DOI:
10.1039/C9RA10428C
(Paper)
RSC Adv., 2020,
10, 10540-10545
A comparative study of high-pressure behaviors of the two polymorphs of Ho2Ge2O7
Received
12th December 2019
, Accepted 17th February 2020
First published on 12th March 2020
Abstract
Two polymorphs of polycrystalline Ho2Ge2O7, one with tetragonal structure and the other with cubic structure, were synthesized by using different methods. The structural stabilities of these two polymorphs under high pressure were investigated by angle-dispersive X-ray diffraction (ADXRD). Pressure-induced amorphization was found in the tetragonal Ho2Ge2O7, which is suggested to be associated with the breaking-up of long chains of the edge-shared polyhedron group Ho4O20. By contrast, cubic Ho2Ge2O7 is stable at high pressures up to 33.3 GPa.
1. Introduction
Spin ice has proven to be one of the most fruitful marriages of theoretical and experimental condensed matter physics.1–4 It is a remarkable magnetic ground state that can arise in geometrically frustrated pyrochlores, A2B2O7, when magnetic rare-earth ions are situated on the vertices of a lattice of corner-sharing tetrahedra. Competing nearest-neighbor and long-range dipolar interactions result in a short-range ordered ground state for each tetrahedron in which two spins point in and two spins point out.5 The spin-ice state was first observed in Ho2Ti2O7 by Harris et al. in 1997;6 since that time, spin ices have been a subject of active experimentation, allowing theorists to come a long way towards understanding this remarkable ground state. Despite significant interest in this class of compounds, only a handful of spin-ice materials have been discovered to date, including the titanates A2Ti2O7,6–8 the stannates A2Sn2O7
9,10 and, more recently, the germanates A2Ge2O7.11,12 Recently, the observation of emergent monopole excitations which have captured the attention of the broader scientific community have made spin-ice materials more intriguing.13–18 Ho2Ge2O7, a member of the rare-earth pyrogermanate series A2Ge2O7, has been attracting extensive interest because it was found to be a new highly correlated spin-ice material with the highest density of monopoles in the Ho series at low temperatures, and the best natural candidate for monopole studies.19
From a structural point of view, rare-earth pyrogermanates A2Ge2O7 possess a variety of crystal structures under ambient conditions, such as triclinic phase for Ln = La, Pr, Nd–Gd, tetragonal phase for Ln = Gd–Lu, and monoclinic phase for In2Ge2O7 and Sc2Ge2O7. Moreover, depending on the synthesis method, different structural modifications of Ln2Ge2O7 can be obtained. By conventional solid state synthesis, Ho2Ge2O7 has the tetragonal structure. However, the cubic phase of Ho2Ge2O7 can be synthesized by the high-pressure and high-temperature (HPHT) method. Therefore, pressure is an important weapon in a researcher's arsenal for exploring phase space. Pressure is also used to drive materials into new electronic states. Under high pressure, some materials become superconductors, others undergo magnetic phase transitions, and others undergo metal–insulator phase transitions.20,21 In magnetic pyrochlore oxides, pressure has been shown to freeze the spin-liquid ground state of Tb2Ti2O7.22 So, studies on the stability of Ho2Ge2O7 under high pressure are particularly important to understand its exotic magnetic phenomenon.
In this work, we successfully synthesized the two types of Ho2Ge2O7 using different methods. The structural stabilities of these two polymorphs of Ho2Ge2O7 were investigated by angle-dispersive synchrotron X-ray powder diffraction (ADXRD) at high pressures. Pressure-induced amorphization was found in the tetragonal Ho2Ge2O7. Meanwhile, the cubic Ho2Ge2O7 was stable up to the highest pressure tested.
2. Experimental section
Synthesis
The tetragonal Ho2Ge2O7 was synthesized by standard solid state reaction method. High purity oxides of Ho2O3 (99.99%, powder) and GeO2 (99.99%, powder) were used as the starting materials. The raw materials with nominal compositions of Ho2Ge2O7 were uniformly mixed in an agate mortar. The powder obtained was pressed into small pellets and then calcined at 1373 K in air for 12 h. The cubic Ho2Ge2O7 was synthesized by the HPHT method. The as-prepared powders were loaded into a cubic anvil HPHT apparatus (SPD-6 × 600) at a temperature of 1573 K and a pressure of 5.2 GPa with a holding time of 15 min.
