DOI:
10.1039/D0RA01507E
(Review Article)
RSC Adv., 2020,
10, 23221-23232
Describing the toxicity and sources and the remediation technologies for mercury-contaminated soil
Received
17th February 2020
, Accepted 31st May 2020
First published on 17th June 2020
Abstract
Mercury (Hg) is a natural element and its compounds are found as inorganic and organic forms in the environment. The different Hg forms (e.g., methylmercury (MeHg)), are responsible for many adverse health effects, such as neurological and cardiovascular effects. The main source of Hg is from natural release. Nevertheless, with the development of industrialization and urbanization, Hg-contaminated soil mainly influenced by human activities (especially near mercury mining areas) has become a problem. Therefore, much more attention has been paid to the development and selection of various treatment methods to remediate Hg-contaminated soils. This paper presented a systematical review of the recent developments for the remediation of Hg-contaminated soils. Firstly, we briefly introduced the Hg chemistry, toxicity and the main human activity-related sources of mercury in soil. Then the advances in remediation technologies for removing Hg pollution from the soil were summarized. Usually, the remediation technology includes physical, chemical and biological remediation technology. Depending on this, we further classified these remediation technologies into six techniques, including thermal desorption, electrokinetic extraction, soil washing, chemical stabilization, phytoremediation and microbial technology. Finally, we also discussed the challenges and future perspectives of remediating Hg-contaminated soils.
1. Introduction
Mercury (Hg) is one of the most toxic heavy metals and is listed as a global pollutant.1 Mercury released from natural and human activities is widely distributed worldwide.2 In the 1950s, Minamata disease was discovered in the Minamata Bay of Japan,3 which led to a global concern regarding Hg pollution. Because of its special physical and chemical properties, Hg exists in liquid form at room temperature and normal pressure, unlike other heavy metals. Moreover, Hg can also exist in the gas form at room temperature and pressure and can be retained in the atmosphere for a long time.4 Once Hg is released into the air, it will stay there in gaseous form and transmit with the atmosphere over long distances before eventual deposition on soil surface.5 The United Nations Environment Programme (UNEP) reported that the global anthropogenic Hg emissions to the atmosphere in 2015 were estimated to be approximately 2220 tons.6 The main anthropogenic sources of Hg include minerals, industrial activities and fossil fuels combustion.7 And anthropogenic mercury can enter the soil through the ways of wet and dry deposition, discharge of waste, use of mercury-containing fertilizers and pesticides.8
With an increase in human activities, including agricultural and industrial activities, anthropogenic emissions have increased the Hg pollution in soil. For example, large-scale Hg mining activities have caused severe Hg pollution to the surrounding area of the mining area.9 Hg can be absorbed by plants and accumulate in the human body through the food chain.5 Furthermore, it is a nonessential toxic heavy metal for the human body. Owing to the toxic effects of Hg on environmental and human health, Hg pollution in soil has increasingly attracted attention.10
Therefore, it is urgent to develop efficient methods to remove Hg from the soil. In recent years, people have been developing materials and technologies for remediation of mercury pollution.10–14 The main Hg removal technologies are physical and chemical remediation, as well as bioremediation technology. In this review, we briefly introduce Hg chemistry and its toxicity and describe in detail the anthropogenic Hg emissions in soil. Then, we systematically summarize the soil remediation technologies, including thermal desorption, electrokinetic remediation, soil washing, chemical stabilization, plant and microbial remediation. Finally, we also discuss the potential challenges and future perspectives for remediation of Hg-contaminated soils.
2. Toxicity of mercury
Mercury in soil exists in the form of inorganic and organic Hg, including metallic or elemental mercury (Hg0) and mercurous (Hg22+), mercuric (Hg2+) and alkylated compounds.15,16 Among these Hg species, common Hg compounds mainly include mercuric sulfide (HgS), mercuric chloride (HgCl2), mercuric oxide (HgO), methylmercury (MeHg), dimethyl mercury ((CH3)2Hg), ethyl mercury (C2H5Hg) and phenylmercury (C6H5Hg).17 Mehyl-Hg is an important metabolite of microbial action and inorganic Hg is converted to MeHg through the methylation of microorganisms. Under anoxic and suboxic conditions, inorganic Hg is transformed into MeHg through the action of sulfate-reducing bacteria and iron-reducing bacteria.18 Fig. 1 displays the geochemical cycle of Hg in different forms.10,19
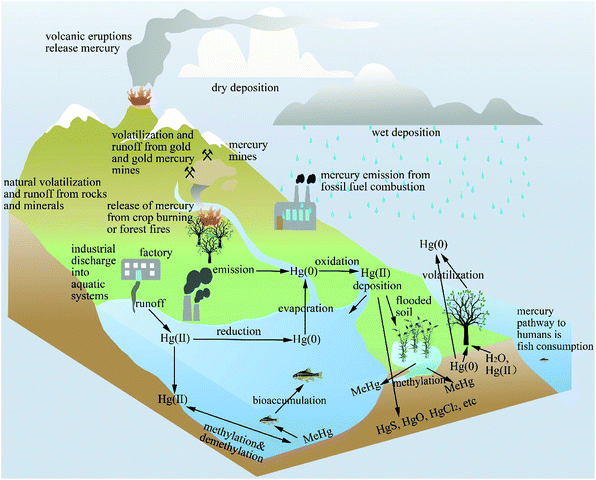 |
| Fig. 1 Geochemical cycle of mercury (Hg).10,19 | |
Mercury and its compounds can cause serious damage to human health. It has been considered to be a neurotoxin and immunotoxin and has been listed as one of the ten most dangerous chemicals to public health by the World Health Organization (WHO).20 Inorganic Hg can inhibit the activity of enzymes in the body, destroy the normal metabolism of cells, thus affecting various body functions. Exposure to organic Hg can cause neurological and cardiovascular diseases.21 Methylmercury, the organic form of Hg, has a great negative effect on brain function, and its toxicity can be accumulated and biomagnified in the food chain.10 Mercury can be transferred to foetuses through the placenta.22 Previous studies have shown that excessive intake of MeHg by pregnant women could affect the intelligence of newborns, thus expectant mothers have a high risk of MeHg exposure if they consume too many Hg-contaminated products during pregnancy.4,23 In addition, patients with chronic Hg poisoning will still experience distal paraesthesia of their extremities and lips 30 years after cessation of MeHg exposure.24
3. Sources of mercury in soil
The sources of Hg pollution in soil mainly include naturally release and anthropogenic discharge. Fig. 1 demonstrates the sources of Hg from natural release and anthropogenic emission.10,19 According to the geological background of high Hg, the distribution of Hg in the soil is not uniform, thereby different soils have varying concentration of Hg. Furthermore, the natural weathering process and activities of the Earth's crust also continue to release Hg into the soil. However, anthropogenic emissions have been an important source of Hg pollution in recent years. The global assessment records of the UNEP show that the deposition rate of Hg has increased by 1.5–3 times in the past century due to an increase in industrial and agricultural activities and pollution emissions, such as medical treatments.25 Therefore, we do not discuss natural Hg emissions in this paper.
