DOI:
10.1039/D0RA04204H
(Paper)
RSC Adv., 2020,
10, 28300-28313
The interaction between oxytocin and heparin
Received
11th May 2020
, Accepted 20th July 2020
First published on 29th July 2020
Abstract
Oxytocin (OXT) is a small cyclic peptide that is administered to pregnant women to induce birth in cases where labour is prolonged. It has previously been observed that patients taking a low molecular weight heparin, dalteparin (DAL), and then prescribed, OXT experienced a swifter labour compared to women given OXT alone. Herein are described the interactions between OXT and a number of heparin-based oligosaccharides; DAL; fondaparinux (FP), which is a synthetic heparin oligosaccharide that represents the predominant antithrombin binding-site, and a family of chemically-derived heparin hexasaccharides. The latter oligosaccharides were chosen as they represent sequences found within the polysaccharide dalteparin. Furthermore, the carbohydrate chemical space was investigated by comparing the interaction between OXT and four chemically derivatived heparin hexasaccharides; I2S-A6SNS (DP6), I2OH-A6SNS (DP6-2OH, de-2-O-sulfated hexasaccharide), I2S-A6OHNS (DP6-6OH, de-6-O-sulfated hexasaccharide) and I2S-A6SNAc (DP6-NAc, de-N-sulfated hexasaccharide). The interactions between the peptide and oligosaccharides were studied using a series of 13C–1H and 15N–1H HSQC NMR experiments, at a range of temperatures. This approach allowed the binding epitopes of the peptide and oligosaccharides to be identified, highlighting that 6-O- and N-sulfation substituent groups of heparin are important for the interaction between the peptide and carbohydrate. This is an important observation as de-N-sulfation is a traditional method for decreasing the anticoagulation properties of heparin. Furthermore, low temperature experiments of the OXT
:
FP complex indicate that hydrogen-bonding is very important for the interaction between the peptide and oligosaccharide.
1. Introduction
Heparin has been used as an anticoagulant in the clinic for over 80 years,1 the anticoagulant activity is driven through interactions with members of the clotting cascade, most notably thrombin and antithrombin.2 Heparin is a biological medicine, being derived from living organisms, the majority of which is extracted from the intestinal mucosa of pigs.3
Heparin is a linear polyanionic carbohydrate, composed of the repeating disaccharide unit of an uronic acid bound to glucosamine through (1 → 4) glycosidic linkages. Heparin is heterogenous due to the carbohydrate's variable chain lengths and different substitutions of the disaccharide subunits. The uronic acid residue is primarily α-L-iduronic acid but is also present as its C-5 epimer β-D-glucuronic acid. Also, the uronic acid can be O-sulfated at position-2. The N-glucosamine can be decorated by sulfates at several positions. At position-2 (the amino function) the glucosamine can carry either an acetyl, sulfate or amine group. Furthermore, the glucosamine can be O-sulfated at position-6 and more rarely at position-3 (Fig. 1A).4 The primary, but not exclusive, binding site of antithrombin in heparin is a pentasaccharide containing an α-D-glucosamine that is trisulfated; O-sulfated at positions-3 and -6 and N-sulfated. The sequence of the pentasaccharide can be found in Fig. 1C.5
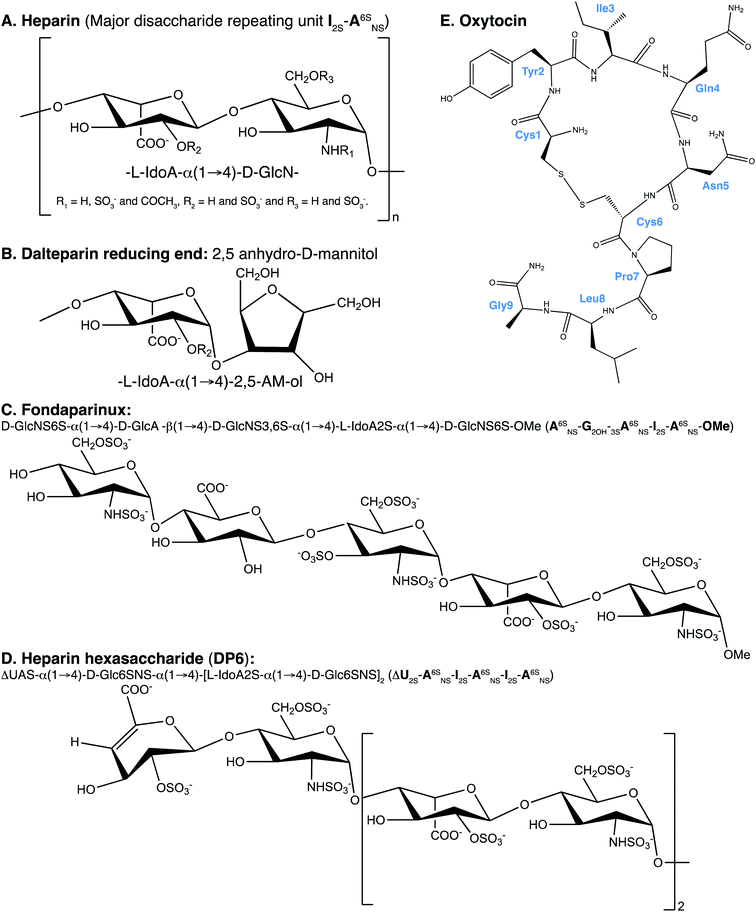 |
| Fig. 1 Illustration of the structures of (A) heparin, the major repeating unit of which is the trisulfated disaccharide I2S-A6SNS; (B) the nominal reducing end of DAL, an iduronic acid group linked to 2,5 anhydrous-D-mannitol (2,5-AM-ol), the terminal group can also be sulfated at position 6 (6-O-sulfo-2,5-anhydro-D-mannitol); (C) fondaparinux (FP), (D) the trisulfated heparin hexasaccaride DP6 and (E) OXT, where the amino acids are labelled in blue. I stands for iduronate, G for glucuronic acid, A for glucosamine, ΔU for an unsaturated uronic acid and nr indicates that the residue is at the nonreducing end of the molecule. The sub- and superscripts denote the position of sulfation (S) or acetylation (NAc), respectively. AN and IN refer to position N (either C atom or H atom depending on the context) of the glucosamine or iduronate residue, respectively. For example, I2S-A6SNS corresponds to the disaccharide 2-O sulfated iduronic acid linked to 6-O-sulfated N-sulfated glucosamine. A2* signifies position 2 of glucosamine, which is N-sulfated and O-sulfated at positions 6 and 3. | |
