DOI:
10.1039/D0RA02195D
(Paper)
RSC Adv., 2020,
10, 23080-23090
Terpyridine-based Pd(II)/Ni(II) organometallic framework nano-sheets supported on graphene oxide—investigating the fabrication, tuning of catalytic properties and synergetic effects†
Received
9th March 2020
, Accepted 1st June 2020
First published on 17th June 2020
Abstract
Tailoring the structures of catalysts and the arrangement of organic bimetallic catalysts are essential in both fundamental research and applications. However, they still impose enormous challenges such as size and active species distribution, ordered uniformity, and controllable composition, which are critical in determining their specific activities and efficiency. Herein, a novel terpyridine-based hetero-bimetallic Ni/Pd nanosheet supported on graphene oxide (denoted as GO@Tpy-Ni/Pd) was fabricated, which exhibited higher catalytic activity, substrate applicability and recyclability for the Suzuki coupling reaction under mild conditions. The catalytic mechanism was heterogeneous catalysis at the interface and the synergetic effect between Pd and Ni resulted in a little Ni(0)/Pd(0) cluster including Pd(II)/Ni(II) as a whole being formed through electron transfer on the catalytic surface. This phenomenon could be interpreted as the nanoscale clusters of Ni/Pd being the real active centre stabilized by the ligand and GO and the synergetic effect. The absorption and desorption of different substrates and products on Ni/Pd clusters, as calculated by DFT, was proved to be another key factor.
1. Introduction
Transition-metal-catalyzed cross-couplings have become truly fundamental tools for organic synthesis.1–3 Hetero-multi-metallic catalysts show new performances and capabilities due to the synergistic effects between the metals.4–6 In the last few decades, many hetero-bimetallic nanostructures, including nickel-based, nanoparticles, were widely used in industrial processes owing to their conspicuous activity and outstanding recyclability.7–15 These nanoparticles, with high catalytic activity, were also the most disposed to aggregation, which affected their catalytic performance.16 To solve this problem, appropriate supports and ligands, such as carbon materials and other materials, could strongly prevent metal species from aggregating.17 Nevertheless, practical supported hetero-multi-metallic nanocatalysts did not have well-distributed states that could reduce the catalytic efficiency and the emergence of undesired side reactions.16 It also made it extremely difficult to control the ratio and electron distribution, which were essential for enhancing catalytic activity.18 Therefore, understanding the fundamental mechanisms involved in how to form uniform active centres, why each component interacted and what was the optimized combination for deeply affecting the overall properties became more important research.19,20 It was also very important for the metal catalysts to be supported, since nano-particles of catalysts only having very weak interaction with supports might easily aggregate and leach, resulting in degrading performance. Covalent attachment was a very effective method for anchoring catalytic active centres.21,22 Therefore, the rational fabrication of the ordered self-assembled (SAM) catalytic monolayer and its functionality during catalysis must be investigated deeply,23 including how to identify every element, ensure the proper composition, and produce accurate and stable nano-catalysts.
Self-assembly (SAM) offers customized design, controllable orientation, ease of recovery and stable monolayers. It also has the advantages of homogeneity and heterogeneity through the ingenious design of single-molecular structures.24 Previous studies have shown that the catalytic activity of the hetero-bimetallic catalysts could be enhanced by tuning their composition, morphology and the relative distributions of electrons, which are related to the electrical characteristics of the ligand, supports, and the synergy of hetero-bimetals.25–39 Appropriate supports could promise a high dispersion of metallic catalyst and the maximum utilization of noble materials has attracted much attention;19,40 graphene oxide (GO) has been recognized as an ideal candidate for supporting various metals and their complexes.41–43 Pd catalyst doped with Ni, Fe, Co or Cu, reduces the loading of Pd and significantly enhances catalytic activity by adjusting the distance between nickel and palladium, charge distribution and the arrangement of active sites.34,36,44 Palladium was selected because of its efficient catalytic activity for Suzuki cross-coupling. However, its application was restricted by the limited reserves that were conventionally challenging for Pd catalysts.2 Nickel shares common chemical features and outer shell electron distribution with palladium.45,46 Cross-coupling reactions catalyzed by nickel have recently been attracting significant attention because of the lower toxicity, lower cost and greater nucleophilicity due to the smaller size of nickel as compared with Pd, which is critical for the Suzuki coupling reaction. Nickel cannot be simply considered to be a substitute for palladium since it possesses distinctive catalytic properties that palladium does not have.47,48 However, Ni is sensitive to solvent, a greater amount is needed, and severe catalytic conditions are usually required.49–65 The combination of the advantages of Pd and Ni for catalyzing the coupling reaction has recently attracted tremendous attention.66 The design of ordered, more cost-effective and eco-friendly forms to adjust catalytic properties and elucidate synergy is greatly needed.67–69
In this paper, the terpyridine group was selected as the binding site for palladium and nickel. The character of the terpyridine linkage with GO could provide the proper steric, coordination, and electronic requirements, leading to the bimetallic catalyst demonstrating high catalytic performance. The terpyridine Pd/Ni catalytic monolayer fixed on the graphene oxide nano-sheet was prepared and its catalytic performance and the synergistic effects between different metals were systematically investigated.
2. Experimental
2.1 Reagents and instruments and general procedure
Chemical reagents and instruments used for characterization, general synthesis and the general procedure for the coupling reaction and RactIR recording are provided in the ESI.†
2.2 Preparation and characterization of GO@Tpy-Ni/Pd nano-sheets
The main target here was that the two different metals were arranged in monolayers, which were mutually improved to form higher active species that were different from both individuals. The designed bimetallic catalytic monolayer was fabricated as shown in Scheme 1. Characterization was as follows:
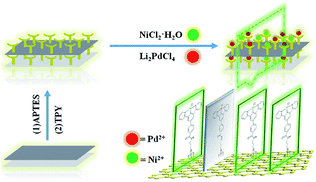 |
| Scheme 1 Preparation route of GO@Tpy-Pd/Ni nano-sheets. | |
FT-IR spectra of GO, GO@APTES, GO@Tpy and GO@Tpy-Pd/Ni were obtained (Fig. S1†). Various peaks for C–O (1053 cm−1), C
O (1726 cm−1), C
C (161 cm−1),
C–H (1381 cm−1) and a broad peak from 3000 to 3500 cm−1 (–OH) revealed the existence of the carboxyl group, hydroxyl group, and epoxy group on GO.70 Compared with GO, the grafted ligand and organometallic compounds could be identified by observing the changes in the functional groups at different steps. After GO was modified with APTES, Si–O–C and Si–O–Si peaks appeared at 1026 cm−1 and 1110 cm−1, providing evidence of silylanization to give GO@APTES.71 The band for the stretching vibration of C
N was observed at 1614 cm−1, suggesting that terpyridine derivatives were linked with GO to yield GO@Tpy. The C
N peak in GO@Tpy was red shifted after coordinating with Ni/Pd solution, attributed to the electron delocalization of C
N due to coordination with metals to form GO@Tpy-Pd/Ni.
The intensity ratio of D/G in RS provides important information.72 The Raman spectra of GO, GO@APTES, GO@Tpy and GO@Tpy-Pd/Ni were measured (Fig. S2†). The ID/IG ratio for GO was about 0.94, while those for GO@APTES, GO@Tpy and GO@Tpy-Pd/Ni were 0.98, 1.03 and 1.02, respectively. This suggested that more numerous but smaller sp2 carbon domains were introduced into GO and the graphene lattice became a little disordered after modification.73
GO, GO@APTES, GO@Tpy and GO@Tpy-Pd/Ni were also characterized by XRD (Fig. S3†). The XRD pattern of GO showed a peak at 10.4° due to the functional groups introduced.74 The XRD patterns of GO@APTES, GO@Tpy and GO@Tpy-Pd/Ni showed a broad peak at 2θ = 21°, confirming that the major oxygen-containing groups of GO were functionalized. Moreover, the diffraction peak at around 10.1° did not vanish, indicating that the structure of GO was not destroyed during the fabrication process.75
SEM and TEM images of GO, GO@APTES, GO@Tpy and GO@Tpy-Pd/Ni were obtained (Fig. S4†). It was apparent that the nano-sheets were layered structures that were closely associated with each other.56 The SEM images showed extending sheets of lateral dimensions ranging from a few to ten micrometers (Fig. S4A†). The SEM image of GO@APTES (Fig. S4B†), GO@Tpy (Fig. S4C†) and GO@Tpy-Pd/Ni (Fig. S4D†) also presented neat sheet-like structures, demonstrating that the ordered Pd/Ni monolayer was modified on GO. Moreover, other sheet-like structures were observed (Fig. S4E†). The TEM images of different steps were also measured (Fig. S4F–H†). The layer-like sheet of GO could also be observed, which was the evidence that the morphology of GO did not change during the modification steps. The catalysts having thin crumpled flakes with wrinkles and many folded regions made it easy for the substrate to access many active sites.
