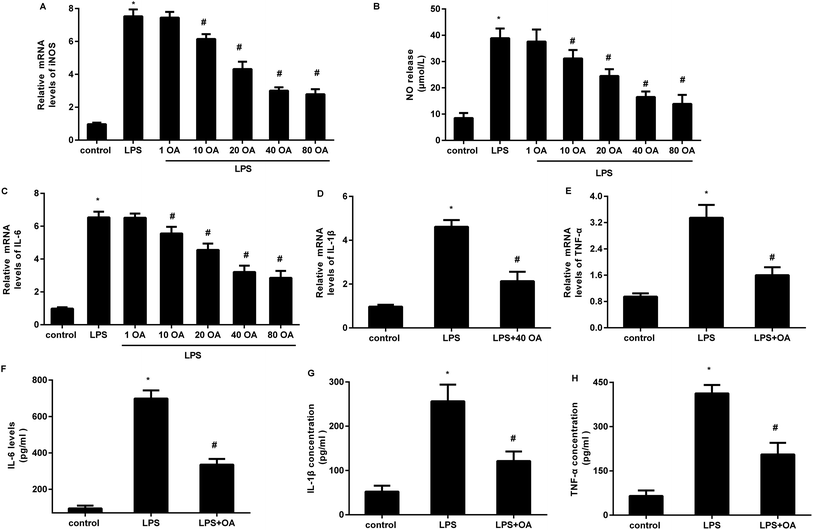 |
| Fig. 2 Oleanolic acid suppressed microglial inflammatory response under LPS conditions. (A) After treatment with various doses of oleanolic acid, cells were exposed to LPS. Then, the mRNA levels of iNOS were analyzed. (B) The contents of NO production were detected. (C) The subsequent effects on the mRNA levels of IL-6 were determined. (D and E) Cells treated with 40 μM of oleanolic acid were stimulated with LPS for 12 h. Then, qRT-PCR assay was performed to detect the mRNA levels of IL-1β (D) and TNF-α (E). (F–H) ELISA analysis was conducted to measure the concentrations of IL-6 (F), IL-1β (G), and TNF-α (H) in supernatants from microglia under oleanolic acid and/or LPS conditions. *P < 0.05 vs. control group, #P < 0.05 vs. LPS group. | |
Oleanolic acid shifts microglial polarization from M1 to M2 phenotype in LPS-stimulated BV2 cells
Microglia adopt two distinct activation phenotypes: a classical neurotoxic M1 phenotype and a neuroprotective anti-inflammatory M2 phenotype. Therefore, we next explored whether oleanolic acid effects microglial polarization. As presented in Fig. 3A and B, LPS stimulation elevated the transcripts of CD16 (Fig. 3A) and CD86 (Fig. 3B), the markers of M1 microglial phenotype. Intriguingly, oleanolic acid pretreatment notably antagonized LPS-induced increases in CD16 and CD86 mRNA levels. Concomitantly, LPS exposure suppressed the transcript of Arg-1, a sensitive marker of M2 microglial phenotype (Fig. 3C). However, oleanolic acid enhanced the expression of Arg-1 (Fig. 3C) and anti-inflammatory IL-10 levels (Fig. 3D) relative to the LPS-treated groups.