Low molecular weight heparins (LMWH) are heparins that have been depolymerised using chemical or enzymatic means to give rise to this class of compounds with a molecular weight of less than 8000 Da.6 They are enriched in the antithrombin binding domain (which is a consequence of the resistance of this trisulfated residue to the chemical process used) and have improved pharmaco-kinetics/-dynamics compared to unfractionated heparin. An important property of LMWHs is that they induce fewer incidences of thrombocytopenia, which is an immune response to the complex between platelet factor 4 and heparin.7
Oxytocin (OXT) is a nonapeptide hormone of the following sequence; Cys–Tyr–Ile–Gln–Asn–Cys–Pro–Leu–Gly (Fig. 1E). It forms a cyclic structure, maintained by a disulfide bond between the two cysteine residues and its C-terminus is converted to an amide. Oxytocin can be found in multiple locations in the body and has been implicated in a wealth of physiological and psychological conditions.8 It is best known for its role in uterine muscles contractility, which was first demonstrated in cats,9 and is being administered as a drug to initiate and accelerate labour.10 The biological activity of OXT is mediated through oxytocin receptors, these are G-protein-coupled receptors that also require magnesium11 and cholesterol.12
Recently, it has been shown that when low molecular weight heparin, dalteparin (DAL), is administered to pregnant women in combination with OXT, the patients exhibited greatly reduced labour times, compared to administration of OXT alone.13 The mechanism for this is two-fold; firstly low molecular weight heparin effects cervical ripening. Secondly, there are indications that heparin interacts with the oxytocin signalling pathway alone.13 This effect is also discussed in the review by Lindahl et al.,14 interestingly this review is centred on the fact that heparan sulfate has been implicated in many disease, but the use of heparin/heparan sulfate-based drugs is still underutilised. Dalteparin is produced from heparin that is extracted from porcine intestinal mucosa. The unfractionated heparin undergoes deaminative cleavage with nitrous acid, followed by reduction and a chromatographic purification process.15 The depolymerisation process results in the formation of a 2,5-anhydro-D-mannitol ring at the reducing end of the polysaccharide, which can be used to identify the LMWH16 (Fig. 1B). The main drawback in using DAL as a birthing aid is that the LMWH is an anticoagulant. To this end, a non-anticoagulant LWMH is being developed by Dilafor AB and Opocrin S.p.A, tafoxiparin. This product is currently undergoing trials, entitled ‘Effect of Tafoxiparin on Cervical Ripening and Induction of Labour in Term Pregnant Women With an Unripe Cervix’ and has been assigned the following identifier, NCT04000438.17
The work presented herein investigates a direct interaction between OXT and heparin oligosaccharides, using nuclear magnetic resonance (NMR) spectroscopy. With the aim of identifying the site of interaction in both molecules. Initially, three oligosaccharides were used; DAL, the low molecular weight heparin first observed to have a synergistic affect with OXT, fondaparinux (FP) and a heparin hexasaccharide (DP6). Fondaparinux is a pentasaccharide produced synthetically (Fig. 1C), which here, serves as a model compound for the heparin antithrombin binding site.18–20 The heparin hexasaccharide DP6 has an average composition of three disaccharide repeating units of 2-O-sulfated iduronic acid linked to 6-O- and 2-N-sulfated glucosamine [I2S-A6SNS]3 (Fig. 1D). It represents the general sequence of heparin. Dalteparin is a heterogenous mixture of large molecules not ideal for NMR studies and, since DAL is comprised of regions containing both FP and DP6, it was logical to investigate the interaction of OXT with FP and DP6, as well as with DAL.