The major global Hg reservoirs (atmosphere, terrestrial environments (especially soil) and aquatic ecosystems (e.g., ocean)) continue to be affected by anthropogenic activities.26 Anthropogenic emissions are one of the main sources of Hg pollution in soil. In daily life and production, industrial and agricultural activities (intentional or unintentional) directly discharge large amounts of Hg into the environment, resulting in Hg pollution in soil. The anthropogenic activities that emit Hg mainly include coal combustion, garbage incineration, metal refining and manufacturing, chlorine alkali industries, mercury mining and metallurgy, landfill and so on.5,9 In addition, research shows that dry and wet atmospheric depositions have an important contribution to Hg pollution in soil. The concentration of atmospheric mercury in China is about 2–10 times the background value of northern hemisphere.27 The estimation results show that atmospheric Hg deposition accounts for more than 60% of the total agricultural soil Hg input in China.8
3.1. Mercury minerals
Mining activities, especially mercury mining, can cause serious Hg-contamination of mines and surrounding area, hence Hg pollution in various Hg mining areas always received much more attention. For example, Guizhou province of China is located in the Hg ore belt around the Pacific Ocean and is known as the “mercury capital” of China,25,28 which has a long 3000 years history of mining.29 The metal Hg reserves account for 80% of the total Hg reserves in China.28 Mining operations have significantly promoted the mobilization of Hg through smelting activities and the deposition of mine tailings, leading to increased levels of Hg in soil.29 Long-term mining activities and illegal handicraft mining have led to high total concentration of Hg in soil, air, water, sediments and organisms in Wanshan, Guizhou province.25,30 In detail, the results show that the concentration of Hg in the tailings of the Wanshan Hg mining area is up to 4400 mg kg−1, and the concentration of Hg in the surface soil of the Hg mining area is as high as 790 mg kg−1.29 This means that the tailings have a significant effect on the content of Hg in soils of the mining area. Similarly, the paddy soil near the abandoned handicraft mining area has high amounts of total Hg and MeHg.31 Moreover, Hg pollution in the mining area not only affects the surface soil but also migrates downward. The distribution of Hg in the Wanshan soil shows that the concentration of Hg is 0.48 mg kg−1 at a soil depth of 45 cm, and the depth of the abnormal Hg concentration can reach 50 cm.9 Herein, in the 12th Five Year Plan for the prevention and control of heavy metal pollution, China has listed Hg pollution as one of the key prevention and control targets of heavy metal pollution, especially Hg pollution-related mining areas and surrounding areas (http://www.gov.cn).
3.2. Metal smelting
Many studies indicate that metal smelting, especially nonferrous metal smelting, cause serious Hg pollution in the surrounding environment. Zinc smelting is considered an important anthropogenic source of Hg pollution in nearby the soil. During the process of Zn smelting with sulfide compounds, the desulfurization, coal combustion, and final smelting process are the three main sources of Hg emission.32 Since Zn and Hg are both sulfur-friendly elements, mercury is an important element related to zinc in zinc ores.33 And the concentration of Hg in zinc ores can reach 100–300 mg kg−1.34 Therefore, in the process of zinc smelting, Hg can be inevitably volatilized from ores to the atmosphere in the form of Hg0, reactive gaseous Hg and particulate Hg.34 Zheng et al.35 analyzed Hg concentration in the surrounding environment of a zinc smelter in Liaoning Province of China and found that Hg deposition is an important source of Hg in the surrounding soil, vegetables and corn, when zinc smelting occurs. The spatial distribution of metal elements in the paddy soil around the large-scale lead–zinc smelter in Zhuzhou City, Hunan Province shows that Hg pollution in the soil around the smelter is serious: the assessment results indicate that the amount of Hg in the soil is highly increased; the potential ecological risk assessment shows that the soil is at a relatively high risk of Hg pollution within a radius of 4 km from the smelter; and the contribution rate of Hg reaches up to 19.4%.36 Therefore, metal smelting is an important source of Hg pollution in soil.
3.3. Coal combustion
Approximately 75% of Hg in the atmosphere comes from fossil fuel combustion, especially the combustion of coal.25 The soil is the largest sink of Hg in the atmosphere. Most of the Hg produced by combustion can enter a terrestrial ecosystem and accumulate in the soil. The results of Monitoring Centre showed that high Hg-contaminated soil in Beijing, Guiyang, Guilin, Guangzhou, Nanjing, Taiyuan, and Chongqing was caused by a great deal of coal combustion.25 Usually, the environment around a coal-fired power plant is the most significant place polluted by Hg emission.37,38 The operation of the Baoji Coal-Fired Power Plant in Shanxi province for many years has resulted in high Hg concentrations in the surrounding soil. The Hg concentration ranges from 0.137 to 2.105 mg kg−1 with an average value of 0.606 mg kg−1, which is higher than the maximum Hg concentration threshold in agricultural soil for the United Kingdom and China.39
4. Remediation of Hg-contaminated soils
Generally, soil contamination has characteristics of invisibility, lag, and accumulation. For heavy metal contamination, it has the characteristic of irreversibility. Once heavy metals contaminate the soils, it is difficult to completely remove from them. Mercury is persistent and volatile due to its special physical and chemical properties. It can enter the soil through many pathways and can exist in the soil in different forms, causing serious soil pollution. Therefore, Hg pollution treatment and remediation in soil have received much more attention. The physical and chemical properties of soil and occurrence of Hg are important factors affecting remediation efficiency. Therefore, appropriate remediation technology should be adopted depending on the soil properties and the morphological characteristics of Hg in the soil.
In summary, there are two remediation technology mechanisms for Hg-contaminated soils: (1) removing harmful substances from the soil to reduce the total concentration of Hg; and (2) changing the mobility and biological availability of Hg in the soil to reduce the risk of pollution.40 According to the remediation mechanisms, remediation of Hg-contaminated soils can be divided into physical, chemical and biological remediation. Fig. 2 shows the main technologies for remediation of heavy metal contaminated soils and Table 1 shows several major remediation techniques for contamination in the soil.
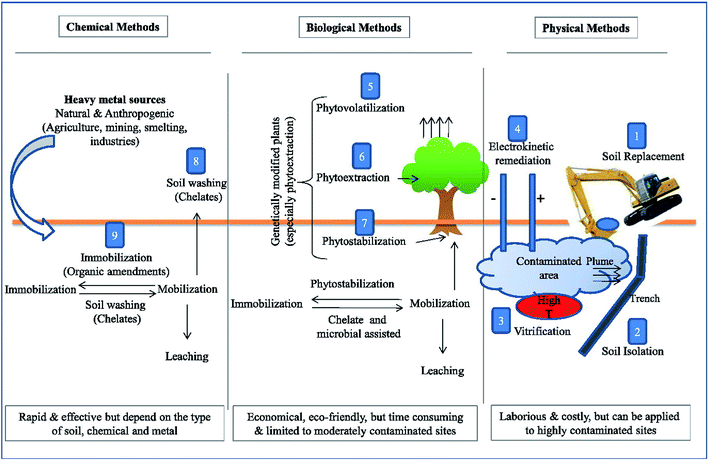 |
| Fig. 2 The technologies for remediation of heavy metal contaminated soils.41 | |
Table 1 Common remediation technologies for contaminated soil
|
Technology |
Operation |
Reagent |
Ref. |
Physical remediation |
Soil replacement |
Cleanning soil replaces contaminated soil |
— |
42 |
Soil vapour extraction |
Reduction of the vapor pressure of soil pores |
— |
43 |
Thermal desorption |
Separation of pollutants from soil by heating |
MgCl2, etc. |
44 |
Electric remediation |
Establish electric field gradient |
KI, EDTA, etc. |
45 and 46 |
Chemical remediation |
Soil washing |
Extraction and separation of contaminants from soil by eluent |
HCl, HNO3, H2SO4, H3PO4, NaCl, Na2S2O3, KI, etc. |
47 |
Chemical stabilization |
Addition of chemical reagents or chemical materials |
Sulfide, phosphate, etc. |
45, 48 and 49 |
Biological remediation |
Phytoremediation |
The use of plants and their associated rhizospheric microorganisms to remove contaminants |
Hyperaccumulators, etc. |
50 and 51 |
Microbial remediation |
Control contaminants in soil by introducing microorganisms |
Bacteria, etc. |
52 and 53 |
Animal remediation |
Utilizing the activities of some lower animals to enhance bioremediation |
Earthworm |
54 and 55 |
4.1. Physical remediation
Physical remediation depends on physical means, mainly including soil replacement, physical separation, soil vapor extraction, fixed/stabilized soil, vitrification, thermal desorption, and electrokinetic remediation technology. In general, physical remediation needs to add chemical reagents into the soil for better remediation efficiency.5