QCM is a useful method in on-line chemical and biological detection through monitoring the change in the mass on the surface of quartz, which also has the advantages of real-time online detection, high sensitivity, and easy operation.76,77 The detection principle is as follows:
ΔF = −2F02(ρqμq) − 1/2Δm/A, −ΔF ∝ Δm. |
QCM was utilized to analyze the preparation process of Au@Tpy-Pd/Ni to simulate the preparation process of GO@Tpy-Pd/Ni. The results are listed in Table 1.
Table 1 Resonance frequency changes in the Au@Tpy-Pd/Ni nanosheet
Entry |
Self-assembly operation |
Frequency (F)/Hz |
ΔF/Hz |
1 |
Au |
9 983 673 |
— |
2 |
Au@OH |
9 983 602 |
↓71 |
3 |
Au@APTES |
9 981 510 |
↓2092 |
4 |
Au@Tpy |
9 977 622 |
↓3888 |
5 |
Au@Tpy-Pd/Ni |
9 974 864 |
↓2758 |
The frequency of the bare quartz wafer was 9
998
673 Hz. During the processes of silanization modification, ligand grafting and metal complexing, the frequency decreased by 71 Hz, 2092 Hz and 3888 Hz, 2758 Hz, respectively. The results illustrated that the quality of the quartz wafer increased during the self-assembly process, which also demonstrated that each self-assembly step on the quartz wafer went on.
The chemical elements in the preparation process of GO@Tpy-Pd/Ni were also measured by XPS analysis (Fig. S5†). The peaks of C 1s and O 1s were detected in GO. Si 2p, Si 2s, and N 1s were clearly detected after anchoring the terpyridine ligand in GO@Tpy. Compared with GO@Tpy, characteristic Pd 3d peaks at 337.92 eV and 343.22 eV denoted the bonding energy (BE) of Pd(II) and the peaks at 855.27 eV, 861.62 eV, 873.42 eV, and 878.54 eV, assigned to the BE of Ni(II), were detected for GO@Tpy-Pd/Ni (Fig. S6†). The characteristics presented above confirmed that the ordered self-assembly of Ni/Pd bimetallic catalytic nano-sheets was fabricated.
3. Results and discussion
3.1 Investigation of the catalytic properties of GO@Tpy-Pd/Ni nanosheets
3.1.1 Evolution of the catalytic performance of GO@Tpy-Pd/Ni. The optimization conditions of Suzuki coupling reactions catalyzed by GO@Tpy-Pd1/Ni1 were initially carried out by screening various solvents, time, bases, temperatures, catalyst loading and substrate usage. The results are summarized in Table S1.† The best yield was obtained by using a H2O/EtOH mixture (v/v = 1
:
3) (entries 1–8). The influence of base was also examined, in which K2CO3 provided the best yield (95%) (entries 7 and 9–11). In addition, 35 °C and 8 h were determined to be the optimum conditions (entries 12–15). With different loadings of catalysts (1, 2 and 3 mg), 1 mg of GO@Tpy-Pd1/Ni1 was the optimized loading (entries 16–17) and the usage of substrate was examined, in which 4-bromotoluene (0.3 mmol) was the best loading (entries 18–19). Based on the results, the best catalytic conditions were K2CO3 as the base, the H2O/EtOH mixture (v/v = 1
:
3) as the solvent, GO@Tpy-Pd1/Ni1 catalyst (1 mg), 4-bromotoluene (0.35 mmol), 35 °C, and 8 h. These were used in further investigations.
3.1.2 The effect of composition on the catalytic properties of GO@Tpy-Pd/Nix. Employing optimized conditions, the effect of composition on the performance of GO@Tpy-Pd/Nix was investigated and the results are listed in Table S2.† Among the three compositions tested, the catalytic yield of GO@Tpy-Ni was only as low as 17%. However, by doping palladium with nickel, the reactivity could be significantly improved, in which GO@Tpy-Pd1/Ni10 provided the best performance. Thus, it was likely that the proper ratio of palladium to nickel on the surface could effectively create a favourable micro-environment to promote catalytic activity. Also, doping with another metal exhibited electronic or steric effects that impacted its catalytic performance by a “cooperative effect”.78 This also implied that proper active sites on the surface could efficiently contact the substrates and catalyze the reaction.
3.1.3 Substrate scoping in the Suzuki coupling reaction catalyzed by GO@Tpy-Pd1/Ni10. Under optimized conditions, Suzuki coupling reactions catalysed by GO@Tpy-Pd1/Ni10 with various aryl halides and arylboronic acids were carried out. The results are depicted in Table S3.† The coupling reactions proceeded with electron-withdrawing and electron-donating aryl bromide and arylboronic acids to give biaryl products with high yields (Table S3,† entries 1–6). However, in the case of aryl chlorides, lower yields were obtained (entries 7 and 8). Coupling product could also be obtained in the case of benzyl bromide with naphthylboronic acid in high yield (entry 9), except with the thiophene derivative (entry 10) due to its poisoning properties toward active sites. These results showed that GO@Tpy-Pd1/Ni10 was an active Ni/Pd bimetallic catalyst for the synthesis of aromatic compounds with higher TON value.
3.1.4 The influence of supports and functional structure on the catalytic performance. Comparison experiments were designed and carried out to investigate the effects of supports on catalytic properties (Table 2).
Table 2 Effects of supports on catalytic propertiesa
Catalyst |
Pd loading (mol g−1) |
Ni loading (mol g−1) |
Molar ratio (Pd/Ni) |
Yieldb/% |
TON |
Reaction conditions: PhB(OH)2 (0.35 mmol), 4-bromotoluene (0.3 mmol), base (0.6 mmol), GO@Tpy-Pd1−x/Nix: 1 mg solvent (5.0 mL) at 35 °C for 8 h. Isolated yield. |
GO |
— |
— |
— |
0 |
0 |
NiCl2/Li2PdCl4 |
1.80 × 10−5 |
3.00 × 10−5 |
1 : 1.70 |
14 |
2333 |
Tpy modified Pd/Ni |
3.92 × 10−5 |
6.89 × 10−5 |
1 : 1.76 |
31 |
2372 |
GO@Tpy-Pd |
7.11 × 10−5 |
— |
— |
91 |
3839 |
GO@Tpy-Ni |
— |
3.28 × 10−5 |
— |
17 |
1555 |
GO@Tpy-Pd1/Ni10 |
1.82 × 10−5 |
2.93 × 10−5 |
1 : 1.60 |
96 |
15 824 |
Gel@Tpy-Pd/Ni |
1.68 × 10−5 |
2.81 × 10−5 |
1 : 1.67 |
59 |
10 535 |
Glass@Tpy-Pd/Ni |
1.74 × 10−5 |
2.75 × 10−5 |
1 : 1.58 |
21 |
3620 |
Coupling compounds were not detected by GO (entry 1). In the case of a simple mixture of Li2PdCl4 and NiCl2, only 14% yield was obtained (entry 2). When a mixture of ligand, Li2PdCl4 and NiCl2 without GO was used, 31% yield was obtained (entry 3). For GO@Tpy-Ni (entry 5), Gel@Tpy-Pd/Ni (entry 7) and Glass@Tpy-Pd/Ni (entry 8), lower yields were obtained, which showed poor activity as compared with GO@Tpy-Pd (entry 4) or GO@Tpy-Pd1/Ni10 (entry 6). GO@Tpy-Pd1/Ni10 also showed higher activity than GO@Tpy-Pd, although the Pd content of GO@Tpy-Pd1/Ni10 was one fourth that of GO@Tpy-Pd. It was evident that the Pd/Ni catalyst could enhance its activity due to the ordered orientation and synergy between nickel and palladium. The TOF value of GO@Tpy-Pd1/Ni10 was 5 times and 3 times greater than that of Glass@Tpy-Pd/Ni and Gel@Tpy-Pd/Ni (entry 7), respectively. This result clearly revealed that GO also had a great role in the activity.79,80 The role of GO containing the functional groups is to disperse and stabilize the bimetallic catalyst, which makes it more favorable for substrates to access the active center because of its two-dimensional configuration and the major interaction of the two metals. This led to efficient electron transfer between the supports, ligand and the catalytic active centre. The catalytic performances of GO@Tpy-Pd1/Ni10 were also compared with the literature (Table S4†).