The study between OXT and heparin was then directed towards investigating the diverse chemical space of heparin and the affect the different sequences found within heparin has on the interaction with OXT. A panel of four heparin hexasaccharides were chosen, with three of the hexasaccharides containing specific chemical modifications; de-2-O-sulfated iduronic acid ([I2OH-A6SNS]3), de-6-O-sulfated glucosamine ([I2S-A6OHNS]3) and N-acetylated glucosamine ([I2S-A6SNAc]3). The fourth member of the panel is the orthodox trisulfated heparin hexasaccharide [I2S-A6SNS]3. Utilising these four chemically defined oligosaccharides allows the sulfation pattern specificity of the interaction between OXT and heparin to be investigated, possibly providing a means of decoupling the anticoagulant properties of heparin from those involved in the interaction with OXT.
The backbone of this study involved measuring a series of 15N–1H and 13C–1H HSQC NMR spectra of OXT alone and in the presence of the different heparin derivatives at a range of different temperatures. By quantifying the chemical shift (CS) perturbations (ΔCS) we were able to observe the interaction between OXT and the polysaccharides, map the binding determinants/epitopes on the molecules and determine binding constants. By perturbing the temperature of the system, we were able to gain further insights into the interaction mechanism.
2. Methods and materials
2.1 Nomenclature
I stands for iduronate, G for glucuronic acid, A for glucosamine, ΔU for an unsaturated uronic acid and nr indicates that the residue is at the nonreducing end of the molecule. The sub- and superscripts denote the position of sulfation (S) or acetylation (NAc), respectively. AN and IN refer to position N (either C atom or H atom depending on the context) of the glucosamine or iduronate residue, respectively. For example, I2S-A6SNS corresponds to the disaccharide 2-O sulfated iduronic acid linked to 6-O-sulfated N-sulfated glucosamine. A2* signifies position 2 of glucosamine, which is N-sulfated and O-sulfated at positions 6 and 3.
2.2 Reagents
The acetate salt of OXT was purchased from Bachem (Bubendorf, Switzerland), with a disulfide bond between Cys1 and Cys6 formed. Sodium fondaparinux (A6SNS-G2OH-3SA6SNS-I2S-A6SNS-OMe) and DAL (EDQM CRS) were sourced from Sigma Aldrich, UK. While, the heparin hexasaccharides (DP6 ([I2S-A6SNS]3), DP6-NAc ([I2S-A6SNAc]3), DP6-6OH ([I2S-A6OHNS]3) and DP6-2OH ([I2OH-A6SNS]3)) were purchased from Iduron (Cheshire, UK).
2.3 NMR sample preparation
The OXT and FP samples used for assignment contained 4 and 2 mM, respectively. Samples used for binding epitope mapping contained 3.3–4 mM OXT and 0.9
:
1, 1.7
:
1 and 3.3
:
1 molar ratios of DP6/DP6-NAc/DP6-6OH/DP6-2OH, FP and DAL, respectively. Titration experiments were carried out by step-wise addition of concentrated polysaccharide solution into a 2 mM OXT sample (0.14 and 0.2 mM DAL and FP, respectively). All samples described above were dissolved in 10 mM phosphate buffer, pH 6.0 supplemented with 10% D2O and 50 μM 4,4-dimethyl-4-silapentane-1-sulfonic acid (DSS). Samples used to investigate the hydroxyl signals contained 2 mM FP and 4 mM OXT and were made up in 25% d6-acetone (v/v), pH 8.2 and 0.15 M NaCl. In this buffer system, the samples were still liquid at 285.5 K.21
2.4 NMR measurements
All NMR experiments were carried out on a Bruker Avance NEO 700 MHz spectrometer equipped with a QCI-F cryoprobe (Bruker, Coventry, UK). Water suppression was achieved using excitation sculpting with gradients.22 Phase sensitive 2D-HSQC spectra were acquired using Echo/Antiecho-TPPI gradient selection (Bruker pulse sequences – hsqcetgpsisp.2 and hsqcetfpf3gpsi for 13C and 15N, respectively). Total Correlation Spectroscopy (TOCSY) spectra were acquired using the DIPSY2 sequence, with a mixing time of 80 ms (Bruker pulse sequence – dipsi2esgpph), while Nuclear Overhauser Effect Spectroscopy (NOESY) spectra were collected with a mixing time of 200 ms (Bruker pulse sequence – noesyesgpph). Typical carrier positions used in all experiments were 117 ppm for 15N, 75 ppm for 13C and 4.7 ppm for 1H. Experiments were carried out at a range of temperatures as noted in the text. Titration experiments were carried out at 280 K and hydroxyl NMR investigation were performed at 258.5 K. Data were processed and analysed using Topspin 4.0.3 (Bruker, Karlsruhe, Germany) software.