4.1.1. Thermal desorption. Thermal desorption remediates the polluted soil through direct or indirect heating and then separates the pollutants based on a low boiling point in the soil. Thermal desorption technology includes two steps: heating polluted soil to volatilize pollutants and tail gas treatment, and is classified as in situ and ex situ. Generally, in situ thermal desorption is applied with soil vapor extraction which can extract the gas in soil.15 During the stage of thermal desorption, pollutants are volatilized and transported to the flue gas treatment system through the carrier gas.56 The thermal treatment remediation is mainly based on the volatility of contaminants.57 Herein, the treated pollutants used in this technique usually require easy volatilization. Mercury is the only heavy metal in the form of a liquid at room temperature. The melting and boiling points, and vapor tension of Hg0 are −38.8 °C, 356.7 °C, and 0.18 Pa, respectively,5 which all show that Hg is volatile. The rate of vaporization increases with temperature. Therefore, to remediate Hg-contaminated soil, thermal desorption can convert Hg in the soil into the gas phase and collect it through heating.15The factors that affect the efficiency and effectiveness of thermal desorption to removal pollutant from soil include: soil characteristics, organic matter, water content, mercury concentration/form, and operational condition.15 The desorption temperature is different when the forms of Hg in contaminated soil are different. The experimental results of Hg speciation analysis by thermal desorption shows that different forms of Hg in the soil volatilize in a certain order with increasing desorption temperature. The increasing order of temperature that is required for the volatilization of various forms of Hg is HgI2 < HgBr2 < Hg2Cl2 = HgCl2 < Hg(CN)2 < HgCl2O8·H2O < Hg(SCN)2 < HgS (red) < HgF2 < Hg2(NO3)2·2H2O < Hg(NO3)2·H2O < HgO (yellow, red) < Hg2SO4 < HgSO4.58 Additionally, Table 2 shows the thermal desorption temperatures for some Hg compounds. Theoretically, the required temperature of Hg and its compound removal is 873 K from the soil by thermal desorption, while 523 K is sufficient for the removal of Hg without Hg oxide.59 The temperature of a typical thermal desorption device used to remove mercury is between 320–700 °C.2
Table 2 Desorption temperatures of different Hg phases60,61
Phase |
Desorption temperature of phases Hg (°C) |
Hg0 |
119 ± 9 |
HgCl2 |
135 ± 5 |
Hg–FeS2 |
169 ± 5 |
HgS metacinnabar |
190 ± 11 |
Hg-OM (Hg bound toorganic matter) |
217 ± 7 |
HgS cinnabar |
303 ± 13 |
HgO |
308 ± 1; 471 ± 5 |
HgSO4 |
580 ± 19 |
Hg(NO3)2·H2O |
215 ± 4; 280 ± 13; 460 ± 25 |
Hg2Cl2 |
170 |
Hg in pyrite |
>450 |
Hg in sphalerite |
600 |
Hg matrix-bound |
200–300 |
A large number of experiments have proven that thermal desorption can effectively remove Hg from the soil. Kucharski et al.62 calculated that the total Hg concentration in the soil decreased by nearly 32% at 373 K in the laboratory and that the total Hg content decreased by 60–70% at 440 K in a small-scale experiment by thermal desorption. Chang and Yen63 successfully used in situ thermal desorption to remove Hg pollution in the soil in southern Taipei, China. The experimental results show that time and temperature are the two important factors affecting Hg removal in soil by thermal desorption. They found that the equilibrium concentration of Hg in soil decreases with increasing temperature. Furthermore, thermal desorption can effectively remove Hg pollution when the temperature is higher than 700 °C and the time is more than 2 hours. With the development of thermal desorption remediation technology, scholars in this field have carried out more in-depth research on it.
Although the experimental results show that a high temperature is beneficial to Hg volatilization from the soil, a high temperature can destroy organic matter in the soil. For agricultural land, the soil will lose its agricultural function after thermal desorption by high temperature. In the future, it is equally important to maintain acceptable soil quality and Hg removal in the soil for agricultural purposes. Some experts have found that when the soil is heated to 280 °C, it can not only avoid an irreversible loss of the soil quality level but also remediate soil pollution to reduce bioavailable Hg.64 Scholars have conducted many experiments to show that the effect of heating time on the removal efficiency depends on the heating temperature. Thus, a low temperature requires a long heating time to ensure that the pollutants are effectively removed.56 Therefore, low-temperature and long-term thermal desorption cannot only effectively remove Hg but also have little adverse effect on the soil structure compared with high-temperature thermal desorption.
To make more effective and practical use of thermal desorption technology for remediation of Hg pollution in soil without affecting soil quality, some experts have combined salts or acids with thermal desorption technology to improve the removal rate under low-temperature conditions. Zhao et al.44 pointed out that MgCl2 can promote the desorption of HgS, Hg(NO3)2·H2O, HgSO4, and HgO, which are four common Hg compounds. Further experimental results also show that the removal rate of Hg in polluted soil treated with MgCl2 increases significantly, which indicates that MgCl2 can promote the transformation of Hg compounds in the soil and reduce the required temperature.44 In the related research on reducing the temperature of thermal desorption, Comuzzi et al.65 proposed a novel method of combining cation exchange and thermal desorption. Using an appropriate salt exchange treatment, the Hg ion in Hg compounds can be exchanged with cations that have an equivalent or higher molecular weight than Hg, which can improve the removal efficiency of Hg and significantly lower the temperature. Some scholars have proposed that citric acid can be used to promote the thermal desorption of Hg-contaminated soil. Citric acid can provide an acidic environment to enhance the volatilization of Hg. Ma et al.66 found that when the citric acid/Hg molar ratio is 15, this method can reduce the Hg concentration from 134 mg kg−1 to 1.1 mg kg−1 at 400 °C in 60 minutes. More importantly, the treated soil still retains most of the physical and chemical properties of the original soil. Therefore, citric acid is an ideal candidate to promote thermal desorption to remediate Hg-contaminated soil.
4.1.2. Electrokinetic remediation. Electrokinetic remediation is an efficient technology to treat heavy metal-contaminated soils. The basic mechanism is to implant inert electrodes on both sides of the contaminated soil and apply voltage to establish an electric field gradient in a suitable intensity so that heavy metal pollutants in the soil can be fixed to both ends for centralized treatment through electromigration, electroosmosis or electrophoresis; the above lowers the amount of heavy metal in the soil.41 Fig. 3 shows the schematic diagram of electrokinetic remediation device (A) and remediation mechanism (B).67
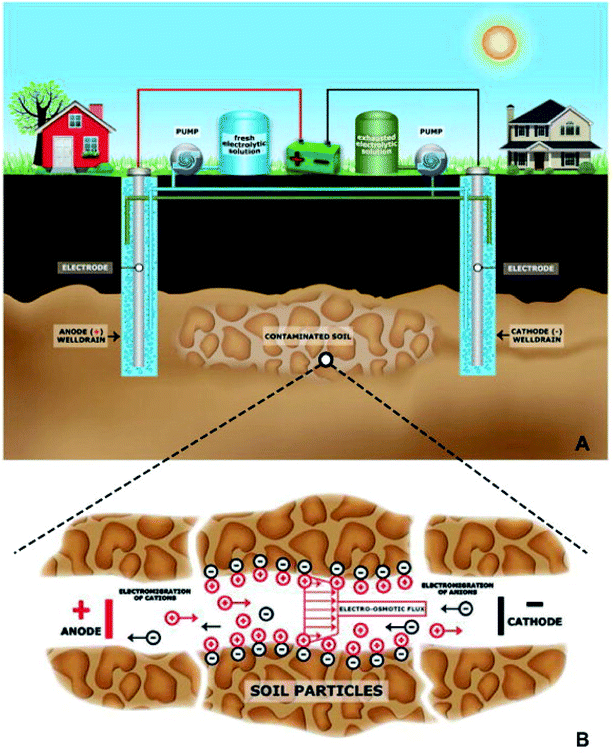 |
| Fig. 3 Schematic diagram of electrokinetic remediation device (A) and mechanism (B).67 | |
The treatment efficiency of direct electrokinetic remediation is relatively low. Accordingly, some methods are used to improve this technology. In general, buffer solution and ion exchange membranes are introduced to control pH, and complexant is used to enhance the ion migration capability.68,69 In addition, Davide et al.67 pointed out that when heavy metal pollutants exist in the form of metal or nonconductive mineral, appropriate chemical reagents need to be added to promote the initial dissolution of pollutants. Therefore, some chemical reagents are usually introduced in the process of electrokinetic remediation. Many studies have shown that iodine solutions can effectively improve the efficiency of electrokinetic remediation, and therefore, KI solutions are often used. Marrugo Negrete and Lopez Barboza46 used KI solution as a complexing agent to remediate Hg-contaminated soil in a mine. The results show that the removal of Hg from the soil is faster and more efficient by increasing the concentration of the KI solution and increasing the voltage between the two electrodes.46 Reddy et al.70 used different concentrations of KI solution and different voltage gradients to remove Hg from kaolin and moraine. The Hg concentration in kaolin and moraine decreased from 500 mg kg−1 to 16 mg kg−1 and 116 mg kg−1, respectively, and the removal rate reached 97% and 77%, respectively.70
Based on the previous achievements of using acid solution and KI solution for improving the electroremediation, García-Rubio et al.71 analysed the feasibility of electrokinetic remediation by using Hg morphology. Based on morphological analysis experiments, they pointed out that the residual Hg in the soluble weak acid part of the soil increased after KI solution-based electrokinetic remediation. Therefore, the same soil should be treated with acidic electroremediation technology to reduce the possibility of an increased risk related to pollution sites.71 In addition to iodide ions, many studies have shown that electrokinetic remediation can achieve satisfactory efficiency for Hg removal in the presence of EDTA. Robles et al.72 used titanium as an electrode and applied a voltage of 5 V to remove Hg from contaminated soil with EDTA for 6 hours. The experimental results showed that 75% of the metals in the soil could be removed. Further analysis shows that the effective removal of Hg pollution observed in the experiment is due to the electromigration of the coordination complex formed by Hg2+ and a terminal hydroxyl in EDTA.72
4.2. Chemical remediation
Chemical remediation is a method in which chemical reagents, reactions, and principles are used to remove contaminants;73,74 the above processes usually cause pollutants to degrade, which either removes or reduces the soil toxicity. Currently, the main chemical remediation methods include soil washing, chemical stabilization, oxidation, reduction and reduction dechlorination, solvent extraction and soil performance improvement remediation technology.