3.1.5 Stability and recycling experiment. To further investigate the recyclability of GO@Tpy-Pd1/Ni10, recycling tests were performed (Fig. 1). After the sixth run, high activity without a discernible loss could be maintained. However, lower yields were observed in seven cycles (61%). When the time was extended to 12 h in the eighth cycle, the yield increased to 85%. Unfortunately, in the ninth cycle, the yield again dropped to 64%, suggesting that the deactivation of the catalyst occurred in the catalytic process.36–39 In particular, when the freshly prepared GO@Tpy-Pd1/Ni10 was exposed for 1 month under ambient conditions, 90% yield could be obtained for the model reaction, indicating that GO@Tpy-Pd1/Ni10 was extremely stable.
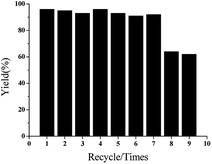 |
| Fig. 1 The recycling experiments of GO@Tpy-Pd1/Ni10 for the Suzuki coupling reaction. | |
To determine the decay mechanism, the changes in the catalyst during the catalytic process were characterized by TEM and SEM (Fig. 2). SEM and TEM after the 1st run showed no obvious changes in the surface morphology (Fig. 2B and E) as compared with the fresh catalyst (Fig. 2A and D). However, there was a clear accumulation on the catalytic surface after six cycles (Fig. 2C and F), which might be unavoidable for most supported metallic catalysts under such reaction conditions. Therefore, we speculated that the decrease in the activity might be due to the aggregation of active species, indicating that it was a structure-sensitive catalytic reaction.81 The Pd and Ni content in GO@Tpy-Pd1/Ni10 recycled for seventh was also measured, in which 1.34 × 10−6 mol g−1 and 1.48 × 10−7 mol g−1 were determined for palladium and nickel, respectively. It indicated that the leaching of metals was also one of the reasons for loss activity.
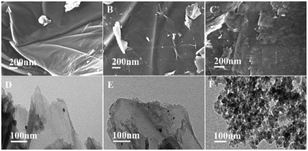 |
| Fig. 2 SEM and TEM images of GO@Tpy-Pd1/Ni10 (A and D) fresh catalyst, (B and E) after the 1st run, (C and F) after the 6th run. | |
3.2 Investigation of the catalytic mechanism
3.2.1 Hot filtration experiment. It is well known that it is important to distinguish heterogeneous catalysts from homogeneous catalysts. The yield increased rapidly before 2 h and then increased slowly after 2 h (Fig. 3, black line). The reaction was finished in 8 h with a 93% yield. The high activity of the GO@Tpy-Pd1/Ni10 was attributed to the favorable configuration and dispersion ability. In order to explore whether Pd leaching occurred during the catalytic process, GO@Tpy-Pd1/Ni10 was removed from the solution in 4 h and the yields were monitored. The yield showed no increase, remaining almost constant (69–71%, Fig. 4, red line), which indicated that no Pd leaching into solution occurred during the catalytic reaction process.35,38
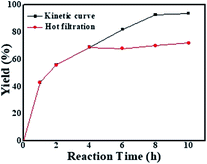 |
| Fig. 3 Kinetic curve and hot filtration experiment for GO@Tpy-Pd1/Ni10. | |
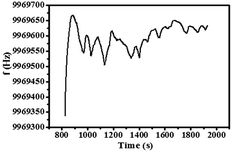 |
| Fig. 4 QCM responses of the sensor in the Suzuki reaction process catalyzed by Au@Tpy-Pd1/Ni10. | |
3.2.2 Poisoning tests. To elucidate the sites on which the catalysis proceeded, poisoning tests were designed and the results are summarized in Table S5.† When a little mercury was added to the catalytic system, only 14% yield was obtained because the limited active centres on the surface of the catalytic nano-sheet could be partially covered by strong poisons in sub-stoichiometric amounts of Hg due to the poor dispersibility of mercury. When thiophene additives were used, even with less than 1.0 equivalent, significant loss of activity was noted due to the strong chemisorption of thiophene on catalytic centres, thus blocking sites for catalytic reaction.82 The results provided evidence that the catalysis mainly proceeded on the surface of GO@Tpy-Pd1/Ni10.
3.2.3 Quartz crystal microbalance (QCM) monitoring. Quartz crystal microbalance (QCM) has the advantages of real-time online detection, high sensitivity, and easy operation.76,77 For further insight into the catalytic mechanism, the Au@Tpy-Pd1/Ni10 self-assembled monolayer was fabricated on the quartz wafer with which the frequency change was detected during the catalysis. As shown in Fig. 4, the frequency of Au@Tpy-Pd1/Ni10 showed the “decrease and increase”. The change in frequency within 700–1300 s was more significant than that during 1300–1700 s. Correspondingly, the quality change on the quartz presented the “increase and decrease” in the catalytic process. This phenomenon indicated that adsorption and desorption occurred. It was clear that the rate of adsorption in the first half was greater than that of desorption, and the desorption rate in the second half was greater than that of adsorption. Finally, the adsorption and desorption reached an equilibrium. The results clearly showed the changes during the catalytic process, which indicated the absorption and desorption processes.
3.2.4 In situ Fourier transform infrared (FTIR) spectroscopy monitoring. The ReactIR 3D maps over time by GO@Tpy-Pd1/Ni10 (Fig. 5A) and Li2PdCl4/NiCl2·6H2O (Fig. 5B) showed a marked difference. For GO@Tpy-Pd1/Ni10, the peak intensity at 754 cm−1 assigned to the coupling product, had no significant change from 0 to 10 min and increased rapidly with time. The final kinetic curve was “S”-shaped until the reaction was completed, which indicated the characteristic heterogeneous catalysis (Fig. 5C, black line).36,83 This could be because the surface of the catalytic monolayer contained a limited number of active centres. The substrates were first adsorbed on the surface without any reaction, which was called an “induced period”. Then, the intermediates were generated by reacting the substrate with specific active sites. Finally, the products were formed and diffused to the reaction solution from the catalytic surface. This was consistent with QCM detection.
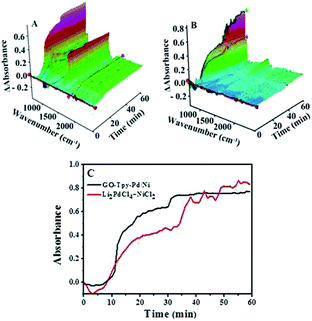 |
| Fig. 5 ReactIR plots over time for the formation of 4-phenyltoluene: (A) 3D map of by GO@Tpy-Pd1/Ni10; (B) 3D map of Li2PdCl4/NiCl2·6H2O; (C) kinetic analysis of the reaction catalyzed by GO@Tpy-Pd1/Ni10 and Li2PdCl4/NiCl2·6H2O using the band at 754 cm−1. Reaction conditions: PhB(OH)2 (1 mmol), 4-bromotoluene (1.5 mmol), base (2 mmol), solvent (6 mL), 35 °C, 1 h. | |
When the same amount of Li2PdCl4/NiCl2·6H2O was used, no coupling product was observed because of the limitation of the amount of catalyst, which indicated that the orientation and distribution of active centres were crucial factors in catalysis. When a greater amount was added, the peak intensity of the product gradually increased within 10–35 minutes and rapidly increased until it reached equilibrium, in which the trend showed a “step” shape (Fig. 5C, red line); however, its intensity was lower than that of GO@Tpy-Pd1/Ni10. This provided evidence that the ordered bimetallic monolayer had a higher catalytic activity under the same conditions.