Characterization
Under ambient conditions, the crystal phase structures of the synthesized samples were characterized by X-ray powder diffraction (XRD) using a Rigaku D/max-2500 with Cu Kα radiation (λ = 1.54056 Å) in the range 2θ from 10° to 90° at a scanning rate of 4° min−1. The high-pressure angle-dispersive XRD patterns for the two types of Ho2Ge2O7 were collected at beamline 4W2 of the Beijing Synchrotron Radiation Facility, using a monochromatic wavelength of 0.6199 Å. A diamond-anvil cell (DAC) was utilized to generate high pressure, using a T301 stainless steel gasket which was pre-indented to 50 μm thickness. One piece of the as-prepared samples, a small piece of ruby as the pressure calibrant23 and a 16
:
3
:
1 methanol/ethanol/water mixture as pressure-transmitting medium were loaded into the diamond-anvil cells. The experimental parameters, including the distance between sample and detector, were calibrated using CeO2 standard reference material. FIT2D software was employed to convert the image plate records into intensity versus diffraction angle 2θ patterns. Rietveld analyses were performed with the software GSAS.24 The refinement parameters were the lattice constants, the atomic position of oxygen, a Chebyshev polynomial background, pseudo-Voigt profile parameters, a common isotropic thermal parameter for all atom sites and an overall intensity scaling factor.
3. Results and discussion
Crystal structures under ambient conditions
The observed and calculated XRD patterns of the two types of Ho2Ge2O7 together with their differences are shown in Fig. 1. The diffraction peaks in Fig. 1a match well with the tetragonal structure Ho2Ge2O7 (S.G. P41212, no. 92) and the obtained cell parameters are: a = b = 6.8041(1) Å, c = 12.3734(1) Å, V = 572.83(1) Å3 with Z = 4. In this kind of crystal structure, each Ho3+ ion is coordinated to seven oxygen atoms. The Ge2O7 unit consists of two tetrahedra (GeO4) joined by a bridging oxygen atom. The bridging oxygen atoms of the Ge2O7 unit do not coordinate to the Ho3+ ion. The coordination polyhedron of the Ho3+ ion is a distorted pentagonal bipyramid with the Ho3+ ion located nearly in the basal plane. The pentagonal axis is almost parallel to the crystal c-axis. Each HoO7 polyhedron shares three of its O–O edges with neighboring polyhedra. Schematic illustrations are shown in Fig. 2a and b.
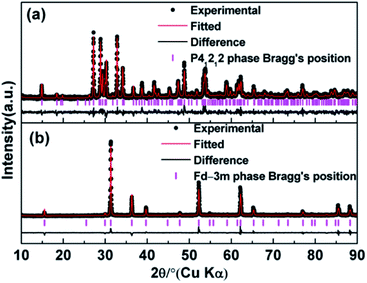 |
| Fig. 1 Observed, calculated and difference X-ray powder patterns of Ho2Ge2O7 at ambient pressure: (a) Rietveld refinement for the tetragonal Ho2Ge2O7 and (b) Rietveld refinement for the cubic Ho2Ge2O7. | |
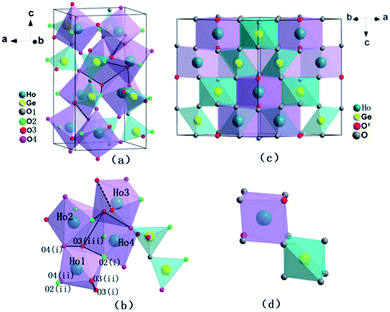 |
| Fig. 2 Schematic representation of the crystal structures of the tetragonal Ho2Ge2O7 ((a) and (b)) and the cubic Ho2Ge2O7 ((c) and (d)). | |
The crystal structure data of the cubic Ho2Ge2O7 were refined by Rietveld analysis of the X-ray powder diffraction data. The observed and calculated XRD patterns along with the difference plot are shown in Fig. 1b. The cubic Ho2Ge2O7 belongs to the Fd
m (no. 227) space group with the lattice parameters a = 9.8974(3) Å and Z = 8. In this cubic phase, it can be formulated as Ho2Ge2O6O′ with the Ge ion site at 16c, Ho at 16d, O at 48f and O′ at 8b. The Ho site (16d) coordination polyhedron is a distorted cube that generally contains larger cations, and the Ge site (16c) is a distorted octahedron. It is worth noting that there is only one adjustable positional parameter x for the O atom at the 48f site. Schematic illustrations of the cubic Ho2Ge2O7 are shown in Fig. 2c and d. In addition, the refined atomic position coordinates of the two polymorphs of Ho2Ge2O7 are given in Table 1.