4.2.1. Soil washing. Soil washing is a remediation technology that leaches contaminants from the soil by using various chemical reagents.45 The reagents used in soil washing can promote the dissolution or migration of pollutants in the soil. In operation, the remediation technique can be divided into two methods: in situ and ex situ leaching. The in situ leaching technology infuses the leaching solution into the polluted soil and then recovers the heavy metals in the filtrate, including the detergent dosing system, the leaching solution collection system under the soil and the leaching solution treatment system.75 In the case of ex situ leaching, the contaminated soil should be excavated and put into the corresponding treatment equipment before cleaning the waste liquid treatment and other processes.75 In general, heterotopic leaching is combined with physical separation technology, and the excavated soil is first screened for soil particles and debris and large particles are removed.In the process of Hg pollution remediation in the soil through soil washing, water-soluble Hg is usually extracted by acidic, alkali or chelating agents. Generally, the use of acidic or alkali agents depends on the dissolution of Hg compounds or the dissolution of Hg-containing components adsorbed by soil components, while chelating agents can remove Hg through complexation.2,76 In terms of chemical elution remediation, the eluent is very important. At present, acidic leaching agents include HCl, HNO3, H2SO4, and H3PO4; salt leaching agents include NaCl, Na2S2O3, and KI; artificial chelating agents include ethylenediaminetetraacetic acid (EDTA), nitrilotriacetic acid (NTA), diethylenetriaminepentaacetic acid (DTPA), ethylenediamine disuccinate (EDDS), and methylglycine-N,N-diacetic acid (MGDA); and natural organic chelating agents include citric acid, malic acid, oxalic acid, malonic acid, humic acid, and fulvic acid.47 In recent years, many scholars have found that different chemical eluents have different removal efficiencies. Subirés-Muñoz et al.77 studied several typical chemical eluents. Their research results show that iodide (e.g., KI), EDTA and thiosulfate (Na2S2O3) can effectively remove Hg from the polluted soil polluted. However, due to the leaching effect of EDTA is affected by a competition with other heavy metal elements in soil, the low concentration of EDTA solution can only obtain a low rate of removal.77 In addition, Jia et al.78 also found that KI, EDTA and thiosulfate (Na2S2O3) have a better removal effect on mercury in the soil compared to citric acid, tartaric acid and sodium dodecyl sulfate, especially Na2S2O3 solution.
4.2.2. Chemical stabilization. Chemical stabilization involves the addition of chemical reagents or chemical materials to immobilize heavy metals in the soil through complexation, precipitation and adsorption reactions, which reduce the mobility and bioavailability of heavy metals.41,45 Chemical stabilization cannot remove or extract pollutants from soil, but it can significantly reduce their mobility/solubility and their concentration in soil pore water, thus reducing the transfer rate of heavy metals to plants, microorganisms, and water.79,80 Previous results showed that the bioavailability, mobility, toxicity, and potential risk of heavy metals are affected by their form, not by their total concentration in environment.81,82 Thus, different forms of heavy metals have different environmental effects.83 Similarly, the mobility and bioavailability of Hg also depend on its distribution in soil. Based on this, some materials can be used to immobilize Hg and herein reduce the bioavailability of Hg in the soil.HgS is a very insoluble sulfide compound that is easy to form and very stable under reduction conditions. William et al.84 pointed out that the low solubility and inactive redox reaction characteristics of HgS enable Hg to exist in a stable and harmless form and show no propensity to volatilize. When Hg exists in the oxidized form of Hg2+, the introduction of sulfide as a stabilizer into the medium can make Hg2+ form stable HgS and reduce the concentration of Hg2+; in some reaction processes, Hg is first oxidized to Hg2+ by a strong oxidizing acid (such as nitric acid) and then precipitated to form HgS.85 Currently, many experts have used sulfide as a chemical fixative for remediation of Hg pollution in soil. Some studies have shown that sulfide minerals have high affinity with Hg and herein have great thermodynamic potential in fixing Hg.48,86 It is considered to be one of the most effective adsorption materials for Hg(II). Among them, the most common sulfide minerals are iron sulfide, such as pyrite, magnetite and tetragonal pyrite.48 Liu et al.87 studied the mechanism of FeS adsorption and the stabilization of water-soluble Hg(II) through batch adsorption experiments of HgCl2 and synthetic FeS under anoxic conditions. The results showed that FeS could effectively fix Hg(II) from solution because Hg(II) had lower solubility than FeS. This reaction process includes coprecipitation and adsorption, although precipitation is the main factor and accounts for 77% of the overall remediation.87
To improve the remediation performance of sulfide ore, many experts have combined chemical stabilization with nanotechnology to prepare sulfide ore nanoparticles and obtain more efficient stabilizers. Xiong et al.88 successfully synthesized FeS nanomaterials with carboxymethylcellulose as a stabilizer and explored the performance of FeS nanomaterials for mercury stabilization through a series of adsorption and dynamic column experiments. The adsorption experiments show that iron sulfide nanoparticles can effectively fix Hg in sediment and significantly reduce the concentration of water-soluble Hg; moreover, morphology analysis of Hg shows that FeS nanoparticles can greatly reduce the amount of bioavailable Hg.88
Since then, some experts have further explored the feasibility of carboxymethylcellulose (CMC)–FeS nanomaterials for use in Hg removal and have found that CMC–FeS nanomaterials have excellent stability and a strong affinity for Hg. Through batch and column experiments, Gong et al.89 indicated that when the molar ratio between CMC and FeS is controlled at an appropriate proportion, it can effectively immobilize Hg in sediment/soil. Further, adsorption experiments showed that a low pH, high concentrations of Cl− and organic matter can inhibit the adsorption of Hg in solution.90 According to previous studies, FeS is an effective fixative for Hg pollution in soil, and the FeS nanomaterial modified with CMC as a stabilizer has a better adsorption effect.