2.5 Chemical shifts assignment
The following references were used to assign the molecules investigated in this study: OXT,23 FP,24 DAL15 and DP6/DP6-NAc/DP6-6OH/DP6-2OH.25,26
2.6 ΔCS perturbations
Chemical shift (CS) perturbations for each atom where simply calculated by subtracting the CS value of the free molecule, CSFreenuclei x, from that of the bound molecule CSBoundnuclei x. For example, for 1H: |
ΔCSH = CSBoundH − CSFreeH
| (1) |
The weighted changes in chemical shift are then calculated: |
 | (2) |
and |
 | (3) |
Residues are considered to be involved in binding if they experience a ΔCS larger than the mean plus one standard deviation for 15N–1H chemical shift perturbations and the mean plus three standard deviations for 13C–1H chemical shift perturbations.27,28 The means and standard deviations were determined temperature wise.
2.7 Evaluation of the dissociation constants
The use of chemical shift changes upon binding to evaluate dissociation constants is well established. The complex concentration can be indirectly measured from the weighted changes in chemical shifts (eqn (2)) and their dependence on the concentration of the added polysaccharide. Thus, allowing determination of its dissociation constant. The changes in chemical shift were plotted as a function of the added peptide concentration and assuming a 1
:
1 simple binding model, KD = [O][Ps]/[OPs] were fit globally to the equation: |
 | (4) |
where [O] and [Ps] represent the concentrations of OXT and the ligand polysaccharides, respectively, x = [Ps]/[O] and ΔCS0 and a = KD/[O] were fitted by Origin 2019 (Originlab, MA, US). This approach was previously implemented in Schnur et al.29
3. Results and discussion
Herein we study the interaction between OXT and the LMWH DAL, as well as the model oligosaccharides, DP6 and FP, that represent sequences found in DAL. These interactions were studied by measuring a set of 15N–1H and 13C–1H HSQC NMR spectra of the complexes at different temperatures (280–328 K in 6 K steps). The binding epitopes were mapped by comparing the differences between the chemical shifts of the free and bounds molecules (ΔCS perturbations). If the ΔCS is zero then the chemical environment around the molecule being observed has not changed on the addition of the ligand and no interaction has taken place. Analysis of the ΔCSHN perturbations allowed the peptide to be observed solely, primarily the peptide backbone. While, the ΔCSHC perturbations could be used to observe both the peptide (backbone and side chains) and carbohydrates. The signals were generally well dispersed, with no overlap between the peptide and carbohydrate features (Fig. 2). Dalteparin is a heterogenous mixture of polysaccharide chains of different length and with a variety of sequences. As such, it gives rise to broadened peaks in its NMR spectrum, which are harder to interpret and difficult to assign specifically. However, FP and DP6, which are shorter, are more amenable to NMR studies (Fig. 1). Thus, allowing us to study the reciprocal effect of OXT on FP and DP6. Furthermore, three chemical modified heparin hexasaccharides (DP6-NAc, DP6-2OH and DP6-2OH) were used, in conjunction with, DP6 to study the heparin chemical space, investigating whether any particular moiety (I2S, A6S or ANS) was vital for the interaction between OXT and heparin.