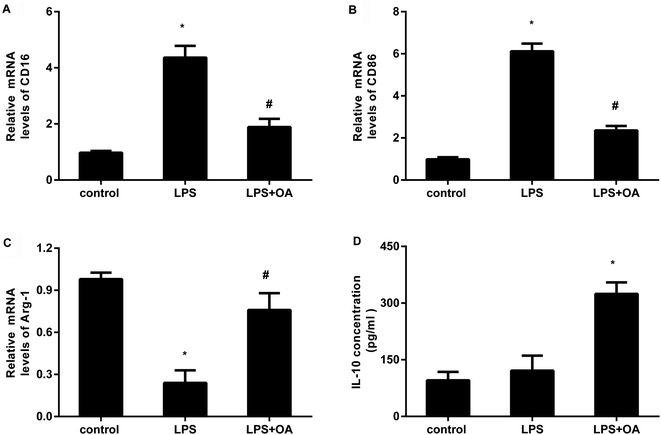 |
| Fig. 3 Treatment with oleanolic acid affected microglial polarization. (A and B) After pretreatment with oleanolic acid for 10 h, cells were exposed to LPS. Then, the mRNA levels of M1 phenotype marker CD16 (A) and CD86 (B) were determined by qRT-PCR. (C and D) The subsequent effects on the M2 phenotype marker Arg-1 transcript (C) and IL-10 concentration (D) were assessed. *P < 0.05, #P < 0.05. | |
TLR4-NF-κB signaling is involved in the protective efficacy of oleanolic acid against microglial polarization-mediated inflammation
The aberrant activation of TLR4-NF-κB signaling is involved in pain and inflammation-related diseases, including neuropathic pain. Herein, LPS treatment increased the protein expression of TLR4 and p-p65 NF-κB in the microglia (Fig. 4A and B). Nevertheless, these increases were inhibited after oleanolic acid administration, indicating the suppressive effect of oleanolic acid on LPS-evoked activation of the TLR4-NF-κB pathway in microglia. Notably, transfection with recombinant TLR4 (rTLR4) vector dramatically elevated the expression of TLR4 and p-p65 NF-κB (Fig. 4C and D). Intriguingly, reactivating TLR4 signaling by rTLR4 transfection reversed the inhibitory effects of oleanolic acid against LPS-induced M1 phenotype marker CD16 (Fig. 4E) and CD86 (Fig. 4F). However, oleanolic acid-increased the M2 phenotype marker Arg-1 mRNA, which was overturned after restoring the TLR4 pathway in LPS-treated microglia (Fig. 4G). Simultaneously, the increased levels of IL-6 (Fig. 4H), IL-1β (Fig. 4I), and TNF-α (Fig. 4J) from the microglia upon LPS treatment were attenuated by oleanolic acid administration, which was reversed following rTLR4 transfection.
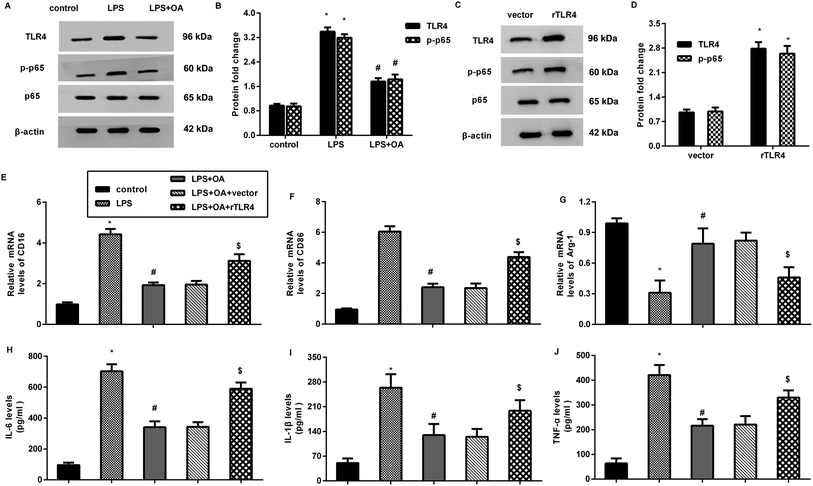 |
| Fig. 4 Activation of the TLR4-NF-κB pathway was involved in oleanolic acid-mediated microglial inflammation. (A and B) The protein expression levels of TLR4 and p-p65 NF-κB were analyzed in microglia exposed to oleanolic acid and LPS. (C and D) Cells were transfected with recombinant TLR4 vectors and the subsequent protein levels of TLR4 and p-p65 NF-κB were determined. (E–G) The mRNA levels of CD16 (E), CD86 (F), and Arg-1 (G) were analyzed by qRT-PCR. (H–J) ELISA assay was carried out to evaluate the releases of IL-6 (H), IL-1β (I), and TNF-α (J) in the supernatants. *P < 0.05 vs. control group, #P < 0.05 vs. LPS group, $P < 0.05 vs. LPS + OA group. | |
Oleanolic acid exerts anti-nociceptive effects against SNL-induced neuropathic pain
To further elucidate the therapeutic potential of oleanolic acid in neuropathic pain in vivo, SNL was performed to construct the neuropathic pain model. As shown in Fig. 5A, mice noticeably decreased the paw withdrawal threshold at days 1, 3, 5, and 7 after SNL, which was dose-dependently offset after oleanolic acid treatment. In addition, administration with oleanolic acid also antagonized SNL-induced down-regulation of thermal pain thresholds of paw withdrawal latency (Fig. 5B). Thus, these findings indicate the beneficial efficacy of oleanolic acid against neuropathic pain.