Apart from sulfide ores such as pyrite, many researchers also use sodium sulfide as a fixative. Devasena and Nambi91 performed a series of experiments, and the results showed that the polysulfide solution had a good in situ immobilization effect on the entrapped Hg0. Wei and Liu92 also showed that Na2S was a good stabilizer that had a highly stable efficiency and could effectively reduce the leaching toxicity of Hg compared with FeS, Na2S, pyrite, CaO, or their joint use.
In addition, some experts have explored other materials, such as basic materials, biochar, activated carbon, phosphate, ferromanganese oxide materials, layered silicate minerals and organic matter as fixatives. For example, Xie et al.49 showed that a mixture of bentonite and diammonium hydrogen phosphate can significantly reduce both the effective Hg in soil and the amount of Hg in iron-manganese oxide in the field. Furthermore, the amount of Hg in the aboveground part and root system of a Chinese cabbage planted in a treated farmland also significantly decreased.
4.3. Biological remediation
Biological remediation/bioremediation is a method that reduces, removes or immobilizes harmful contaminants in the soil and purifies the soil by using biologicals.93 Bioremediation is mainly divided into two categories: plant remediation and microbial remediation in which the development of plant remediation has received much more attention.
4.3.1. Phytoremediation. Phytoremediation is the application of plants and their associated rhizospheric microorganisms to remove, degrade or immobilize pollutants in soils, as well as in sediments, underground and surface water.50 Phytoremediation is recognized as a green technology with good public perception and is widely used for the removal of pollutants, particularly heavy metals.74 According to different remediation mechanisms, the phytoremediation of Hg-contaminated soil mainly includes phytostabilization, phytoextraction, phytotransformation (organics), phytovolatilization and rhizofiltration.94 Fig. 4 shows the mechanisms of phytoremediation. Different plant properties are used in different phytoremediation technologies.50 When phytoremediation technology is applied to the remediation of Hg-contaminated soil, Hg is transported from the roots to the aboveground part of the plant through a relevant organizational structure of the plant after the plant roots absorb Hg. During the whole process of absorption and transportation, Hg will interact with each part of the plant to some degree, and some specific plants can remove or fix Hg in the soil through these actions for soil remediation.
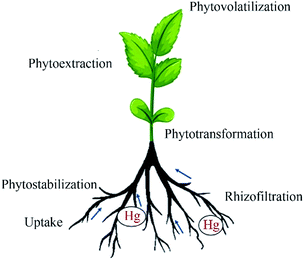 |
| Fig. 4 Mechanisms of phytoremediation.95 | |
Phytostabilization mainly uses the roots of plants to limit contaminant mobility and bioavailability in the soil and occurs by way of biochemical processes that occur in roots or within the root neighbourhood.5 Thus, the bioavailability and mobility of Hg in the soil can be reduced through the absorption and accumulation of Hg by plant roots or the interaction between Hg and plant rhizosphere substances. Phytostabilization remediates contaminated soil mainly through adsorption, precipitation, complexation or metal valence reduction.94 In essence, phytostabilization technology does not reduce the amount of Hg in the soil; it only changes the form of Hg to accumulate and precipitate the Hg in the roots, which reduces the mobility of Hg in the soil.
According to the mechanism of immobilization, the plants generally hold the characteristics of a developed root system, high Hg tolerance, and low transport coefficient. Experimental results show that willow is a suitable plant to remediate Hg-contaminated soil. First, Hg has a low transport coefficient in willow, and the Hg absorbed by its root system does not transfer to the branches and leaves; second, willow can fix bioavailable Hg in the soil through the root system, and with its growth, the amount of exchangeable Hg in the soil also decreases.96 Greger et al.97 also pointed out that there was no risk of Hg being re-released to the atmosphere through the stomata of leaves by using willow as a remediation plant because Hg absorbed by willow roots can only transfer a small amount to the branches and leaves. Recent studies have shown that Jerusalem artichoke is also a plant that has the potential to restore mercury contaminated soil, and different Jerusalem artichoke plants have different Hg tolerances.98
Phytoextraction refers to the use of plants to uptake metals from the soil through roots and translocate them to the aboveground parts of the plant; then, the metals are recovered from the harvestable parts of the plants.57,99 According to this principle of remediation, the plants selected for this technology are usually hyperaccumulator plants. Hyperaccumulator plants can absorb heavy metals and transport them to the aboveground parts. Generally, they have characteristics of accumulating and storing heavy metals at concentrations many times greater than non-hyperaccumulators growing in the same habitat.51
Zhao et al.100 found that the Miscanthus sinensis plant was tolerant at high levels of Hg exposure in soil and grew even if the Hg concentration in the soil is up to 706 mg kg−1. When growing in soil with Hg at a level of 183 mg kg−1, the Hg bioconcentration and translocation factors of Miscanthus sinensis are close to or greater than 1. Thus, Miscanthus sinensis is deemed to be a potential phytoremediator. In addition, Xun et al.101 found that Cyrtomium macrophyllum, which grows in polluted soil of a mining area, is a potential Hg accumulating plant with a transport factor of 2.62 and a Hg concentration of 36.44 mg kg−1 accumulated in the aboveground part. It has a good Hg accumulation and transport capacity. To date, there are few reports about Hg-enriched plants, and screening these plants is still in progress. Therefore, many experts have studied the use of other plants to complete the extraction and remediation of Hg-contaminated soil. Marrugo-Negrete et al.102 conducted an experimental study on the extraction of Hg with Jatropha curcas. According to the results, although the concentration of the aboveground part of Jatropha curcas is relatively low, it can grow in soil with high Hg concentrations and remediate it. Therefore, Jatropha curcas is a good choice to remediate Hg-contaminated soil.
Phytovolatilization refers to the use of plants to take up contaminants from the soil, converting them into volatile forms and releasing them into the atmosphere.94 In short, this process is the volatilization of pollutants or metabolites by using plants.103 Phytovolatilization mainly removes some volatile pollutants in soil. Based on the volatility of Hg, this technology can be used to remediate Hg-contaminated soil. The Hg in the soil is absorbed and transformed through the plants and then volatilized to the atmosphere in the form of gaseous Hg. This technology is usually combined with genetic engineering. The Hg invertase gene is introduced into a target plant by genetic engineering so that the plant can convert the combined Hg into zero-valent Hg and release it into the atmosphere through the leaves.104 Two genes, merA and merB, are related to Hg accumulation and transformation. When these two genes are expressed in plants, MeHg can be transformed into elemental Hg and then evaporated into the atmosphere through the leaves.105,106 However, plant volatilization transfers Hg from the soil to the atmosphere. The potential risk of atmospheric Hg pollution has not been solved. Therefore, plant volatilization has not been widely used in practical remediation.
4.3.2. Microbial remediation. Microbes are ubiquitous in the environment and have a superior effect in heavy metal-contaminated sites, so they play a vital role in converting toxic heavy metals into nontoxic forms.107 Microorganisms that are resistant to metals can be used as bioremediation agents.108 Previous studies have shown that microorganisms can restore the environment through a variety of methods, such as “binding, immobilization, oxidation, transformation, and volatilization of heavy metals”.107 Studies have found that Hg-tolerant microorganisms with a mer operon can effectively control Hg pollution in the environment.109,110 There are some mer operons in the genome of Hg-resistant bacteria, including certain functional genes as well as promoters, regulatory genes, and operators.111 The most common functional genes are merA and merB, which encode Hg-ion reductase and organic Hg lyase. With the help of reductase, lyase can reduce highly toxic organic Hg compounds to almost nontoxic volatile Hg.111 In addition, Dash et al.112 isolated Bacillus thuringiensis PW-05 from the coast of Orissa, which has a mer operon similar to most Hg-resistant bacteria. At the same time, real-time PCR monitoring and AAS studies confirm that the bacteria have the potential for Hg bioremediation. Mahbub et al.113 found that strain SE2 was isolated from Hg-contaminated soil and had a high Hg resistance, which confirmed that the strain could be used for Hg remediation through Hg reductase and qualitative detection of volatile Hg. In addition, Paenibacillus sp. have also been shown to have the potential for Hg remediation.53Currently, many Hg-resistant bacteria with the ability of Hg remediation have been found and confirmed. With the development of further research, an increasing number of microorganisms will be found one by one, and microbial remediation will develop into a promising Hg-remediation method. Moreover, with the development of modern genetic engineering, microbial remediation is more practical. Mahbub et al.114 indicated that because polluted soil may include heavy metal pollution other than Hg, isolate or genetically modify and characterize are needed to obtain strains which can tolerate a variety of heavy metals.