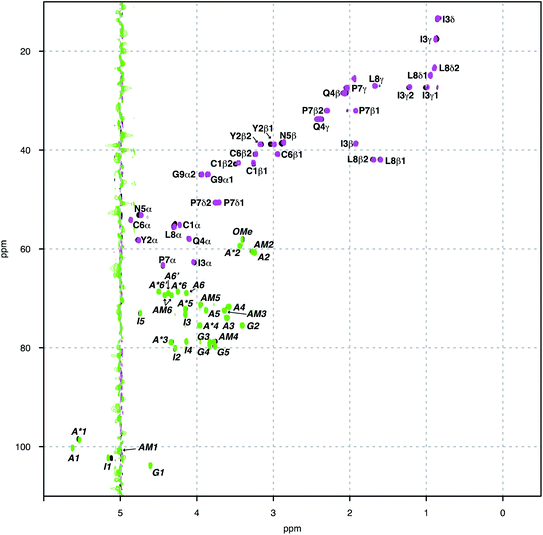 |
| Fig. 2 1H–13C-HSQC NMR spectra overlay of OXT : FP 1.7 : 1 (black), OXT only (magenta) and FP only (green). 1H–13C-HSQC NMR Spectra collected at 280 K. Peaks are labelled with assignment in regular and italic font for OXT and FP, respectively. It can clearly be seen by visual inspection that the following signals are perturbated upon interaction; FP – G4, A*1, A*3, I1, I2, I4, I5 and AM4; OXT – C1β, Y2α, Y2β, I3α, I3γ12, I3 γ13 and I3γ12, N5α, N5β, L8α. | |
3.1 Mapping the binding site of OXT to DAL, FP and DP6
The following molar ratios were used to map the binding site of OXT to DP6, FP and DAL; 0.9
:
1, 1.7
:
1 and 3.3
:
1, respectively. The rationale behind these values is related to the heterogeneity of the oligosaccharides and their respective lengths. Dalteparin has an average molecular weight of ∼6000 Da, which equates to an approximate length of 18–20 disaccharide units. When compared to DP6, which is a hexasaccharide, DAL can interact with many peptides given that it is approximately 3 times longer in length. Furthermore, DAL and DP6 are heterogenous, containing a mixture of oligosaccharides with different sulfation patterns and containing chains with the uronic acids in both iduronic and glucuronic acid formed. Unlike, FP which is a chemically synthesised pentasaccharide with a defined sequence (A6SNS-G2OH-3SA6SNS-I2S-A6SNS-OMe), therefore less of the material is required when compared to DP6 to obtain a similar response.
Analysis of the chemical shift perturbations in the 15N–1H HSQC NMR spectra (ΔCSHN) shows that the peptide interacts with DAL, FP and DP6 in a similar manner. The binding site mainly involves I3, Q4 and C6 and to a lesser extent Y2 and L8 (back bone amides, Fig. 3). Judging from the ΔCS values of I3 and Q4, the interaction between OXT and both FP and DAL becomes stronger as the temperature of the complex is decreased, suggesting it is a favourable energetic state. It should be noted that the 15N–1H backbone peak of Y2 does not appear and could not be assigned at temperatures higher than 298 or 304 K. This could imply some structural flexibility of OXT.
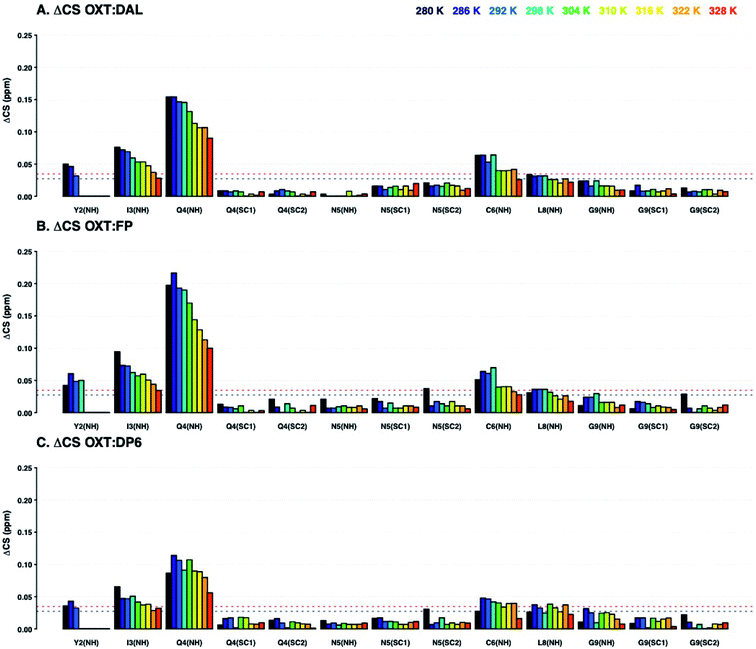 |
| Fig. 3 Change in 15N–1H chemical shifts of residues of OXT upon binding to DAL (A.), FP (B.), or DP6 (C.) at a range of temperatures (280–328 K in 6 K steps, dark blue to red, respectively). The Y axis shows ΔCS in ppm (see Materials and methods). The X axis indicated the atom in the amino acid, ‘SC’ stands for side-chain. The figure illustrates that the binding epitope in OXT for heparin-related oligosaccharides involved Y2(NH), I3(NH), Q4, C6(NH) and L8(NH). The horizontal lines indicate ΔCS values larger than the mean (black dotted lined) and larger than the mean plus one standard deviation (red dotted line). | |
Analysis of the ΔCSHC perturbations suggests that the interaction of OXT with the carbohydrate involves residues Y2 (β), I3 (α and γ) and Q4 (not seen in OXT
:
DP6) as seen with the ΔCSHN perturbations. Also, C1 (α and β) and N5 (α and β) participate in binding (Fig. 4). That is, binding of the heparin polysaccharides (DAL, FP and DP6) affects mainly the ring of OXT (residues 1–6), rather than its tail (residues 7–9). Interestingly, residues found across the ring are involved, suggesting a “face-on” interaction where the polysaccharides lie across the cyclic nonapeptide ring, a schematic of the affected atoms in OXT can be seen in Fig. 11. The first impression from a comparison of the three panels of Fig. 3 and 4, is a striking similarity between the effects of the three heparin derivatives on OXT; a not unexpected result, as the three derivatives are closely related and have much in common. However, a couple of slight differences can be found; the side-chain of Q4 is less affected in the presence of DP6 in comparison to DAL and FP, suggesting, perhaps, an involvement of either the hydroxyl groups of glucuronic acid or the triply-sulfated N-glucosamine. Likewise, the effect seen on C6 only with DAL suggests it is brought about by groups that do not appear on both FP and DP6 or, is due to the long chain oligosaccharides found in DAL.