3.2.5. SEM and TEM images of GO@Tpy-Pd1/Ni10 in the catalytic process. The surface morphology changes in the catalyst during the catalytic process were characterized by SEM and TEM. Compared with the GO@Tpy-Pd1/Ni10, the surface was still a lamellar folded structure after 2 h, 4 h, and 8 h and remained in the basic morphology as shown in Fig. 6. It could be concluded that the designed GO@Tpy-Pd1/Ni10 was structurally stable during the catalytic process.
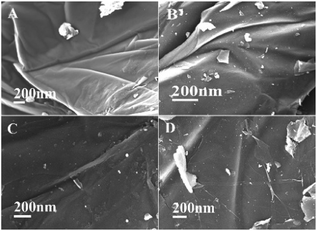 |
| Fig. 6 SEM images of GO@Tpy-Pd1/Ni10 at (A) 0 h, (B) 2 h, (C) 4 h, and (D) 8 h. | |
Correspondingly, TEM images of GO@Tpy-Pd1/Ni10 show a wrinkle-like two-dimensional thin-layer structure (Fig. 7) and nano-sized particles were generated due to the formation of palladium–nickel metal elements after 2 hours and 4 hours. This could be related to the aggregation of the catalytic species to form Ni/Pd clusters due to the migration of active atoms by coupling with each other through complex physicochemical processes during catalysis.82
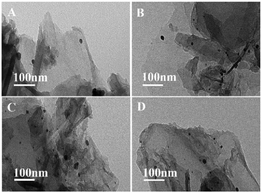 |
| Fig. 7 TEM images of GO@Tpy-Pd1/Ni10 for catalysis at (A) 0 h, (B) 2 h, (C) 4 h, (D) 8 h. | |
3.2.6 XPS investigation of GO@Tpy-Pd1/Ni10 in the catalytic process. The XPS survey spectrum and N 1s, Pd 3d, B 1s and Br 3d XPS spectra of GO@Tpy-Pd1/Ni10 during the catalytic process were obtained (Fig. 8). For the GO@Tpy-Pd1/Ni10, a pair of Pd 3d3/2 and Pd 3d5/2 peaks appeared at 340.74 eV and 335.14 eV, assigned to Pd(0) after 2 h (Fig. 8B), and the intensity of Pd(0) gradually increased with time. In contrast, the intensity of Pd(II) gradually decreased during the catalytic procedure, meaning that a little Pd(II) was reduced to Pd(0), which was the real active centre. The intensity of Pd(0) almost disappeared at the end, and the intensity of the Pd(II) peak gradually recovered, suggesting that Pd(0) might be oxidized to Pd(II). During this process, the N 1s energy level peak had also undergone certain changes (Fig. 8A), in which the N 1s peak appeared at 399.6 eV before catalysis. However, the N 1s peaks shifted to 400.29 eV (1 h), 400.44 eV (4 h) and 399.98 eV (8 h) during the reaction. It was not difficult to find that the position of the N 1s peak in the catalytic process showed a trend of first increasing and then decreasing. This was due to the electron transfer caused by the change in the valence state during the reaction between palladium and nickel that were coordinated with metals. Interestingly, B 1s and Br 3d were detected at 1 h and 4 h, but no B 1s and Br 3d at the end (Fig. 8C and D). Metal elements were generated after starting catalysis and the oxidation of benzyl bromide with metal to give intermediate Br–Pd–Ph inside or on the surface. Afterwards, reduced elimination was achieved by phenylboronic acid with intermediates to yield the coupling product on removing B and Br from the surface.
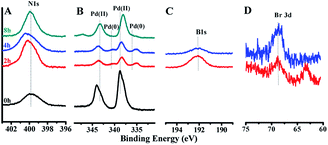 |
| Fig. 8 XPS of (A) N 1s, (B) Pd 3d, (C) B 1s and (D) Br 3d at 0 h, 2 h, 4 h, and 8 h. | |
The energy level change in Ni 2p during the catalytic process was also analyzed (Fig. 9) and the binding energy of the Ni 2p energy peak was reduced after 2 h. The Ni 2p3/2 energy level peak and its satellite peak appeared at 852.84 eV and 863.34 eV after 4 h, which was attributed to Ni(0) being generated due to electron transfer from GO. This could be in the form of several atoms-clusters Ni/Pd during this time, which made it easy for the oxidation step because of much more negative active centres (Scheme 2). The binding energy of the Ni 2p energy level peak gradually returned to the pre-reaction state after 8 h and Ni(0) was oxidized to Ni(II). Regarding the XPS spectra of Ni 2p and Pd 3d, the atomic cluster of Ni(0)/Pd(0) surrounded by appropriate Pd(II)/Ni(II) played a significant role in the catalysis due the synergistic effect, being mainly responsible for its higher activity. It was clear that the clusters were coordinated with ligand mostly at the surface of the organometallic framework. The surface can be regarded as an infinite “organometallic pool” containing a few active species,84 showing the great impact of the modification of a metallic surface by organometallic compounds on its catalytic properties.
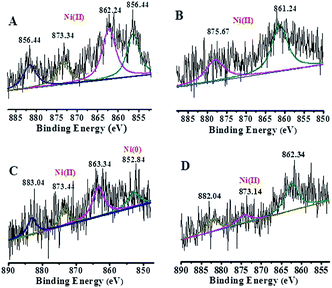 |
| Fig. 9 High-resolution XPS of Ni 2p at different times: (A) 0 h, (B) 2 h, (C) 4 h, and (D) 8 h. | |
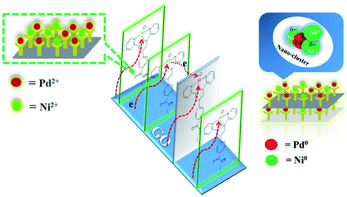 |
| Scheme 2 Proposed formation of the active centre Ni/Pd cluster during the catalytic process. | |
The activity of the catalyst depended on the real active sites on the catalytic surface which were affected by the structure of the ligand and possibilities of electron transfer. In this catalytic monolayer, graphene oxide sheet itself showed some major possibilities of electron transfer and promoted the catalytic activity. The different possibilities of electron transfer in GO@Tpy-Pd1/Ni10 were as follows: (i) Ni(II) to adjacent Pd(II); (ii) Ni(II) passed through adjacent graphene nanolayers to adjacent Pd(II); (iii) Pd(II), Ni(II) to the adjacent carbon atom/graphene nano-sheet. The number of different ways for electron transfer depicted in GO@Tpy-Pd1/Ni10 was considerably higher as compared to the mono-metallic GO@Tpy-Pd and Si@Tpy-Pd1/Ni1 catalyst.
In order to further investigate the synergy between palladium and nickel presented during the catalytic reaction, density functional theory was utilized to calculate the energy barrier of the oxidative insertion process catalyzed by Pd(0) and Ni(0). The Pd–Br, Pd–C and C–Br distances were 3.13, 2.00, and 2.26 Å in transition state TS1, and the energy barrier of the oxidative insertion was 3.6 kcal mol−1 (Fig. S7†). The Ni–Br, Ni–C and C–Br distances were 2.84, 2.54 and 2.12 Å in transition state TS2, and the EB of the oxidative insertion was 17.8 kcal mol−1, which was higher than that of Pd(0) (Fig. S8†). The results showed that both Pd(0) and Ni(0) could form oxidation intermediates, although Pd(0) was much more active than Ni(0) in the oxidation step. The results were consistent with the experiments listed in Table 2.
One of the necessary steps during the catalytic reaction was the adsorption of one or more substrates. This was necessary for investigating the effects of the adsorbed substrates on the active species because it could help to elucidate the mechanism. The overall thinking was that the significant catalysts should integrate with substrates, intermediates or target molecules in certain concentrations to indicate whether the interaction force was too weak to activate the reactants and too strong to release the compounds.85 Thus, it provided a simple way to find the right metal combination in which different metals had various selectivities and functions, although many aspects must be simultaneously taken into account.86–88 DFT was used for investigating the absorption ability of the substrate and desorption ability of the product on different sites on the catalytic surface (Fig. S9†), by which the suitable selection of the bimetallic combination could be predicted.89–92 The results are shown in Table S6,† which were calculated by DFT (Fig. S10†).