However, the method of using bacterial volatilization to remediate Hg pollution needs to be improved because most of the Hg in the soil is combined with soil particles. To efficiently use microorganisms for volatilization remediation of Hg in soil, Chen et al.115 developed a two-stage system: chemical extraction followed by microbial reduction. The experimental results show that ammonium thiosulfate is a good extraction agent and does not inhibit bacterial growth. Therefore, a combination of chemical extraction and bioremediation is a useful remediation technique.
5. Conclusions and future perspectives
Although the main source of Hg pollution in soil come from natural release, other pollution sources, especially human activities, such as industrial and agricultural activities, further aggravate Hg pollution in soil. Mining, metal smelting, fossil fuel combustion, pesticide application and sewage irrigation significantly lead to an accumulation of Hg in soil. To better understand and explore more effective treatment methods for Hg-contaminated soil, we conclude the recent development of soil remediation methods, which include thermal desorption, electrokinetic remediation, soil washing, chemical stabilization, plant remediation and microbial remediation.
When specific remediation projects are carried out, we should select appropriate remediation methods depending on their advantages and disadvantages and the types of soil. For example, agricultural soil remediation should carefully use thermal desorption because too high of a thermal decomposition temperature will destroy the organic matter in soil, change the soil structure, and even become inappropriate for use as agricultural land. When soil washing and chemical stabilization are used, secondary pollution of chemical reagents should be prevented. In essence, chemical stabilization only changes the form of Hg in the soil; therefore, considering that different reagents have different reaction times, it is necessary to monitor the whole remediation process and add reagents in time to ensure that the remediation is in an effective state. Although phytoremediation has received much more attention among various remediation methods, effective hyperaccumulator plants for Hg have not been found.
With the development of science and technology, various methods of remediation have been greatly developed. Recently, various emerging materials, such as nanomaterials (carbon nanotubes, nanosheets and magnetic nanocomposites), graphene, biochar and other new materials have become the research hotspots. In addition, genetic engineering technology is also gradually applied to remediation. Usually, plants with an ability of hyperaccumulation are generally short, and most tall plants do not have the ability of hyperaccumulation. To our surprise, combining with genetic engineering, phytoremediation has become the most promising remediation technology for Hg-contaminated soil. Moreover, genetic engineering is also beneficial to the development of microbial remediation technology. Overall, future requirements for remediation technology that will be used for Hg pollution in soil should attempt to be economical and easy to operate and take into account the disposal of Hg-bearing remediation residues.
Conflicts of interest
There are no conflicts to declare.
Acknowledgements
This work is supported by the National Science Foundation of China-Project of Karst Science Research Center (U1612442), Financial support from National Key Research and Development Program of China (No. 2019 YFC1803601), Science and Technology Program of Guizhou Province [Grant No. (2019)5618, 2019(2963), and (2019)4428], and Opening fund of State Key Laboratory of Environmental Geochemistry (SKLEG2019606, 2019717). Dr Nabeel Khan Niazi is thankful to University of Agriculture Faisalabad, Pakistan.
References
- L. Chen, S. Liang, M. Liu, Y. Yi, Z. Mi, Y. Zhang, Y. Li, J. Qi, J. Meng, X. Tang, H. Zhang, Y. Tong, W. Zhang, X. Wang, J. Shu and Z. Yang, Nat. Commun., 2019, 10, 1484 CrossRef.
- J. Xu, A. G. Bravo, A. Lagerkvist, S. Bertilsson, R. Sjoblom and J. Kumpiene, Environ. Int., 2015, 74, 42–53 CrossRef CAS PubMed.
- M. Sakamoto, T. Itai, K. Marumoto, M. Marumoto, H. Kodamatani, T. Tomiyasu, H. Nagasaka, K. Mori, A. J. Poulain, J. L. Domingo, M. Horvat and A. Matsuyama, Environ. Res., 2020, 180 Search PubMed.
- J. Yan, C. Wang, Z. Wang, S. Yang and P. Li, Appl. Geochem., 2019, 106, 112–119 CrossRef CAS.
- J. Wang, X. Feng, C. W. Anderson, Y. Xing and L. Shang, J. Hazard. Mater., 2012, 221–222, 1–18 CAS.
- UNEP, United Nations Environment Programme, Geneva, 2018 Search PubMed.
- W. C. Li and H. F. Tse, Environ. Sci. Pollut. Res., 2015, 22, 192–201 CrossRef CAS PubMed.
- H. B. Zhang, Y. Li, Y. M. Luo and P. Christie, J. Soils Sediments, 2015, 15, 962–971 CrossRef CAS.
- P. Li, X. B. Feng, G. L. Qiu, L. H. Shang and Z. G. Li, J. Hazard. Mater., 2009, 168, 591–601 CrossRef CAS.
- L. Wang, D. Hou, Y. Cao, Y. S. Ok, F. M. G. Tack, J. Rinklebe and D. O'Connor, Environ. Int., 2020, 134, 105281 CrossRef CAS PubMed.
- D. Raj, A. Kumar and S. K. Maiti, Chemosphere, 2020, 248, 125857 CrossRef CAS PubMed.
- G. Lu, C. Yue, G. Qiu, M. Guo and M. Zhang, J. Chem. Eng. Jpn., 2017, 50, 155–160 CrossRef CAS.
- Y. Ting and H.-C. Hsi, Sustainability, 2019, 11 Search PubMed.
- A. M. Kunkel, J. J. Seibert, L. J. Elliott, R. Kelley, L. E. Katz and G. A. Pope, Environ. Sci. Technol., 2006, 40, 2384–2389 CrossRef CAS PubMed.
- F. He, J. Gao, E. Pierce, P. J. Strong, H. Wang and L. Liang, Environ. Sci. Pollut. Res. Int., 2015, 22, 8124–8147 CrossRef CAS PubMed.
- I. Al-Saleh, M. Nester, M. Abduljabbar, R. Al-Rouqi, C. Eltabache, T. Al-Rajudi and R. Elkhatib, Int. J. Hyg. Environ. Health, 2016, 219, 129–141 CrossRef CAS PubMed.
- D. Raj and S. K. Maiti, Environ. Monit. Assess., 2019, 191 Search PubMed.
- M. Wang, Y. Li, D. Zhao, L. Zhuang, G. Yang and Y. Gong, Chem. Eng. J., 2020, 381 Search PubMed.
- T. Devisscher, T. Hayden and J. Jabbour, in UNEP YEAR BOOK 2009, February 2009 Search PubMed.
- G. Bjorklund, M. Dadar, J. Mutter and J. Aaseth, Environ. Res., 2017, 159, 545–554 CrossRef PubMed.
- W. Zhang, G. Zhen, L. Chen, H. Wang, Y. Li, X. Ye, Y. Tong, Y. Zhu and X. Wang, Energy Policy, 2017, 106, 579–587 CrossRef.
- R. Soler-Blasco, M. Murcia, M. Lozano, X. Aguinagalde, G. Iriarte, M.-J. Lopez-Espinosa, J. Vioque, C. Iniguez, F. Ballester and S. Llop, Environ. Int., 2019, 130 Search PubMed.
- W. Zhang, X. Zhang, Y. Tian, Y. Zhu, Y. Tong, Y. Li and X. Wang, J. Hazard. Mater., 2018, 354, 198–205 CrossRef CAS PubMed.
- E. Ha, N. Basu, S. Bose-O'Reilly, J. G. Dorea, E. McSorley, M. Sakamoto and H. M. Chan, Environ. Res., 2017, 152, 419–433 CrossRef CAS PubMed.