Table 1 The refined atomic coordinates of the cubic and tetragonal Ho2Ge2O7 at ambient pressure
Rwp and Rp as defined in GSAS.24 |
Compound |
Ho2Ge2O7 |
Ho2Ge2O7 |
Crystal system |
Cubic |
Tetragonal |
Space group |
Fd m (227) |
P41212 (92) |
a/Å |
9.8974(3) |
6.8041(1) |
b/Å |
9.8974(3) |
6.8041(1) |
c/Å |
9.8974(3) |
12.3734(1) |
Atoms |
Wyckoff (x y z) |
Wyckoff (x y z) |
Ho |
16d (0.5 0.5 0.5) |
8a (0.8761(7) 0.3413(8) 0.1352(1)) |
Ge |
16c (0 0 0) |
8a (0.8857(3) 0.1469(9) 0.6199(4)) |
O(1) |
48f (0.3255(10) 0.125 0.125) |
4a (0.8141(5) 0.1859(6) 0.7500(8)) |
O(2) |
8b (0.375 0.375 0.375) |
8a (−0.0319(8) 0.1362(6) 0.6260(2)) |
O(3) |
|
8a (0.0565(6) 0.3575(4) 0.5886(7)) |
O(4) |
|
8a (0.6774(3) 0.1254(6) 0.5528(4)) |
Residualsa/% |
Rwp: 7.07% |
Rwp: 5.83% |
Rp: 5.64% |
Rp: 4.61% |
Pressure-induced amorphization of the tetragonal Ho2Ge2O7
The in situ XRD patterns of the tetragonal Ho2Ge2O7 at various pressures up to 22.5 GPa were collected and a few representative patterns are shown in Fig. 3. As can be seen from Fig. 3a, the pressure-dependent X-ray data do not reveal any new diffraction peaks or peak splitting, which indicates that a typical tetragonal structure remains from ambient pressure up to 13.6 GPa. With further increasing pressure, most of the sharp bands have disappeared at 16.9 GPa and no diffraction peaks can be observed, which suggests the formation of an amorphous phase. After releasing the pressure, pressure-induced amorphization of Ho2Ge2O7 is maintained, which indicates the nonreversible nature of the phase transition. A few small peaks appeared after quenching and holding the sample under ambient conditions for 10 h. The recorded pattern for 10 h after release of pressure is almost identical to that of the original phase of tetragonal Ho2Ge2O7, as shown in Fig. 3b.