Pd(0) had a higher absorption for p-bromotoluene and a weakened absorption for phenyl boronic acid as compared to Pd(II) and Ni(II), indicating that the oxidative insertion could be easily completed by Pd(0) with p-bromotoluene. On the other hand, Pd(II) or Ni(II) in the vicinity of Pd(0) had a higher absorption ability for phenyl boronic acid, which facilitated metallic transfer, with the oxidative insertion intermediate formed on the next-nearest neighbor Pd(0) site. Pd(II), Ni(II) and Pd(0) had similar absorption abilities for coupling compounds. The DFT results obtained above showed that Pd(0) presented the higher activity because of its higher activation toward p-bromotoluene, and weaker absorption for the products. Meanwhile, Pd(II) and Ni(II) showed their stronger absorption for phenyl boronic acid, which was helpful for metallic transfer, and lower absorption for the coupling compound, which diffused easily from the surface. It was evident that the bimetallic catalyst had higher activity than the single metallic catalyst due to their synergy in position, orientation or distribution-selective processes, indicating that both active-sites and non-active sites participated simultaneously in the catalytic process as shown in Scheme 3. The presumed processes confirmed by DFT were consistent with the XPS variation during the catalytic process as shown in Fig. 9. We propose that new adsorption sites were likely formed with different electron densities, strengthening p-bromotoluene adsorption for Pd/Ni, which made Pd(0) compete more efficiently for adsorption sites in the form of the co-adsorption of phenyl boron acid and p-bromotoluene adsorption; therefore, nickel was considered as a promoter.93 This also suggests that Pd(0)/Ni(0) and Pd(II)/Ni(II) are not mutually exclusive, but have synergistic interactions as whole, indicating that one can consider that active clusters adopt a coordination sphere already present in the organometallic catalyst surface.19 This could be called the self-assembly organometallic framework nano-sheet (OMFNS), in which the morphology of the organometallic surface, orientation of the organometallic framework in the monolayer, kinds of ligands, support selected, ratio of bimetal, and interaction between aryl groups were crucial to the activity and stability of the catalyst.
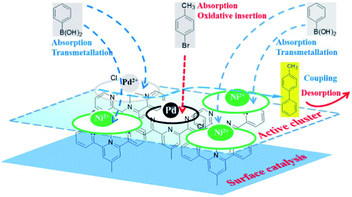 |
| Scheme 3 Synergy among the active sites during the catalytic process in the self-assembly of the organometallic framework films (OMFFs). | |
4. Conclusion
A new graphene oxide-supported Ni/Pd terpyridine self-assembled catalytic monolayer (denoted as GO@Tpy-Ni/Pd) was fabricated, which exhibited higher catalytic activity, substrate applicability and recyclability as a heterogeneous catalyst for the Suzuki coupling reaction under mild conditions. QCM was used for characterizing and monitoring the reaction by the on-line detection of the frequency changes in a quartz wafer linked to the bimetallic catalyst, and clearly different rates of adsorption and desorption were presented during the catalytic process. The synergetic mechanism between nickel and palladium was investigated in detail, in which the atomic-scale cluster of Ni/Pd could be formed. The real active centre was the crucial factor that made it easy for the initial oxidation step to occur. The absorption and adsorption on the Ni/Pd clusters in the bimetallic Pd–Ni core–shell nanoparticles as effective catalysts for the Suzuki reaction surface for different substrates and products, as calculated by DFT, was also an important factor. This indicated that the synergy between the active site and the doped second metal made it easy for the initial oxidation step and trans-metallation to occur, by which the proper design of the catalyst, including the ligand, selection of the support, the suitable combination of metals and elucidation of the catalytic mechanism could be achieved.
Conflicts of interest
There are no conflicts to declare.
Acknowledgements
This work was supported by the NSFC (21861132002), the Henan Natural Science Foundation of China (192102210046) for their financial support. The authors thank Prof. Zhi Ma (Institute of Chemistry, Chinese Academy of Sciences, Shanghai) for Raman measurement.
Notes and references
- B. H. Lipshutz, N. A. Isley, J. C. Fennewald and E. D. Slack, On the Way Towards Greener Transition-Metal-Catalyzed Processes as Quantified by E Factors, Angew. Chem., Int. Ed., 2013, 52, 10952–10958 CrossRef CAS PubMed.
- N. A. Isley, F. Gallou and B. H. Lipshutz, Transforming Suzuki−Miyaura Cross-Couplings of MIDA Boronates into a Green Technology: No Organic Solvents, J. Am. Chem. Soc., 2013, 135, 17707–17710 CrossRef CAS PubMed.
- T. Iwasaki and N. Kambe, Ni-Catalyzed C–C Couplings Using Alkyl Electrophiles, Top. Curr. Chem., 2016, 374(5), 66 CrossRef PubMed.
- P. X. Xi, Y. Cao, F. C. Yang, C. Ma, F. J. Chen, S. Yu, S. Wang, Z. Z. Zeng and X. Zhang, Facile synthesis of Pd-based bimetallic nanocrystals and their application as catalysts for methanol oxidation reaction, Nanoscale, 2013, 5, 6124–6130 RSC.
- F. Saleem, Z. C. Zhang, B. Xu, X. B. Xu, P. L. He and X. Wang, Ultrathin Pt–Cu nanosheets and nanocones, J. Am. Chem. Soc., 2013, 135, 18304–18307 CrossRef CAS PubMed.
- Z. Wang, B. Li, X. Y. Zhang and X. S. Fan, One-Pot Cascade Reactions Leading to Pyrido[2′,1′:2,3]imidazo[4,5-c][1,2,3]triazolo[1,5-a]quinolines under Bimetallic Relay Catalysis with Air as the Oxidant, J. Org. Chem., 2016, 81, 6357–6363 CrossRef CAS PubMed.
- J. Xiang, P. Li, H. B. Chong, L. Feng, F. Y. Fu, Z. Wang, S. L. Zhang and M. Z. Zhu, Bimetallic Pd–Ni core–shell nanoparticles as effective catalysts for the Suzuki reaction, Nano Res., 2014, 7(9), 1337–1343 CrossRef CAS.
- R. K. Rai, K. Gupta, S. Behrens, J. Li, Q. Xu and S. K. Singh, Highly Active Bimetallic Nickel–Palladium Alloy Nanoparticle Catalyzed Suzuki–Miyaura Reactions, ChemCatChem, 2015, 7, 1806–1812 CrossRef CAS.
- S. F. Cai, H. H. Duan, H. P. Rong, D. S. Wang, L. S. Li, W. He and Y. D. Li, Highly Active and Selective Catalysis of Bimetallic Rh3Ni1 Nanoparticles in the Hydrogenation of Nitroarenes, ACS Catal., 2013, 3, 608–612 CrossRef CAS.
- M. Ahmadi, F. Behafaid, C. H. Cui, P. Strasser and B. R. Cuenya, Long-range segregation phenomena in shape-Selected bimetallic nanoparticles: Chemical state effects, ACS Nano, 2013, 7, 9195–9204 CrossRef CAS PubMed.
- S. U. Son, Y. J. Jang, J. Park, H. B. Na, H. M. Park, H. J. Yun, J. Lee and T. Hyeon, Designed Synthesis of Atom-Economical Pd/Ni Bimetallic Nanoparticle-Based Catalysts for Sonogashira Coupling Reactions, J. Am. Chem. Soc., 2004, 126, 5026–5027 CrossRef CAS PubMed.
- P. Serna, P. Concepcioń and A. Corma, Design of highly active and chemoselective bimetallic gold–platinum hydrogenation catalysts through kinetic and isotopic studies, J. Catal., 2009, 265, 19–25 CrossRef CAS.
- M. Masjedi, L. T. Yildirim and S. Özkar, Novel homogeneous catalyst comprising ruthenium and trimethylphosphite for the hydrolysis of sodium borohydride, J. Mol. Catal. A: Chem., 2012, 355, 186–191 CrossRef CAS.