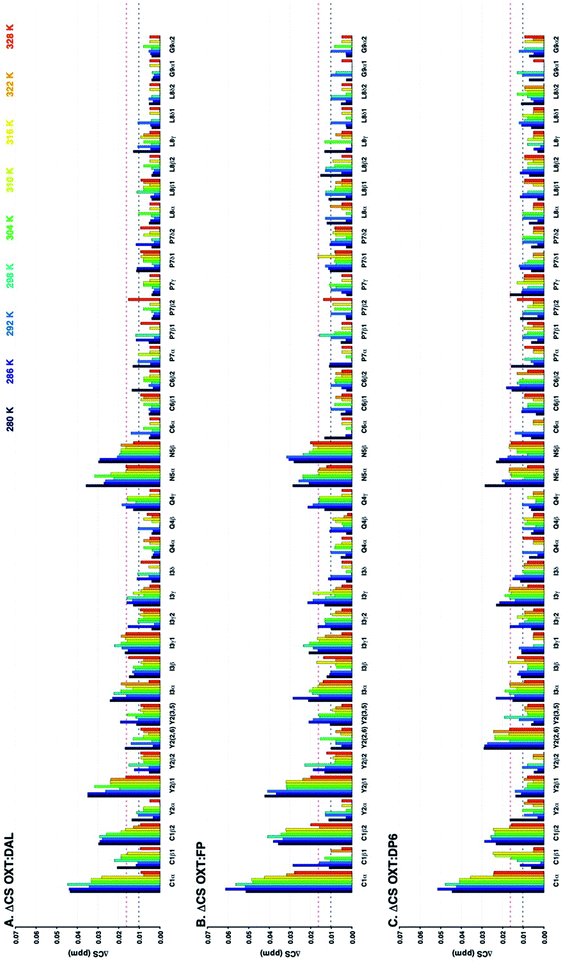 |
| Fig. 4 Changes in the 13C–1H chemical shifts of OXT residues upon binding to DAL (A), FP (B) or DP6 (C) at a range of temperatures (280–328 K in 6 K steps, dark blue to red, respectively). The Y axis shows ΔCS in ppm (see Materials and methods) and the X axis indicate the atom in the amino acid. The figure indicates that the binding OXT interaction epitope for heparin related oligosaccharides involves; C1, Y2, I3, Q4 and N5. The horizontal lines indicate ΔCS values larger than the mean (black dotted lined) and larger than the mean plus three standard deviations (red dotted line). | |
It is worth mentioning that at higher temperatures, the OXT spectrum exhibited a population of secondary peaks, that grows stronger with increased temperatures, suggesting, once more that OXT can exist in a secondary, less energetically favourable, conformation.
3.2 Mapping the binding site of DAL, FP and DP6 to OXT
In addition to observing the peptide, ΔCSHC perturbations allowed the effects of the interaction on the carbohydrate to be mapped as well. Dalteparin is not best suited for peak assignment and ΔCSHC determination with high confidence as it is a highly heterogenous mixture of polysaccharide chains with different substitutions and lengths (Fig. 1A). Therefore, model compounds approximating regions within DAL were studied, FP and DP6 (Fig. 1C and D, respectively).
Owing to its low molecular weight, the NMR spectra of FP contain sharp, well defined spectral features that allowed analysis and full assignment (example in Fig. 2 and 5B), enabling the study of the reciprocal effect of OXT on FP.