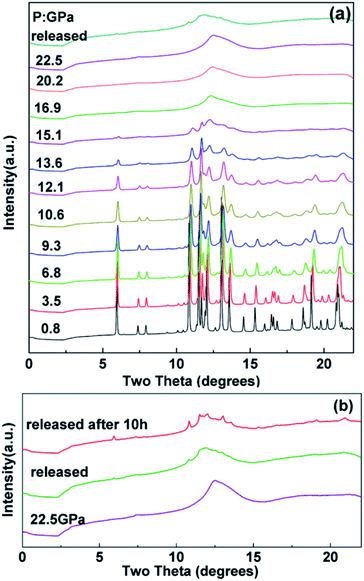 |
| Fig. 3 (a) Representative X-ray diffraction patterns of the tetragonal Ho2Ge2O7 at various pressures. (b) X-ray diffraction pattern for Ho2Ge2O7 after the release of pressure. | |
Pressure-induced amorphization has been the subject of intense study for the past few years because of its importance in materials science and solid state physics.25–27 A pressure-induced amorphization of α-NaVO3 was observed by Raman spectroscopy, which involved the tetrahedral VO4 chains breaking up abruptly at the transition pressure.28 In addition, silicates and metavanadate compounds also have chain structures and are the best examples of amorphous materials.29–31 As can be seen in Fig. 2a and b, each HoO7 polyhedron shares three of its O–O edges with neighboring polyhedra. And we can clearly see the four polyhedra (Ho1O7, Ho2O7, Ho3O7, Ho4O7) close together. The Ho1O7 polyhedron and Ho3O7 polyhedron share an O3(i)–O3(ii) edge with the four other closest polyhedra. In the crystal structure of Ho2Ge2O7, there is a chain, whose constituent unit (Ho4O20) is four edge-shared polyhedrons closely linked. In order to understand the reason for the amorphization, we plotted the variation of bond length with pressure for tetragonal Ho2Ge2O7 (Fig. 4). The bond lengths of Ho–O3(i) and Ho–O3(ii) always remain longer than the other Ho–O bond distance, from ambient pressure to the highest pressure. So the infinite Ho4O20 chain is relatively easy to disconnect at this junction. Pressure-induced amorphization of Ho2Ge2O7 is suggested to be associated with the breaking-up of long chains of the edge-shared polyhedron group Ho4O20. The tetragonal Ho2Ge2O7 showed long-range order at low magnetic fields, but its behavior was similar to spin-ice freezing in a sufficiently strong magnetic field.32 Owing to the appearance of pressure-induced amorphization, we can speculate that the tetragonal Ho2Ge2O7 may become paramagnetic under high pressure.
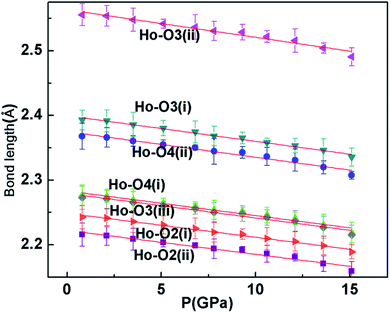 |
| Fig. 4 The Ho–O bond lengths in the tetragonal Ho2Ge2O7 at various pressures. | |
The pressure dependences of the lattice parameters and volume of tetragonal Ho2Ge2O7 up to 22.5 GPa are shown in Fig. 5. It is shown that the lattice parameters and volume of Ho2Ge2O7 decrease smoothly with increasing pressure. In order to determine the bulk modulus B0, its pressure derivative
, and the molar volume under ambient conditions V0, the experimental pressure–volume data of the Ho2Ge2O7 were fitted to a third-order Birch–Murnaghan equation of state (Fig. 5c).33 The obtained bulk modulus B0 is 193(4) GPa for the tetragonal phase with fixed
= 4.
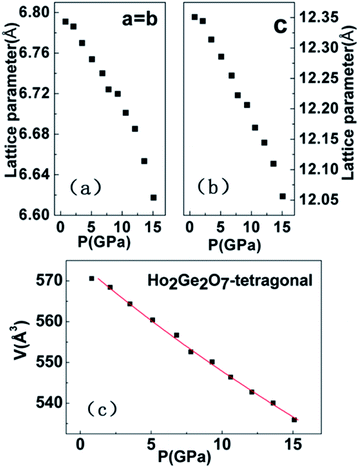 |
| Fig. 5 The lattice parameters ((a) and (b)) and unit cell volume (c) as a function of pressure for the tetragonal Ho2Ge2O7. | |
Structural stability of the cubic Ho2Ge2O7 under high pressure
In situ XRD patterns of the cubic Ho2Ge2O7 were collected up to 33.3 GPa at room temperature and the representative patterns are shown in Fig. 6. No splitting or extra peaks appear in the patterns, demonstrating that the cubic phase of the Ho2Ge2O7 remains stable within the whole pressure range. In this ordered pyrochlore structure, all of the atoms are sited at defined positions, except for the O48f atom. Hence, the degree of structural ordering can be determined by varying the x positional parameter of the O48f atom. Previous studies on pyrochlore oxides have revealed that a sudden change of the x positional parameter of the O48f atom occurred in the process of pressure-induced structural phase transition. For example, there was a rapid decrease of the x positional parameter with increasing pressure in Gd2Zr2O7, implying a phase transition;34 the x-coordinate of the O48f atom increased dramatically after the transformation in Sm2Zr2O7.35 From our refined results, the x parameter for the O48f atom as a function of pressure, as shown in the inset of Fig. 7, does not exhibit any anomaly up to the highest pressure employed, indicating the structural stability of the cubic Ho2Ge2O7. Recent experimental studies have reported that the pyrochlore Ho2Ge2O7 exhibited all the distinctive properties of a dipolar spin ice: a small, positive Curie–Weiss constant; Pauling zero-point entropy; magnetization which saturated to half the magnetic moment; a spin-freezing transition in the ac susceptibility; and the characteristic magnetic diffuse scattering of spin ices.19 The stability of the structure ensures the stability of these excellent properties under high pressure.