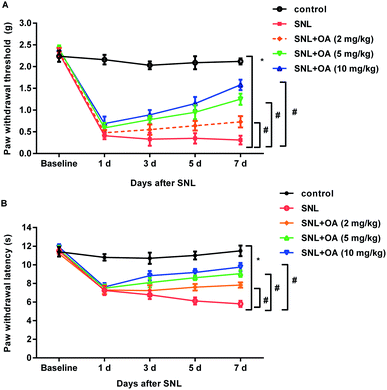 |
| Fig. 5 Injection with oleanolic acid ameliorated allodynia and hyperalgesia in SNL-mimicked neuropathic pain models. (A) Mice were administrated with oleanolic acid (OA; 2, 5, and 10 mg kg−1) after SNL for 5 consecutive days. The mechanical allodynia on 1, 3, 5, and 7 d post-SNL was evaluated by Von Frey test. (B) The heat hyperalgesia was also performed to assess the pain behavior. *P < 0.05 vs. control group, #P < 0.05 vs. SNL group. | |
Oleanolic acid suppresses microglial activation-mediated neuroinflammation in mice after SNL treatment
Further analysis confirmed that oleanolic acid suppressed the expression of IBA-1 in the spinal cord at 7 days after SNL, indicating the inhibitory effects of oleanolic acid on microglial activation (Fig. 6A). Moreover, oleanolic acid injection inhibited SNL-increased expression of CD86 (Fig. 6B) but increased the expression of Arg-1 (Fig. 6C) in neuropathic pain mice induced by SNL surgery. Simultaneously, SNL increased the concentrations of M1 phenotype pro-inflammatory cytokine IL-6 (Fig. 6D), IL-1β (Fig. 6E), and TNF-α (Fig. 6F), which was counteracted after oleanolic acid treatment. Concomitantly, injection with oleanolic acid enhanced the production of M2 phenotype anti-inflammatory cytokine IL-10 in SNL-induced mice model (Fig. 6G).