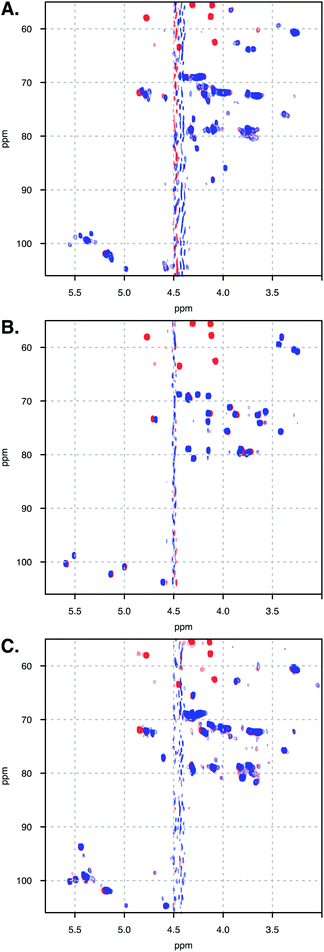 |
| Fig. 5 Spectra overlay of free (A) DAL, (B) FP and (C) DP6 (blue), and in complex with OXT (red). Spectra measured at 328 K. | |
The ΔCSHC results for FP upon binding OXT are presented in Fig. 6A. Atoms on the G2, A*3, I4 and AM5 rings of FP (Fig. 1) experience changes in their chemical shifts in the presence of OXT (Fig. 6A). The spread of the effect over the length of the polysaccharide agrees with the notion of a “face-on” interaction between the sugar and the ring of OXT.
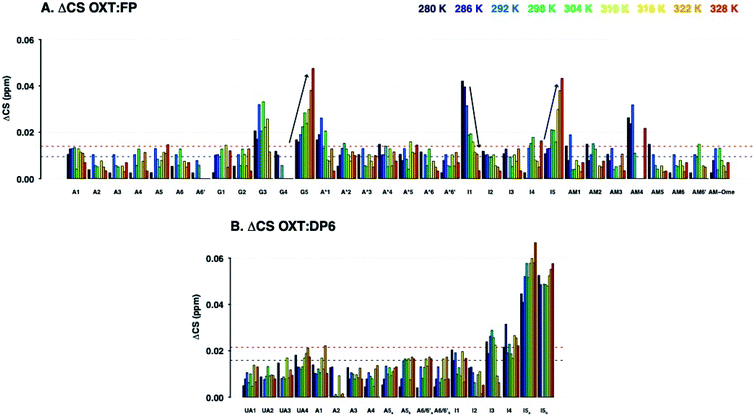 |
| Fig. 6 Change in 13C–1H chemical shifts of FP (A) and DP6 (B) residues upon addition of OXT at a range of temperatures (280–328 K in 6 K steps, dark blue to red, respectively). The Y axis showing ΔCS in ppm (see Materials and methods) and the X axis indicates the residue and atom in the polysaccharide (For DP6 (B) residue numbers not assigned). The horizontal lines indicate ΔCS values larger than the mean (black dotted lined) and larger than the mean plus three standard deviations (red dotted line).There are two different phenomena observed as the temperature increased, firstly the ΔCS for G3, I1, A1* and AM4 in FP decreases upon the change in temperature, this is similar to what is observed in OXT. Conversely, the ΔCS for G2–5 and I4–5 in FP increases as the temperature is increased, it is hypothesized that is due to changes in the hydrogen bond-network between the carboxyl groups and the surrounding water. This is also observed in the more heterogenous DP6, the ΔCS for I5a and I5b both also increase. | |
Interestingly, the trend of change with temperature differs for different atoms and two distinct effects can be seen in the OXT
:
FP interaction. Firstly, ΔCSHC decreases with increasing temperatures for I4–1 and AM5–4, as seen for OXT residues. However, the opposite is seen for position-5 of both the glucuronic and iduronic acid residues. This counter-intuitive behaviour, where ΔCSHC perturbations increase with higher temperatures, could be explained by perturbation of the hydrogen-bonding network formed by these acid groups due to OXT binding, whether by OXT interacting directly with the carboxylic groups of rings I4 and AM5 (Fig. 1) or by OXT imposing a conformational change in the saccharide that does not sustain the otherwise favourable hydrogen bond network. Interestingly, atoms A*3–1, I4–1 and AM5–4 are involved, suggesting perhaps a change in the glycosidic bonds centred around I4. It should be noted that position-5 of the uronic acid residues are very sensitive to their environment.
The heparin-derived hexasaccharide, DP6, were produced by depolymerising heparin by using heparinase 1 and then separation by high-resolution gel chromatography, therefore, they are size defined but not sequence defined, containing primarily, but not solely, the trisulfated repeating unit I2S-A6SNS (Fig. 1D). Hence, it was not surprising that its NMR spectra revealed the presence of structures which were not the majority structure I2S-A6SNS, such as GlcA (Fig. 5C). This is most likely as a result of not being chemically synthesised but rather biologically sourced.