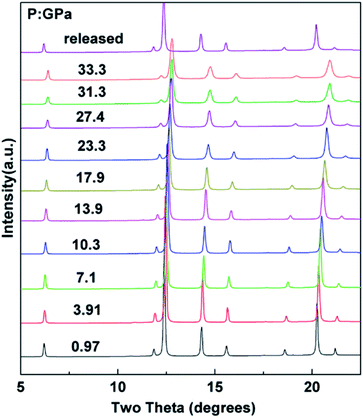 |
| Fig. 6 Selected XRD patterns of the cubic Ho2Ge2O7 with increasing pressure. The cubic structure is stable up to 33.3 GPa. | |
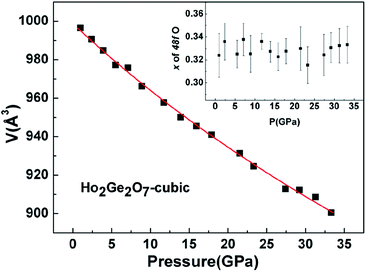 |
| Fig. 7 Observed P–V variation fitted with the third-order Birch–Murghnan (B–M) equation of state for the cubic Ho2Ge2O7. The inset shows the pressure dependence of the x parameter for the O48f atom. | |
The pressure dependence of the unit cell volume of the cubic Ho2Ge2O7 is shown in Fig. 7. The data are fitted by a third-order Birch–Murnaghan equation of state as is the case for the tetragonal Ho2Ge2O7. The obtained bulk modulus B0 is 263(4) GPa, quite a bit larger than for other ordered pyrochlore oxide materials. For example, the bulk moduli of A2Ti2O7 (A = Ho, Y, Tb, Sm) were 213(2), 204(3), 199(1) and 164.8(1.5) GPa, respectively.36,37 And also for Gd2Zr2O7, the bulk modulus was 186(12) GPa.34 By comparison, the hardness of the cubic phase in Ho2Ge2O7 is higher than that of the tetragonal phase. The difference in bulk modulus might be due to the differences in structure and cohesive energy among these germanates.
4. Conclusions
In summary, the structural behaviors of two types of Ho2Ge2O7 were studied under high pressure by in situ XRD measurements. Pressure-induced amorphization was found in the tetragonal Ho2Ge2O7, which has a chain structure, and is suggested to be associated with the breaking-up of long chains of the edge-shared polyhedron group Ho4O20. By contrast, the cubic Ho2Ge2O7 structure is stable at high pressures up to 33.3 GPa. The bulk modulus of the tetragonal Ho2Ge2O7 was obtained as B0 = 193(4) GPa, and B0 = 263(4) GPa was obtained for the cubic phase.
Conflicts of interest
There are no conflicts to declare.
Acknowledgements
This work was financially supported by the National Natural Science Foundation of China under Grant No. 51172091, the Natural Science Foundation of Guangxi under grant No. 2018GXNSFBA050034, the Young and Middle-aged Teachers Basic Ability Improvement Project of Guangxi Department of Education, No. 2017KY0168, Introduction of Talent Research Projects, No. 2016MDQD001 and the Xiangsihu Young Scholars and Innovative Research Team of GXUN.