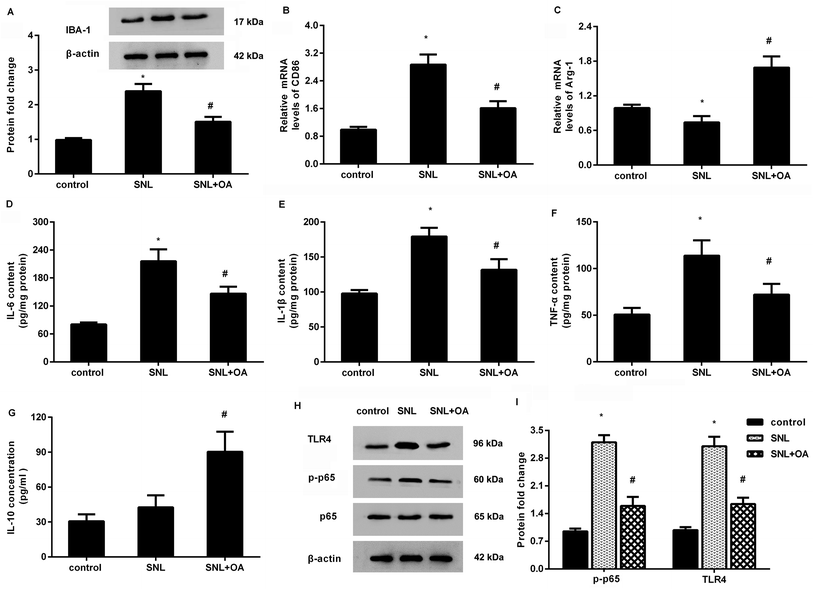 |
| Fig. 6 Oleanolic acid administration alleviated neuroinflammation and microglial polarization in mice. (A) Mice were injected with the indicated doses of oleanolic acid starting from 1 h after SNL surgery. The protein levels of microglial marker IBA-1 were analyzed in spinal cord tissues of mice at 7 d post-SNL. (B and C) The mRNA levels of CD86 (B) and Arg-1 (C) were detected by qRT-PCR. (D–I) The subsequent effects on IL-6 (D), IL-1β (E), TNF-α (F), and IL-10 (G) concentrations, TLR4 pathway activation (H and I) were also measured. *P < 0.05 vs. control group, #P < 0.05 vs. SNL group. | |
Oleanolic acid mutes the activation of TLR4-NF-κB pathway in SNL-induced models
As shown in Fig. 6H, SNL-induced mice models exhibited higher protein expression of TLR4 and p-p65 NF-κB relative to the control groups. Notably, oleanolic acid administration noticeably restrained the activation of this pathway by decreasing the expression of TLR4 and p-p65 NF-κB in mice after SNL surgery.
Discussion
Injury to the peripheral nerve usually gives rise to intolerable neuropathic pain that leads to persistent and spontaneous pain in patients. Notably, gaining favorable management of neuropathic pain remains a great challenge in clinical treatments due to the unsatisfactory efficacy of the current strategy, such as non-steroidal anti-inflammatory drugs.6 In the current study, oleanolic acid, a biologically active triterpenoid, shifted LPS-induced microglial polarization from M1 type to M2 phenotype and therefore, the attenuated microglial activation-induced inflammation by suppressing the TLR4-NF-κB signaling. Notably, injection with oleanolic acid alleviated SNL-induced neuroinflammation as well as mechanical and heat hypersensitivity in mice. Thus, the current research highlights oleanolic acid as a promising therapeutic avenue for neuropathic pain after peripheral nerve injury.
Neuropathic pain after nerve injury is characterized by neuroinflammation after glial cell activation in the corresponding spinal cords. Microglia are the major immune effectors of glial cells in the central nervous system and can adopt two distinct activation phenotypes: classical M1 phenotype (pro-inflammation) and alternative M2 phenotype (anti-inflammation). Convincing evidences have confirmed that microglia play key roles in the pathogenesis of neuropathic pain.7,12 To decipher the function of oleanolic acid in neuropathic pain, we investigated its effects on microglia activation-induced neuroinflammation. Analogous to previous reports,27 LPS exposure enhanced microglial activation by increasing its marker IBA-1 expression. Noticeably, oleanolic acid treatment engendered not only little cytotoxicity but also suppressed LPS-induced microglial activation.
Alterations in microglia M1/M2 polarization have been implicated in neurodegenerative diseases, including neuropathic pain. In adolescence, microglia can polarize to generate M1 phenotype to release abundant pro-inflammatory cytokines, which is concomitant with the emergence of neuropathic pain.7 Intriguingly, facilitating phenotypic transformation from M1 phenotype to M2 phenotype has become a subject of interest based on the potential therapeutic value against neuroinflammation-related diseases.12 In the current study, LPS stimuli facilitated microglial polarization towards M1 phenotype and inhibited M2 polarization as the evidence that LPS increased M1 type marker CD16 and CD86 levels, and reduced the expression of M2 marker Arg-1. Intriguingly, oleanolic acid administration shifted LPS-induced M1 polarization towards M2 polarization in the microglia. Similar to a previous study, LPS treatment increased the production of pro-inflammatory cytokines IL-6, IL-1β, and TNF-α but inhibited the release of anti-inflammatory IL-10 levels in the microglia.28 Importantly, oleanolic acid restrained LPS-induced pro-inflammatory cytokine expression, whereas it increased the IL-10 levels. Thus, these findings suggest that oleanolic acid may attenuate microglial activation-induced neuroinflammation by regulating LPS-triggered microglial phenotype transformation from M1 to M2 polarization.