- G. B. Jiang, J. B. Shi and X. B. Feng, Environ. Sci. Technol., 2006, 40, 3672–3678 CrossRef CAS PubMed.
- D. Obrist, J. L. Kirk, L. Zhang, E. M. Sunderland, M. Jiskra and N. E. Selin, Ambio, 2018, 47, 116–140 CrossRef PubMed.
- S. X. Wang, L. Zhang, L. Wang, Q. R. Wu, F. Y. Wang and J. M. Hao, Front. Environ. Sci. Eng., 2014, 8, 631–649 CrossRef CAS.
- H. Zhang, X. Feng, T. Larssen, G. Qiu and R. D. Vogt, Environ. Health Perspect., 2010, 118, 1183–1188 CrossRef CAS PubMed.
- X. Feng and G. Qiu, Sci. Total Environ., 2008, 400, 227–237 CrossRef CAS PubMed.
- P. Li, X. Feng, G. Qiu, L. Shang and G. Li, Environ. Geochem. Health, 2009, 31, 683–691 CrossRef CAS PubMed.
- X. Xu, Y. Lin, B. Meng, X. Feng, Z. Xu, Y. Jiang, W. Zhong, Y. Hu and G. Qiu, Appl. Geochem., 2018, 88, 267–275 CrossRef CAS.
- X. B. Feng, G. H. Li and G. L. Qiu, Atmos. Environ., 2004, 38, 6223–6230 CrossRef CAS.
- G. Li, X. Feng, G. Qiu, X. Bi, Z. Li, C. Zhang, D. Wang, L. Shang and Y. Guo, Environ. Pollut., 2008, 154, 21–31 CrossRef CAS PubMed.
- X. Feng, G. Li and G. Qiu, Sci. Total Environ., 2006, 368, 47–55 CrossRef CAS PubMed.
- N. Zheng, J. Liu, Q. Wang and Z. Liang, Appl. Geochem., 2011, 26, 188–193 CrossRef CAS.
- Z. Li, X. Feng, G. Li, X. Bi, G. Sun, J. Zhu, H. Qin and J. Wang, Appl. Geochem., 2011, 26, 160–166 CrossRef CAS.
- J. Ali, T. G. Kazi, H. I. Afridi, J. A. Baig, M. S. Arain, Naeemullah and S. Farooq, Int. J. Coal Geol., 2016, 156, 50–58 CrossRef CAS.
- J. Ali, T. G. Kazi, M. Tuzen and N. Ullah, Environ. Sci. Pollut. Res., 2017, 24, 17731–17740 CrossRef CAS PubMed.
- X. Yang and L. Wang, Environ. Geol., 2008, 53, 1381–1388 CrossRef CAS.
- G. N. Koptsik, Eurasian Soil Sci., 2014, 47, 707–722 CrossRef CAS.
- S. Khalid, M. Shahid, N. K. Niazi, B. Murtaza, I. Bibi and C. Dumat, J. Geochem. Explor., 2017, 182, 247–268 CrossRef CAS.
- Z. D. Nejad, M. C. Jung and K.-H. Kim, Environ. Geochem. Health, 2018, 40, 927–953 CrossRef.
- J. T. Albergaria, M. da Conceicao, M. Alvim-Ferraz and C. Delerue-Matos, Environ. Pollut., 2006, 143, 146–152 CrossRef CAS PubMed.
- T. Zhao, X. Wang, X. Yang and X. Yan, Res. J. Environ. Sci., 2015, 28, 425–430 CAS.
- Y. Y. Gong, D. Y. Zhao and Q. L. Wang, Water Res., 2018, 147, 440–460 CrossRef CAS PubMed.
- J. L. Marrugo Negrete and E. Lopez Barboza, Rev. Fac. Ing., Univ. Antioquia, 2013, 136–146 Search PubMed.
- L. Yi, Y. Tao, Y. Liu, W. Wang, J. Wen and J. Xiao, J. Saf. Environ., 2012, 12, 42–46 CAS.
- J. Bower, K. S. Savage, B. Weinman, M. O. Barnett, W. P. Hamilton and W. F. Harper, Environ. Pollut., 2008, 156, 504–514 CrossRef CAS PubMed.
- Y. Xie, X. Feng and J. Wang, Chinese J. Ecol., 2014, 33, 1935–1939 CAS.
- A. Marques, A. Rangel and P. M. L. Castro, Crit. Rev. Environ. Sci. Technol., 2009, 39, 622–654 CrossRef CAS.
- S. A. Asad, M. Farooq, A. Afzal and H. West, Chemosphere, 2019, 217, 925–941 CrossRef CAS PubMed.
- S. J. Ye, G. M. Zeng, H. P. Wu, C. Zhang, J. Dai, J. Liang, J. F. Yu, X. Y. Ren, H. Yi, M. Cheng and C. Zhang, Crit. Rev. Biotechnol., 2017, 37, 1062–1076 CrossRef CAS PubMed.
- J. Szakova, J. Havlickova, A. Sipkova, J. Gabriel, K. Svec, P. Baldrian, J. Sysalova, P. Coufalik, R. Cervenka, O. Zverina, J. Komarek and P. Tlustos, J. Environ. Sci. Health, Part A: Toxic/Hazard. Subst. Environ. Eng., 2016, 51, 364–370 CrossRef CAS PubMed.
- Y. Gao and Y. Luo, Acta Pedol. Sin., 2005, 42, 140–148 Search PubMed.
- L. Wang, C. Yan, H. Wang and X. Zhang, Biotechnol. Bull., 2016, 32, 51–58 CAS.
- C. Zhao, Y. Dong, Y. P. Feng, Y. Z. Li and Y. Dong, Chemosphere, 2019, 221, 841–855 CrossRef CAS PubMed.
- C. Li, K. Zhou, W. Qin, C. Tian, M. Qi, X. Yan and W. Han, Soil Sediment Contam., 2019, 28, 380–394 CrossRef CAS.
- M. Rumayor, M. Diaz-Somoano, M. A. Lopez-Anton and M. R. Martinez-Tarazona, Talanta, 2013, 114, 318–322 CrossRef CAS PubMed.
- M. Hempel and J. Thoeming, in Mercury Contaminated Sites: Characterization, Risk Assessment and Remediation, 1999, pp. 113–130 Search PubMed.
- A. Navarro, I. Canadas, D. Martinez, J. Rodriguez and J. L. Mendoza, Sol. Energy, 2009, 83, 1405–1414 CrossRef CAS.
- M. Rumayor, J. R. Gallego, E. Rodriguez-Valdes and M. Diaz-Somoano, J. Hazard. Mater., 2017, 325, 1–7 CrossRef CAS PubMed.
- R. Kucharski, U. Zielonka, A. Sas-Nowosielska, J. M. Kuperberg, A. Worsztynowicz and J. Szdzuj, Environ. Monit. Assess., 2005, 104, 341–351 CrossRef CAS PubMed.
- T. C. Chang and J. H. Yen, J. Hazard. Mater., 2006, 128, 208–217 CrossRef CAS PubMed.
- M. J. Sierra, R. Millan, F. A. Lopez, F. J. Alguacil and I. Canadas, Environ. Sci. Pollut. Res., 2016, 23, 4898–4907 CrossRef CAS PubMed.
- C. Comuzzi, B. Lesa, E. Aneggi, G. Dolcetti and D. Goi, J. Hazard. Mater., 2011, 193, 177–182 CrossRef CAS PubMed.
- F. Ma, C. Peng, D. Hou, B. Wu, Q. Zhang, F. Li and Q. Gu, J. Hazard. Mater., 2015, 300, 546–552 CrossRef CAS PubMed.
- D. Rosestolato, R. Bagatin and S. Ferro, Chem. Eng. J., 2015, 264, 16–23 CrossRef CAS.
- Z. Yao, J. Li, H. Xie and C. Yu, Procedia Environ. Sci., 2012, 16, 722–729 CrossRef CAS.