- M. S. Kutubi, K. Sato, K. Wada, T. Yamamoto, S. Matsumura, K. Kusada, H. Kobayashi, H. Kitagawa and K. Nagaoka, Dual Lewis Acidic/Basic Pd0.5Ru0.5-Poly(N-vinyl-2-pyrrolidone) Alloyed Nanoparticle: Outstanding Catalytic Activity and Selectivity in Suzuki-Miyaura Cross-Coupling Reaction, ChemCatChem, 2015, 7, 3887–3894 CrossRef CAS.
- T. E. Barder, S. D. Walker, J. R. Martinelli and S. L. Buchwald, Catalysts for Suzuki-Miyaura Coupling Processes: Scope and Studies of the Effect of Ligand Structure, J. Am. Chem. Soc., 2005, 127, 4685–4696 CrossRef CAS PubMed.
- X. F. Yang, A. Q. Wang, B. T. Qiao, J. Li, J. Y. Liu and T. Zang, Single-Atom Catalysts: A New Frontier in Heterogeneous Catalysis, Acc. Chem. Res., 2013, 46, 1740–1748 CrossRef CAS PubMed.
- R. J. Key, J. M. M. Tengco, M. D. Smith and A. K. Vannucci, A Molecular/Heterogeneous Nickel Catalyst for Suzuki-Miyaura Coupling, Organometallics, 2019, 38, 2007–2014 CrossRef CAS.
- K. Fuku, T. Sakano, T. Kamegawa, K. Mori and H. Yamashita, Enhanced hydrogenation activity of nano-sized Pd–Ni bimetal particles on Ti-containing mesoporous silica prepared by a photo-assisted deposition method, J. Mater. Chem., 2012, 22, 16243–16247 RSC.
- S. K. Manoja, V. D'Elia, E. Pump, L. Falivene, M. Harb, S. O. Chikh, L. Cavallo and J. M. e. Basset, The Comparison between Single Atom Catalysis and Surface Organometallic Catalysis, Chem. Rev., 2020, 120, 734–813 CrossRef PubMed.
- H. Fei, J. Dong, M. J. Arellano-Jimenez, G. Ye, N. D. Kim, E. L. G. Samuel, Z. Peng, Z. Zhu, F. Qin, J. Bao, M. J. Yacaman, P. M. Ajayan, D. Chen and J. M. Tour, Atomic cobalt on nitrogen-doped graphene for hydrogen generation, Nat. Commun., 2015, 6, 8668–8671 CrossRef CAS PubMed.
- H. L. Su, S. J. Wu, Z. F. Li, Q. S. Huo, J. Q. Guan and Q. B. Kan, Co(II), Fe(III) or VO(II) Schiff base metal complexes immobilized on graphene oxide for styrene epoxidation, Appl. Organomet. Chem., 2015, 29, 462–467 CrossRef CAS.
- T. S. Marshall, M. O'Brien, B. Oetter, A. Corpuz, R. M. Richards, D. K. Schwartz and J. W. Medlin, Controlled selectivity for palladium catalysts using self-assembled monolayers, Nat. Mater., 2010, 9, 853–858 CrossRef PubMed.
- X. S. Zhao, F. B. Su, Q. F. Yang, W. P. Guo, X. P. Bao, L. Z. Lv and C. Zhou, Templating methods for preparation of porous structures, J. Mater. Chem., 2006, 16, 637–648 RSC.
- K. Hara, S. Tayama, H. Kano, T. Masuda, S. Takakusagi, T. Kondo, K. Uosaki and M. Sawamura, Functionalization of silicon surfaces with catalytically active Pd complexes and application to the aerobic oxidation of benzylic alcohols, Chem. Commun., 2007, 4280–4282 RSC.
- M. L. Xiao, L. G. Feng, J. B. Zhu, C. P. Liu and W. Xing, Rapid synthesis of a PtRu nano-sponge with different surface compositions and performance evaluation for methanol electrooxidation, Nanoscale, 2015, 7, 9467–9471 RSC.
- J. Wang, B. Mu, Z. H. Fu, L. Wang, T. S. Li and Y. J. Wu, Cyclopalladated ferrocenylimines with ester groups for Heck and Suzuki coupling reactions, Chin. J. Catal., 2014, 35(7), 1059–1067 CrossRef CAS.
- B. Mu, T. S. Li, J. Y. Li and Y. J. Wu, The highly efficient Suzuki–Miyaura cross-coupling reaction using cyclopalladated N-alkylferrocenylimine as a catalyst in aqueous medium at room temperature under ambient atmosphere, J. Organomet. Chem., 2008, 693(7), 1243–1251 CrossRef CAS.
- B. Mu, T. S. Li, Z. H. Fu and Y. J. Wu, Cyclopalladated ferrocenylimines catalyzed-homocoupling reaction of arylboronic acids in aqueous solvents at room temperature under ambient atmosphere, Catal. Commun., 2009, 10, 1497–1501 CrossRef CAS.
- Z. H. Fu, N. Zhang, T. S. Li, W. J. Xu and Y. J. Wu, N-hydroxymethyl acrylamide polymer brush and its application in catalyzing coupling reaction, J. Colloid Interface Sci., 2013, 394, 409–418 CrossRef CAS PubMed.
- N. Zhao, T. S. Li, Z. Zhai, J. J. Qiu, W. J. Xu, M. H. Liu and Y. J. Wu, Cyclopalladated Arylimine Self-Assembly Films for Suzuki Reaction, ChemCatChem, 2013, 5(6), 1481–1489 CrossRef CAS.
- Z. H. Fu, T. S. Li, X. H. He, J. Liu, W. J. Xu and Y. J. Wu, Cyclopalladated ferrocenylimine functionalized polymer brushes film and its mechanism investigation of heterogeneous catalysis, J. Mol. Catal. A: Chem., 2014, 395, 293–299 CrossRef CAS.
- H. Liu, T. S. Li, X. X. Xue, W. J. Xu and Y. J. Wu, The mechanism of a self-assembled Pd (ferrocenylimine)–Si compound-catalysed Suzuki coupling reaction, Catal. Sci. Technol., 2016, 6, 1667–1676 RSC.
- Z. Q. Xue, P. P. Huang, T. S. Li, P. X. Qin, D. Xiao, M. H. Liu, P. L. Chen and Y. J. Wu, A novel “tunnel-like” cyclopalladated arylimine catalyst immobilized on grapheme oxide nano-sheet, Nanoscale, 2017, 9, 781–791 RSC.
- X. Gu, T. S. Li, P. L. Chen, M. H. Liu and Y. J. Wu, A Facile Fabrication of Ordered Component-tunable Hetero-bimetallic Self-assembly Nano-sheet for Catalyzing Click Reaction, ACS Omega, 2107, 2, 5415–5433 CrossRef PubMed.
- L. H. Wang, P. P. Huang, J. Yang, T. S. Li, L. Y. Mao, M. H. Liu and Y. J. Wu, Fabrication and Catalytic Properties of Ordered Cyclopalladated Diimine Monolayer—Investigation on Catalyzing Mechanism, RSC Adv., 2018, 8, 31860–31867 RSC.
- P. P. Huang, Z. Q. Xue, T. S. Li, Z. Y. Liu, D. H. Wei, M. H. Liu and Y. J. Wu, Investigation on Electron Distribution and Synergetic to Enhance Catalytic Activity in Bimetallic Ni(II)/Pd(II) Molecular Monolayer, ChemCatChem, 2018, 10, 5141–5155 CrossRef CAS.
- P. P. Huang, E. R. Song, Y. M. Sun, T. S. Li, M. H. Liu and Y. J. Wu, Schiff-based Pd(II)/Fe(III) Bimetallic Self-assembly Monolayer—Preparation, Structure, Catalytic Dynamic and Synergistic, Mol. Catal., 2019, 469, 75–86 CrossRef CAS.
- W. L. Shang, X. F. Zeng, T. S. Li, W. J. Xu, D. H. Wei, M. H. Liu and Y. J. Wu, Controlled Distribution of Active Centre to Enhance Catalytic Activity of Ordered Pd/Co Catalytic Nano-monolayer, J. Catal., 2019, 376, 228–237 CrossRef CAS.
- E. R. Song, J. Wang, T. S. Li, W. D. Zhao, M. H. Liu and Y. J. Wu, Novel Ordered Cyclopalladated Aryl Imine Monolayers—Structure Designing for Enhancing Catalytic Performance, Mol. Catal., 2020, 482, 110671 CrossRef.