Mechanistically, this research corroborated that LPS exposure activated the pathway of TLR4-NF-κB in the microglia. Acceptably, LPS can engage with its receptor TLR4 on the microglia and subsequently induces excessive secretion of pro-inflammatory cytokines, such as IL-1β. An increasing amount of research has implicated TLR4-NF-κB signaling in the pathogenic progression of inflammation-related diseases, including inflammatory and neuropathic pain.9 Intriguingly, emerging studies confirm the aberrant activation of TLR4 pathway in rat model of chronic neuropathic pain.9,10 We therefore investigated the involvement of TLR4 signaling in oleanolic acid-inhibited microglial inflammation. As expected, oleanolic acid treatment inhibited LPS-induced activation of TLR4-NF-κB signaling in the microglia. More importantly, restoring this pathway overturned the oleanolic acid-mediated shift of microglial polarization from M1 towards the M2 phenotype, resulting in the enhancement of microglia activation-induced inflammation. Analogously, the activation of TLR4-NF-κB signaling induces M1 microglial polarization and release of pro-inflammatory molecules.29,30
To further decipher the function of oleanolic acid in neuropathic pain in vivo, spinal nerve ligation (SNL) surgery was applied to construct the neuropathic pain model.20 It was remarkable that injection with oleanolic acid alleviated allodynia and hyperalgesia in SNL-mimicked mice models. Notably, oleanolic acid administration elevated M2 phenotype marker Arg-1 expression and decreased M1 phenotype marker CD86 expression in the spinal cords of neuropathic pain mice, concomitant with the decreases in pro-inflammatory IL-6, IL-1β, and TNF-α and increases in the anti-inflammatory M2 phenotype cytokine IL-10. These results indicate that oleanolic acid may attenuate pain in the neuropathy model by regulating microglial polarization-mediated neuroinflammation. Intriguingly, promoting microglia polarization towards M2 phenotype relieves pain behavior in the rat model of neuropathy.8 In addition, the activation of TLR4-NF-κB pathway in SNL-induced mice models was also restrained following oleanolic acid administration. Noticeably, the activation of the TLR4-NF-κB pathway in spinal cord results in chronic morphine-induced analgesic tolerance and hyperalgesia in rats.31 Nevertheless, the blockage of TLR4 exerts analgesic properties by restoring the balance between nociceptive factors in neuropathy development.32
Conclusions
In conclusion, one noteworthy observation in the current study was that oleanolic acid shifted microglial activation from M1 phenotype to M2 phenotype and suppressed the subsequent inflammatory response in LPS-treated microglia by blocking the TLR4-NF-κB pathway. Noticeably, administration with oleanolic acid ameliorated the pain behavior and microglial polarization-mediated neuroinflammation, concomitant with the suppression of the TLR4-NF-κB signaling. Thus, the current study may endorse the potential usefulness of oleanolic acid as a therapeutic agent against chronic neuropathic pain based on its anti-nociceptive and anti-inflammatory efficacy. Oleanolic acid is an intracellular or extracellular intervention. However, TLR4 is a known transmembrane protein and can be activated by HMGB1.33,34 How does oleanolic acid regulate TLR4 signaling? Increasing evidence corroborates the critical role of HMGB1 in the development of neuropathic pain.34 A previous study found that oleanolic acid pretreatment alleviated hepatic ischemia reperfusion by decreasing HMGB1 release.14 Does oleanolic acid ameliorate neuropathic pain by regulating TLR4 signaling via HMGB1? This question will be explored in out next study.