- W. nui, M. A. Jianwei, F. A. N. Xiangyu and L. U. O. Qishi, Ecol. Environ., 2007, 16, 223–227 Search PubMed.
- K. R. Reddy, C. Chaparro and R. E. Saichek, J. Environ. Eng., 2003, 129, 1137–1148 CrossRef CAS.
- A. García-Rubio, J. M. Rodríguez-Maroto, C. Gómez-Lahoz, F. García-Herruzo and C. Vereda-Alonso, Electrochim. Acta, 2011, 56, 9303–9310 CrossRef.
- I. Robles, M. G. Garcia, S. Solis, G. Hernandez, Y. Bandala, E. Juaristi and E. Bustos, Int. J. Electrochem. Sci., 2012, 7, 2276–2287 CAS.
- W. J. Ma and B. Q. Jiao, Res. J. Chem. Environ., 2012, 16, 137–139 Search PubMed.
- B. Song, G. Zeng, J. Gong, J. Liang, P. Xu, Z. Liu, Y. Zhang, C. Zhang, M. Cheng, Y. Liu, S. Ye, H. Yi and X. Ren, Environ. Int., 2017, 105, 43–55 CrossRef CAS PubMed.
- Y. Yu and Q. Zhou, Tech. Equip. Environ. Pollut. Control, 2005, 6, 1–7 CAS.
- G. Dermont, M. Bergeron, G. Mercier and M. Richer-Lafleche, J. Hazard. Mater., 2008, 152, 1–31 CrossRef CAS PubMed.
- J. D. Subirés-Muñoz, A. García-Rubio, C. Vereda-Alonso, C. Gómez-Lahoz, J. M. Rodríguez-Maroto, F. García-Herruzo and J. M. Paz-García, Sep. Purif. Technol., 2011, 79, 151–156 CrossRef.
- J. Jia, Y. Huang, F. Liu, W. Shi, C. Hou and Y. Teng, Environ. Prot. Chem. Ind., 2018, 38, 231–235 Search PubMed.
- S. A. A. Tajudin, M. A. M. Azmi and A. T. A. Nabila, IOP Conf. Ser.: Mater. Sci. Eng., 2016, 136, 012043 Search PubMed.
- L. Liu, W. Li, W. Song and M. Guo, Sci. Total Environ., 2018, 633, 206–219 CrossRef CAS PubMed.
- B. A. O. Zhengduo, W. Jianxu, F. Xinbin and S. Lihai, Chinese J. Ecol., 2011, 30, 907–913 Search PubMed.
- K. Fytianos, J. AOAC Int., 2001, 84, 1763–1769 CrossRef CAS PubMed.
- X. Wang and Y. Yang, Environ. Prot. Chem. Ind., 2004, 24, 24–28 CAS.
- W. P. Hamilton and A. R. Bowers, Waste Manag., 1997, 17, 25–32 CrossRef CAS.
- S. Hagemann, Technologies for the stabilization of elemental mercury and mercury-containing wastes, 2009 Search PubMed.
- J. R. Brown, G. M. Bancroft, W. S. Fyfe and R. A. N. McLean, Environ. Sci. Technol., 1979, 13, 1142–1144 CrossRef CAS.
- J. Liu, K. T. Valsaraj, I. Devai and R. D. DeLaune, J. Hazard. Mater., 2008, 157, 432–440 CrossRef CAS PubMed.
- Z. Xiong, F. He, D. Zhao and M. O. Barnett, Water Res., 2009, 43, 5171–5179 CrossRef CAS PubMed.
- Y. Gong, Y. Liu, Z. Xiong, D. Kaback and D. Zhao, Nanotechnol, 2012, 23, 294007 CrossRef PubMed.
- Y. Gong, Y. Liu, Z. Xiong and D. Zhao, Environ. Sci. Technol., 2014, 48, 3986–3994 CrossRef CAS PubMed.
- M. Devasena and I. M. Nambi, J. Environ. Manage., 2013, 130, 185–191 CrossRef CAS PubMed.
- Y. Wei and Y. Liu, Chin. J. Environ. Eng., 2017, 11, 1878–1884 Search PubMed.
- G. Lu, C. Yue, B. Peng, G. Qiu, M. Guo and M. Zhang, Chin. J. Eng., 2017, 39, 1–12 Search PubMed.
- M. Ghosh and S. P. Singh, Appl. Ecol. Environ. Res., 2005, 3, 1–18 CrossRef.
- S. Ashraf, Q. Ali, Z. A. Zahir, S. Ashraf and H. N. Asghar, Ecotoxicol. Environ. Saf., 2019, 174, 714–727 CrossRef CAS.
- Y. Wang, C. Stauffer, C. Keller and M. Greger, Plant Soil, 2005, 275, 67–75 CrossRef CAS.
- M. Greger, Y. Wang and C. Neuschutz, Environ. Pollut., 2005, 134, 201–208 CrossRef CAS PubMed.
- S. Lv, B. Yang, Y. Kou, J. Zeng, R. Wang, Y. Xiao, F. Li, Y. Lu, Y. Mu and C. Zhao, PeerJ, 2018, 6, e4325 CrossRef PubMed.
- D. Mani and C. Kumar, Int. J. Environ. Sci. Technol., 2014, 11, 843–872 CrossRef CAS.
- A. Zhao, L. Gao, B. Chen and L. Feng, Environ. Sci. Pollut. Res., 2019, 26, 34818–34829 CrossRef CAS PubMed.
- Y. Xun, L. Feng, Y. Li and H. Dong, Chemosphere, 2017, 189, 161–170 CrossRef CAS PubMed.
- J. Marrugo-Negrete, J. Durango-Hernandez, J. Pinedo-Hernandez, J. Olivero-Verbel and S. Diez, Chemosphere, 2015, 127, 58–63 CrossRef CAS.
- C. U. Emenike, B. Jayanthi, P. Agamuthu and S. H. Fauziah, Environ. Rev., 2018, 26, 156–168 CrossRef CAS.
- Z. Liu, K. Li, Z. Xu, J. Li and S. Lu, in Proceedings of the 2014 International Conference on Mechatronics, Electronic, Industrial and Control Engineering, ed. L. Chang, C. Guiran and L. Zhen, 2014, vol. 5, p. 1586 Search PubMed.
- S. P. Bizily, C. L. Rugh, A. O. Summers and R. B. Meagher, Proc. Natl. Acad. Sci. U. S. A., 1999, 96, 6808–6813 CrossRef CAS PubMed.
- R. B. Meagher, Curr. Opin. Plant Biol., 2000, 3, 435 CrossRef.
- S. Verma and A. Kuila, Environ. Technol. Innov., 2019, 14 Search PubMed.
- P. Prabhakaran, M. A. Ashraf and W. S. Aqma, RSC Adv., 2016, 6, 109862–109877 RSC.
- A. M. M. Essa, L. E. Macaskie and N. L. Brown, Biochem. Soc. Trans., 2002, 30, 672–674 CrossRef CAS PubMed.
- A. M. A. Nascimento and E. Chartone-Souza, Genet. Mol. Res., 2003, 2, 92–101 Search PubMed.
- H. R. Dash and S. Das, Int. Biodeterior. Biodegrad., 2012, 75, 207–213 CrossRef CAS.
- H. R. Dash, N. Mangwani and S. Das, Environ. Sci. Pollut. Res., 2014, 21, 2642–2653 CrossRef CAS PubMed.
- K. R. Mahbub, K. Krishnan, R. Naidu and M. Megharaj, J. Environ. Sci., 2017, 51, 128–137 CrossRef PubMed.
- K. R. Mahbub, M. M. Bahar, M. Labbate, K. Krishnan, S. Andrews, R. Naidu and M. Megharaj, Appl. Microbiol. Biotechnol., 2017, 101, 963–976 CrossRef CAS PubMed.
- S. C. Chen, W. H. Lin, C. C. Chien, D. C. W. Tsang and C. M. Kao, Chemosphere, 2018, 200, 266–273 CrossRef CAS PubMed.
|
This journal is © The Royal Society of Chemistry 2020 |
Click here to see how this site uses Cookies. View our privacy policy here.