References
- S. T. Bramwell and M. J. P. Gingras, Science, 2001, 294, 1495–1501 CrossRef CAS PubMed.
- J. S. Gardner, M. J. P. Gingras and J. E. Greedan, Rev. Mod. Phys., 2010, 82, 53–107 CrossRef CAS.
- C. Castelnovo, R. Moessner and S. L. Sondhi, Annu. Rev. Condens. Matter Phys., 2012, 3, 35–55 CrossRef CAS.
- M. J. P. Gingras and P. A. McClarty, Rep. Prog. Phys., 2014, 77, 056501 CrossRef CAS PubMed.
- S. T. Bramwell, M. J. Harris, B. C. den Hertog, M. J. P. Gingras, J. S. Gardner, D. F. McMorrow, A. R. Wildes, A. L. Cornelius, J. D. M. Champion, R. G. Melko and T. Fennell, Phys. Rev. Lett., 2001, 87, 047205 CrossRef CAS PubMed.
- M. J. Harris, S. T. Bramwell, D. F. McMorrow, T. Zeiske and K. W. Godfrey, Phys. Rev. Lett., 1997, 79, 2554 CrossRef CAS.
- A. P. Ramirez, A. Hayashi, R. J. Cava, R. Siddharthan and B. S. Shastry, Nature, 1999, 399, 333–335 CrossRef CAS.
- T. Fennell, O. A. Petrenko, B. Fåk, S. T. Bramwell, M. Enjalran, T. Yavors’kii, M. J. P. Gingras, R. G. Melko and G. Balakrishnan, Phys. Rev. B: Condens. Matter Mater. Phys., 2004, 70, 134408 CrossRef.
- H. Kadowaki, Y. Ishii, K. Matsuhira and Y. Hinatsu, Phys. Rev. B: Condens. Matter Mater. Phys., 2002, 65, 144421 CrossRef.
- K. Matsuhira, Y. Hinatsu, K. Tenya, H. Amitsuka and T. Sakakibara, J. Phys. Soc. Jpn., 2002, 71, 1576–1582 CrossRef CAS.
- H. D. Zhou, J. G. Cheng, A. M. Hallas, C. R. Wiebe, G. Li, L. Balicas, J. S. Zhou, J. B. Goodenough, J. S. Gardner and E. S. Choi, Phys. Rev. Lett., 2012, 108, 207206 CrossRef CAS PubMed.
- H. D. Zhou, S. T. Bramwell, J. G. Cheng, C. R. Wiebe, G. Li, L. Balicas, J. A. Bloxsom, H. J. Silverstein, J. S. Zhou, J. B. Goodenough and J. S. Gardner, Nat. Commun., 2011, 2, 478 CrossRef CAS PubMed.
- C. Castelnovo, R. Moessner and S. L. Sondhi, Nature, 2008, 451, 42–45 CrossRef CAS PubMed.
- S. T. Bramwell, S. R. Giblin, S. Calder, R. Aldus, D. Prabhakaran and T. Fennell, Nature, 2009, 461, 956–959 CrossRef CAS PubMed.
- L. D. C. Jaubert and P. C. W. Holdsworth, Nat. Phys., 2009, 5, 258–261 Search PubMed.
- D. J. P. Morris, D. A. Tennant, S. A. Grigera, B. Klemke, C. Castelnovo, R. Moessner, C. Czternasty, M. Meissner, K. C. Rule, J.-U. Hoffmann, K. Kiefer, S. Gerischer, D. Slobinsky and R. S. Perry, Science, 2009, 326, 411–414 CrossRef CAS PubMed.
- T. Fennell, P. P. Deen, A. R. Wildes, K. Schmalzl, D. Prabhakaran, A. T. Boothroyd, R. J. Aldus, D. F. McMorrow and S. T. Bramwell, Science, 2009, 326, 415–417 CrossRef CAS.