Data availability statement
All data generated or analyzed during this study are included in this published article.
Compliance with ethical standards
The experiments involving animals were performed according to the National Institutes of Health Guide for the Care and Use of Laboratory Animals.
Funding
This research did not receive any specific grant from funding agencies in the public, commercial, or not-for-profit sectors.
Conflicts of interest
There are no conflicts to declare.
References
- L. Colloca, T. Ludman, D. Bouhassira, R. Baron, A. H. Dickenson, D. Yarnitsky, R. Freeman, A. Truini, N. Attal, N. B. Finnerup, C. Eccleston, E. Kalso, D. L. Bennett, R. H. Dworkin and S. N. Raja, Nat. Rev. Dis. Primers, 2017, 3, 17002 CrossRef PubMed.
- A. Bedini and S. Spampinato, Curr. Med. Chem., 2018, 25, 3895–3916 CrossRef PubMed.
- R. S. Rasu, K. Vouthy, A. N. Crowl, A. E. Stegeman, B. Fikru, W. A. Bawa and M. E. Knell, J. Manag. Care Spec. Pharm., 2014, 20, 921–928 Search PubMed.
- A. Oosterling, N. te Boveldt, C. Verhagen, W. T. van der Graaf, M. Van Ham, M. Van der Drift, K. Vissers and Y. Engels, Pain Pract., 2016, 16, 413–421 CrossRef PubMed.
- P. Cvrcek, Pain Med., 2008, 9, 253–257 CrossRef PubMed.
- A. Binder and R. Baron, Dtsch Arztebl Int., 2016, 113, 616–625 Search PubMed.
- X. Gong, Y. Chen, B. Fu, J. Jiang and M. Zhang, Neuroscience, 2017, 349, 76–86 CrossRef CAS PubMed.
- K. Popiolek-Barczyk, N. Kolosowska, A. Piotrowska, W. Makuch, E. Rojewska, A. M. Jurga, D. Pilat and J. Mika, Neural Plast., 2015, 2015, 676473 Search PubMed.
- L. Xu, Y. Liu, Y. Sun, H. Li, W. Mi and Y. Jiang, Biomed. Pharmacother., 2018, 107, 526–533 CrossRef CAS PubMed.
- Y. Li, C. Yin, X. Li, B. Liu, J. Wang, X. Zheng, X. Shao, Y. Liang, J. Du, J. Fang and B. Liu, Int. J. Mol. Sci., 2019, 20, 5917 CrossRef PubMed.
- G. L. Jin, S. D. He, S. M. Lin, L. M. Hong, W. Q. Chen, Y. Xu, J. Yang, S. P. Li and C. X. Yu, Neural Plast., 2018, 2018, 9347696 Search PubMed.
- A. Piotrowska, K. Kwiatkowski, E. Rojewska, W. Makuch and J. Mika, Neuropharmacology, 2016, 108, 207–219 CrossRef CAS PubMed.
- I. Potocnjak, L. Simic, I. Vukelic and R. Domitrovic, Food Chem. Toxicol., 2019, 132, 110676 CrossRef CAS PubMed.
- W. Wang, L. Wu, J. Li, J. Ji, K. Chen, Q. Yu, S. Li, J. Feng, T. Liu, J. Zhang, J. Chen, Y. Zhou, Y. Mao, F. Wang, W. Dai, X. Fan, C. Guo and J. Wu, Mediators Inflammation, 2019, 2019, 3240713 Search PubMed.
- T. B. Ayeleso, M. G. Matumba and E. Mukwevho, Molecules, 2017, 22, 1915 CrossRef PubMed.
- S. H. Kim, J. H. Hong and Y. C. Lee, Int. Immunopharmacol., 2014, 18, 311–324 CrossRef PubMed.
- D. Kashyap, A. Sharma, H. S. Tuli, S. Punia and A. K. Sharma, Recent Pat. Inflammation Allergy Drug Discovery, 2016, 10, 21–33 CrossRef PubMed.