- A. A. Ensafi, M. Jafari-Asl and B. Rezaei, Graphene nanosheets functionalized with 4-aminothiophenol as a stable support for the oxidation of formic acid based on self-supported Pd-nanoclusters via galvanic replacement from Cu2O nanocubes, J. Electroanal. Chem., 2014, 731, 20 CrossRef CAS.
- W. Yuan and G. Shi, Graphene-based gas sensors, J. Mater. Chem. A, 2013, 1, 10078–10091 RSC.
- J. Li, Q.-L. Zhu and Q. Xu, Non-noble bimetallic CuCo nanoparticles encapsulated in the pores of metal–organic frameworks: synergetic catalysis in the hydrolysis of ammonia borane for hydrogen generation, Catal. Sci. Technol., 2015, 5, 525–530 RSC.
- H. L. Jiang, T. Akita, T. Ishida, M. Haruta and Q. Xu, Synergistic Catalysis of Au@Ag Core-Shell Nanoparticles Stabilized on Metal-Organic Framework, J. Am. Chem. Soc., 2011, 133, 1304–1306 CrossRef CAS PubMed.
- S. Maheswari, S. Karthikeyan, P. Murugan, P. Sridhar and S. Pitchumani, Carbon−Supported Pd−Co as Cathode Catalyst for Apemfes and Validation by DFT, Phys. Chem. Chem. Phys., 2012, 14, 9683–9695 RSC.
- S. Z. Tasker, E. A. Standley and T. F. Jamison, Recent advances in homogeneous nickel catalysis, Nature, 2014, 509(7500), 299–309 CrossRef CAS PubMed.
- J. Yamaguchi, K. Muto and K. Itami, Recent Progress in Nickel-Catalyzed Biaryl Coupling, Eur. J. Org. Chem., 2013, 19–30 CrossRef CAS.
- B. M. Rosen, K. W. Quasdorf, D. A. Wilson, N. Zhang, A. M. Resmerita, N. K. Garg and V. Percec, Nickel-Catalyzed Cross-Couplings Involving Carbon–Oxygen Bonds, Chem. Rev., 2011, 111, 1346–1416 CrossRef CAS PubMed.
- S. Singh and R. B. Sunoj, Mechanism and Origin of Enantioselectivity in Nickel-Catalyzed Alkyl−Alkyl Suzuki Coupling Reaction, J. Phys. Chem. A, 2019, 123, 6701–6710 CrossRef CAS PubMed.
- F. S. Han, Transition-metal-catalyzed Suzuki–Miyaura cross-coupling reactions: a remarkable advance from palladium to nickel catalysts, Chem. Soc. Rev., 2013, 42, 5270–5298 RSC.
- R. Q. Li, H. Xu, N. Zhao, X. J. Jin and Y. F. Dang, Origins of Chemoselectivity in the Ni-Catalyzed Biaryl and PdCatalyzed Acyl Suzuki−Miyaura Cross-Coupling of N-Acetyl-Amides, J. Org. Chem., 2020, 85, 833–840 CrossRef CAS PubMed.
- C. S. Zhang, R. H. Zhao, W. M. Dagnaw, Z. Y. Liu, Y. Lu and Z. X. Wang, Density Functional Theory Mechanistic Insight into the Base-Free Nickel-Catalyzed Suzuki−Miyaura Cross-Coupling of Acid Fluoride: Concerted versus Stepwise Transmetalation, J. Org. Chem., 2019, 84, 13983–13991 CrossRef CAS PubMed.
- P. A. Payard, L. A. Perego, I. Ciofini and L. Grimaud, Taming Nickel-Catalyzed Suzuki-Miyaura Coupling: A Mechanistic Focus on Boron-to-Nickel Transmetalation, ACS Catal., 2018, 8, 4812–4823 CrossRef CAS.
- Z. C. Cao, S. J. Xie, H. Y. Fang and Z. J. Shi, Ni-Catalyzed Cross-Coupling of Dimethyl Aryl Amines with Arylboronic Esters under Reductive Conditions, J. Am. Chem. Soc., 2018, 140, 13575–13579 CrossRef CAS PubMed.
- S. Akkarasamiyo, J. Margalef and J. S. M. Samec, Nickel-Catalyzed Suzuki-Miyaura Cross-Coupling Reaction of Naphthyl and Quinolyl Alcohols with Boronic Acids, Org. Lett., 2019, 21, 4782–4787 CrossRef CAS PubMed.
- R. J. Key, J. M. M. Tengco, M. D. Smith and A. K. Vannucci, A Molecular/Heterogeneous Nickel Catalyst for Suzuki–Miyaura Coupling, Organometallics, 2019, 38(9), 2007–2014 CrossRef CAS.
- S. B. Tailor, M. Manzotti, S. Asghar, B. J. S. Rowsell, S. L. J. Luckham, H. A. Sparkes and R. B. Bedford, Revisiting Claims of the Iron-, Cobalt-, Nickel-, and Copper-Catalyzed Suzuki Biaryl Cross-Coupling of Aryl Halides with Aryl Boronic Acids, Organometallics, 2019, 38(8), 1770–1777 CrossRef CAS.
- A. M. Oertel, V. Ritleng and M. J. Chetcuti, Synthesis and Catalytic Activity in Suzuki Coupling of Nickel Complexes Bearing n-Butyl- and Triethoxysilylpropyl-Substituted NHC Ligands: Toward the Heterogenization of Molecular Catalysts, Organometallics, 2012, 31, 2829–2840 CrossRef CAS.
- A. K. Cooper, D. K. Leonard, S. Bajo, P. M. Burton and D. J. Nelson, Aldehydes and ketones influence reactivity and selectivity in nickel-catalysed Suzuki–Miyaura reactions, Chem. Sci., 2020, 11, 1905–1911 RSC.
- X. Y. Lu, L. Y. Yan, J. S. Li, J. M. Li, H. P. Zhou, R. C. Jiang, C. C. Liu, R. Lu and R. Hu, Base-free Ni-catalyzed Suzuki-type cross-coupling reactions of epoxides with boronic acids, Chem. Commun., 2020, 56, 109–112 RSC.
- Y. Dong, J. J. Jv, Y. Li, W. H. Li, Y. Q. Chen, Q. Sun, J. P. Ma and Y. B. Dong, Nickel-metalated porous organic polymer for Suzuki–Miyaura cross-coupling reaction, RSC Adv., 2019, 9, 20266–20272 RSC.
- S. D. Ramgren, L. Hie, Y. Ye and N. K. Garg, Nickel-Catalyzed Suzuki-Miyaura Couplings in Green Solvents, Org. Lett., 2013, 15, 3950–3953 CrossRef CAS PubMed.
- J. Xia, Y. Fu, G. He, X. Q. Sun and X. Wang, Core-shell-like Ni-Pd nanoparticles supported on carbon black as a magnetically separable catalyst for green Suzuki-Miyaura coupling reactions, Appl. Catal., B, 2017, 200, 39–46 CrossRef CAS.
- S. Jang, T. Kim and K. H. Park, Fabrication of Crumpled Ball-Like Nickel Doped Palladium-Iron Oxide Hybrid Nanoparticles with Controlled Morphology as Effective Catalyst for Suzuki–Miyaura Coupling Reaction, Catalysts, 2017, 7(9), 247 CrossRef.
- A. Ohtaka, J. M. Sansano and C. Nájera, et al., Palladium and Bimetallic Palladium–Nickel Nanoparticles Supported on Multiwall Carbon Nanotubes: Application to Carbon- Carbon Bond-Forming Reactions in Water, ChemCatChem, 2015, 7(12), 1841–1847 CrossRef CAS.
- M. Sera, M. Yamashita, Y. Ono, T. Tabata, E. Muto, T. Ouchi and H. Tawada, Development of Large-Scale Synthesis using a Palladium-Catalyzed Cross-Coupling Reaction for an Isoquinolone Derivative as a Potent DPP-4 Inhibitor, Org. Process Res. Dev., 2014, 18, 446–453 CrossRef CAS.