- S. R. Giblin, S. T. Bramwell, P. C. W. Holdsworth, D. Prabhakaran and I. Terry, Nat. Phys., 2011, 7, 252–258 Search PubMed.
- A. M. Hallas, J. A. M. Paddison, H. J. Silverstein, A. L. Goodwin, J. R. Stewart, A. R. Wildes, J. G. Cheng, J. S. Zhou, J. B. Goodenough, E. S. Choi, G. Ehlers, J. S. Gardner, C. R. Wiebe and H. D. Zhou, Phys. Rev. B: Condens. Matter Mater. Phys., 2012, 86, 134431 CrossRef.
- S. Deemyad and J. S. Schilling, Phys. Rev. Lett., 2003, 91, 167001 CrossRef.
- A. G. Gavriliuk, V. V. Struzhkin, I. S. Lyubutin, S. G. Ovchinnikov, M. Y. Hu and P. Chow, Phys. Rev. B: Condens. Matter Mater. Phys., 2008, 77, 155112 CrossRef.
- I. Mirebeau, I. N. Goncharenko, P. Cadavez-Pares, S. T. Bramwell, M. J. P. Gingras and J. S. Gardner, Nature, 2002, 420, 54–57 CrossRef CAS PubMed.
- H. K. Mao, J. Xu and P. M. Bell, J. Geophys. Res., 1986, 91, 4673–4676 CrossRef CAS.
- A. C. Larson and R. B. Von Dreele, General Structure Analysis System, LANSCE, MS-H805, Los Alamos, New Mexico, 1994 Search PubMed.
- E. G. Ponyatovsky and O. I. Barkalov, Mater. Sci. Rep., 1992, 8, 147–191 CrossRef.
- A. V. Kolobov, J. Haines, A. Pradel, M. Ribes, P. Fons, J. Tominaga, C. Steimer, G. Aquilanti and S. Pascarelli, Appl. Phys. Lett., 2007, 91, 021911 CrossRef.
- X. Q. Yan, W. J. Li, T. Goto and M. W. Chen, Appl. Phys. Lett., 2006, 88, 131905 CrossRef.
- Z. X. Shen, C. W. Ong, S. H. Tang and M. H. Kuok, J. Phys. Chem. Solids, 1994, 55, 665–669 CrossRef CAS.
- L. E. McNeil and M. Grimsditch, Phys. Rev. Lett., 1992, 68, 83–85 CrossRef CAS PubMed.
- C. Mead, R. J. Hemley and H. K. Mao, Phys. Rev. Lett., 1992, 69, 1387–1390 CrossRef PubMed.
- Y. Li, R. Tang, N. Li, H. Li, X. Zhao, P. Zhu and X. Wang, J. Appl. Phys., 2015, 118, 035902 CrossRef.
- E. Morosan, J. A. Fleitman, Q. Huang, J. W. Lynn, Y. Chen, X. Ke, M. L. Dahlberg, P. Schiffer, C. R. Craley and R. J. Cava, Phys. Rev. B: Condens. Matter Mater. Phys., 2008, 77, 224423 CrossRef.
- F. Birch, J. Geophys. Res., 1978, 83, 1257–1268 CrossRef CAS.
- F. X. Zhang, J. Lian, U. Becker, R. C. Ewing, J. Hu and S. K. Saxena, Phys. Rev. B: Condens. Matter Mater. Phys., 2007, 76, 214104 CrossRef.
- F. X. Zhang, J. Lian, U. Becker, L. W. Wang, J. Hu, S. K. Saxena and R. C. Ewing, Chem. Phys. Lett., 2007, 441, 216–220 CrossRef CAS.
- P. R. Scott, A. Midgley, O. Musaev, D. Muthu, S. Singh, R. Surya-narayanan, A. Revcolevschi, A. Sood and M. Kruger, High Pressure Res., 2011, 31, 219–227 CrossRef CAS.
- F. X. Zhang, B. Manoun, S. K. Saxena and C. S. Zha, Appl. Phys. Lett., 2005, 86, 181906 CrossRef.
|
This journal is © The Royal Society of Chemistry 2020 |
Click here to see how this site uses Cookies. View our privacy policy here.