- J. L. Maia, R. C. Lima-Junior, J. P. David, J. M. David, F. A. Santos and V. S. Rao, Biol. Pharm. Bull., 2006, 29, 82–85 CrossRef PubMed.
- I. C. R. Soares, S. Santos, R. F. Coelho, Y. A. Alves, A. E. Vieira-Neto, K. C. S. Tavares, F. E. A. Magalhaes and A. R. Campos, Chem.-Biol. Interact., 2019, 299, 37–43 CrossRef PubMed.
- Y. Lu, B. C. Jiang, D. L. Cao, Z. J. Zhang, X. Zhang, R. R. Ji and Y. J. Gao, Pain, 2014, 155, 2618–2629 CrossRef PubMed.
- B. C. Jiang, W. X. Sun, L. N. He, D. L. Cao, Z. J. Zhang and Y. J. Gao, Mol. Pain, 2015, 11, 43 CrossRef PubMed.
- K. F. Zhai, H. Duan, C. Y. Cui, Y. Y. Cao, J. L. Si, H. J. Yang, Y. C. Wang, W. G. Cao, G. Z. Gao and Z. J. Wei, J. Agric. Food Chem., 2019, 67, 2856–2864 CrossRef PubMed.
- K. F. Zhai, H. Duan, G. J. Khan, H. Xu, F. K. Han, W. G. Cao, G. Z. Gao, L. L. Shan and Z. J. Wei, J. Agric. Food Chem., 2018, 66, 6073–6082 CrossRef PubMed.
- K. F. Zhai, H. Duan, Y. Chen, G. J. Khan, W. G. Cao, G. Z. Gao, L. L. Shan and Z. J. Wei, Food Funct., 2018, 9, 2070–2079 RSC.
- K. F. Zhai, J. R. Zheng, Y. M. Tang, F. Li, Y. N. Lv, Y. Y. Zhang, Z. Gao, J. Qi, B. Y. Yu and J. P. Kou, Br. J. Pharmacol., 2017, 174, 2818–2831 CrossRef PubMed.
- K. F. Zhai, H. Duan, L. Luo, W. G. Cao, F. K. Han, L. L. Shan and X. M. Fang, Inflammopharmacology, 2017, 25, 523–532 CrossRef PubMed.
- Y. Zheng, X. Hou and S. Yang, Cell. Mol. Neurobiol., 2019, 39, 1081–1092 CrossRef PubMed.
- M. D. Zhu, L. X. Zhao, X. T. Wang, Y. J. Gao and Z. J. Zhang, Brain Res. Bull., 2014, 109, 54–60 CrossRef PubMed.
- M. Zusso, V. Lunardi, D. Franceschini, A. Pagetta, R. Lo, S. Stifani, A. C. Frigo, P. Giusti and S. Moro, J. Neuroinflammation, 2019, 16, 148 CrossRef PubMed.
- M. Huang, Y. Li, K. Wu, W. Yan, T. Tian, Y. Wang and H. Yang, Chem.-Biol. Interact., 2019, 310, 108743 CrossRef CAS PubMed.
- L. Bai, C. Zhai, K. Han, Z. Li, J. Qian, Y. Jing, W. Zhang and J. T. Xu, Neurosci. Bull., 2014, 30, 936–948 CrossRef CAS PubMed.
- A. M. Jurga, E. Rojewska, W. Makuch and J. Mika, Pharm. Biol., 2018, 56, 275–286 CrossRef CAS PubMed.
- N. Morioka, K. Miyauchi, K. Miyashita, T. Kochi, F. F. Zhang, Y. Nakamura, K. Liu, H. Wake, K. Hisaoka-Nakashima, M. Nishibori and Y. Nakata, J. Neurochem., 2019, 150, 738–758 CrossRef.
- Y. Y. Xia, M. Xue, Y. Wang, Z. H. Huang and C. Huang, J. Pain Res., 2019, 12, 2851–2863 CrossRef PubMed.
|
This journal is © The Royal Society of Chemistry 2020 |
Click here to see how this site uses Cookies. View our privacy policy here.