- Y. E. Wu, D. S. Wang, P. Zhao, Z. Q. Niu, Q. Peng and Y. D. Li, Monodispersed Pd–Ni nanoparticles: composition control synthesis and catalytic properties in the Miyaura–Suzuki reaction, Inorg. Chem., 2011, 50, 2046–2048 CrossRef CAS PubMed.
- G. Y. Bao, J. Bai and C. P. Li, Synergistic effect of the Pd–Ni bimetal/carbon nanofiber composite catalyst in Suzuki coupling reaction, Org. Chem. Front., 2019, 6, 352–361 RSC.
- E. D. Sultanova, A. I. Samigullina, N. V. Nastapova, I. R. Nizameev, K. V. Kholin, V. I. Morozov, A. T. Gubaidullin, V. V. Yanilkin, M. K. Kadirov, A. Y. Ziganshina and A. I. Konovalov, Highly active Pd–Ni nanocatalysts supported on multicharged polymer matrix, Catal. Sci. Technol., 2017, 7, 5914–5919 RSC.
- R. K. Rai, K. Gupta, D. Tyagi, A. Mahata, S. Behrens, X. Yang, Q. Xu, B. Pathakad and S. K. Singh, Access to highly active Ni–Pd bimetallic nanoparticle catalysts for C–C coupling reactions, Catal. Sci. Technol., 2016, 6(14), 5567–5579 RSC.
- H. Su, Z. Li, Q. Huo, J. Guan and Q. Kan, Immobilization of transition metal (Fe2+, Co2+, VO2+ or Cu2+) Schiff base complexes onto graphene oxide as efficient and recyclable catalysts for epoxidation of styrene, RSC Adv., 2014, 4, 9990–9997 RSC.
- H. P. Mungse, S. Verma, N. Kumar, B. Sain and O. P. Khatri, Grafting of oxo-vanadium Schiff base on graphene nanosheets and its catalytic activity for the oxidation of alcohols, J. Mater. Chem., 2012, 22, 5427–5433 RSC.
- Z.-G. Le, Z. Liu, Y. Qian and C. Wang, A facile and efficient approach to decoration of graphene nanosheets with gold nanoparticles Appl, Surf. Sci., 2012, 258, 5348–5353 CrossRef CAS.
- Z. Li, S. Wu, H. Ding, D. Zheng, J. Hu, X. Wang, Q. Huo, J. Guan and Q. Kan, Immobilized Cu(ii) and Co(ii) salen complexes on graphene oxide and their catalytic activity for aerobic epoxidation of styrene, New J. Chem., 2013, 37, 1561–1568 RSC.
- S. Choudhary, H. P. Mungse and O. P. Khatri, Dispersion of alkylated graphene in organic solvents and its potential for lubrication applications, J. Mater. Chem., 2012, 22, 21032 RSC.
- Y. Zu, J. Tang, W. Zhu, M. Zhang, G. Liu, Y. Liu, W. Zhang and M. Jia, Graphite oxide-supported CaO catalysts for transesterification of soybean oil with methanol, Bioresour. Technol., 2011, 102, 8939–8944 CrossRef CAS PubMed.
- T. Nakamoto and T. Moriizumi, A Theory of a Quartz Crystal Microbalance Based upon a Mason Equivalent Circuit, Jpn. J. Appl. Phys., 1990, 29, 963–969 CrossRef.
- K. Takada, G. D. Storrier, F. Pariente and H. D. Abrunã, EQCM Studies of the Redox Processes during and after Electropolymerization of Films of Transition-Metal Complexes of Vinylterpyridine, J. Phys. Chem. B, 1998, 102, 1387–1396 CrossRef CAS.
- W. A. Herrmann and B. Cornils, Organometallic Homogeneous Catalysis-Quo
vadis?, Angew. Chem., Int. Ed., 1997, 36, 1048–1067 CrossRef.
- Ö. Metin, S. F. Ho, C. Alp, H. Can, M. N. Mankin, M. S. Gültekin, M. Chi and S. Sun, Ni/Pd Core/Shell Nanoparticles Supported on Graphene as a Highly Active and Reusable Catalyst for Suzuki-Miyaura Cross-Coupling Reaction, Nano Res., 2013, 1, 10–18 CrossRef.
- H. Göksu, H. Can, K. Sendil, M. S. Gültekin and Ö. Metin, Reduced Graphene Oxide Supported CoPd Nanoalloys Catalyzed Tandem Ammonia Borane Dehydrogenationand Hydrogenation of Aromatic Nitro, Nitrile and Carbonyl Compounds, Appl. Catal., A, 2014, 488, 176–182 CrossRef.
- B. C. Gates, Supported Metal Clusters: Synthesis, Structure, and Catalysis, Chem. Rev., 1995, 95, 511–522 CrossRef CAS.
- C. H. Bartholomew, Mechanisms of catalyst deactivation, Appl. Catal., A, 2001, 212, 17–60 CrossRef CAS.
- S. Özkar, R. G. Finke, S. Özkar and R. G. Finke, Nanocluster Formation and Stabilization Fundamental Studies: Ranking Commonly Employed Anionic Stabilizers via the Development, Then Application, of Five Comparative Criteria, J. Am. Chem. Soc., 2002, 124, 5796–5810 CrossRef PubMed.
- M. L. H. Green, A New Approach to the Formal Classification of Covalent Compounds of the Elements, J. Organomet. Chem., 1995, 500, 127–148 CrossRef CAS.
- A. J. Medford, A. Vojvodic, J. S. Hummelshøj, J. Voss, F. A. Pedersen, F. Studt, T. Bligaard, A. Nilsson and J. K. Nørskov, From the Sabatier principle to a predictive theory of transition-metal heterogeneous catalysis, J. Catal., 2015, 328, 36–42 CrossRef CAS.
- V. Ponec, Alloy catalysts: the concepts, Appl. Catal., A, 2001, 222, 31–45 CrossRef CAS.
- A. Christensen, A. V. Ruban, P. Stoltze, K. W. Jacobsen, H. L. Shriver and J. Nørskov, Phase diagrams for surface alloys, Phys. Rev. B: Condens. Matter Mater. Phys., 1997, 56, 5822–5834 CrossRef CAS.
- P. V. Helden, F. Prinsloo, J.-A. van den Berg, B. Xaba, W. Erasmus, M. Claeys and Jan van de Loosdrecht, Cobalt-nickel bimetallic Fischer-Tropsch catalysts: a combined theoretical and experimental approach, Catal. Today, 2020, 342, 88–98 CrossRef.
- X. P. Zhang, J. X. Wang, B. J. Tan, N. Zhang, J. J. Bao and G. H. He, Ce-Co interaction effects on the catalytic performance of uniform mesoporous Cex-Coy catalysts in Hg0 oxidation process, Fuel, 2018, 226, 18–26 CrossRef CAS.
- D. H. Wei, X. Q. Huang, Y. Qiao, J. L. Wang, L. Fei and C. G. Zhan, Catalytic Mechanisms for Cofactor-Free Oxidase-Catalyzed Reactions: Reaction Pathways of Uricase-Catalyzed Oxidation and Hydration of Uric Acid, ACS Catal., 2017, 4623–4636 CrossRef CAS PubMed.
- Y. Wang, D. H. Wei and M. S. Tang, Computational Study on γ-C–H Functionalization of α,β-Unsaturated Ester Catalyzed by N-Heterocyclic Carbene: Mechanisms, Origin of Stereoselectivity, and Role of Catalyst, J. Org. Chem., 2017, 82, 13043–13050 CrossRef CAS PubMed.
- N. Liu, Y. F. Xie, C. Wang, S. J. Li, D. H. Wei and B. Dai, Cooperative Multifunctional Organocatalysts for Ambient Conversion of Carbon Dioxide into Cyclic Carbonates, ACS Catal., 2018, 8, 9945–9957 CrossRef CAS.
- G. Voss, J. Fløystad, A. Voronov and M. Rønning, The State of Nickel as Promotor in Cobalt Fischer–Tropsch Synthesis Catalysts, Top. Catal., 2015, 58, 896–904 CrossRef CAS.
Footnotes |
† Electronic supplementary information (ESI) available. See DOI: 10.1039/d0ra02195d |
‡ Contributed equally to this work. |
|
This journal is © The Royal Society of Chemistry 2020 |
Click here to see how this site uses Cookies. View our privacy policy here.