Thus, our sample is a mixture of short polysaccharide chains with a majority of, but not exclusively, the DP6 structure. As evident from a comparison of panels A and C in Fig. 5, this leads to NMR spectra with a certain level of complexity which prevented sequence specific assignment. We were, however, able to assign families of peaks to atom types (Fig. 6B), allowing us to study the interaction of DP6 to OXT and compare it to the that of FP. As with FP, the iduronic acid seems to be affected by the presence of OXT and specifically atom 5 and to a lesser extent its neighbouring atom 4. Interestingly, peaks assigned to position-3 of glucuronic acid also experienced a change in chemical-shift similar, again, to the observation with FP (data not shown). This suggests an important role for glucuronic acid in the interaction with OXT.
Unfortunately, the heterogenous nature of DAL renders it difficult to analyse by NMR, in which this heterogeneity gives rise to peak broadening. Still, the 13C–1H HSQC NMR spectra of FP, DP6 and DAL are closely related, as clearly evident from Fig. 5. When comparing the overlaid spectra of each of the polysaccharides alone and in the presence of OXT, a great similarity is revealed. For example, the peaks around 4.8 and 72 ppm, which in FP is assigned to I5, experience a change in chemical-shift in all cases while peaks around 3.3 and 61 ppm, assigned to A2 and AM2 in FP, do not change position in all polysaccharides. Thus, we feel confident that the information learned for FP and DP6 can be used to approximate the interactions of DAL with OXT as well.
3.2.1 Sulfates are not the only chemical groups involved in the interaction between OXT and FP. Hydroxyl and amine residues are among the chemically active groups of carbohydrates, which do not appear in a 13C–1H HSQC spectrum and are solvent exchangeable, therefore, not visible under experimental conditions usually employed. However, Beecher et al. demonstrated that at low temperatures it is possible to observe the solvent exchangeable hydroxyl and amine protons present in FP.21 Although hydrogen bonds have previously been identified to be import for heparin:protein interactions,30 they are rarely investigated in solution. One-dimensional 1H and TOCSY NMR spectra of FP alone and in the presence of OXT at 258.5 K were acquired (dissolved in 25% d6-acetone (v/v) to prevent sample freezing; Materials and methods). Signals from A1–NH, A1–OH3, A1–OH4, G2–OH2, G2–OH3, I4–OH3 and A5–OH3 can be observed for FP (Fig. 7). Two phenomena can be observed upon binding of FP to OXT: change of signal intensity and position. Signals assigned to A1–NH and I4–OH3 significantly had their CSs perturbated upon binding (Fig. 7). The chemical group G2–OH2 also experienced chemical shift changes but to a lesser extent. Features arising from G2–OH2, G2–OH3 and I4–OH3 lost intensities upon binding, while A1-OH3 and A1–OH4 did not appear in the spectra of the bound oligosaccharide (Fig. 7). The changes in hydroxyl signals in the FP spectra upon addition of OXT, suggest the interaction affects the hydrogen bond network of FP or its structure. The presence of the two phenomena, peak position shift and intensity loss, suggest a complex interaction between FP and OXT with different processes occurring on different time scales.
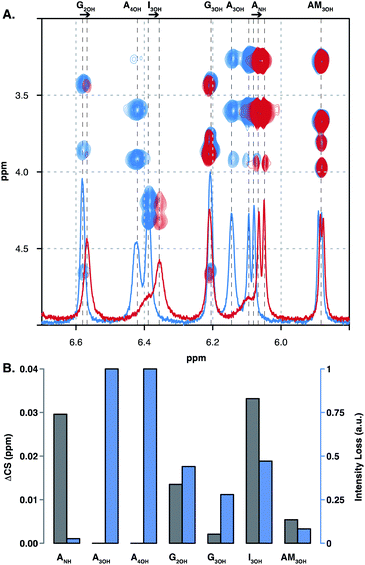 |
| Fig. 7 Low temperature NMR of the carbohydrate amine and hydroxyl groups. (A) Overlay of TOCSY and 1D-1H spectra of OXT alone (blue) and in complex with FP (red). Assignments of hydroxyl residues of FP appear on the bottom. Arrows indicate system shifts. (B) ΔCS of hydroxyl residues of FP in the F2 dimension (grey bars) and normalised signal intensity loss (blue bars; 0 = no intensity change and 1 = the signal has completely disappeared). | |
3.2.2 The effect of heparin substitution pattern on the interaction. To further investigate the interaction between heparin and OXT three chemically modified hexasaccharides were sourced (DP6-NAc, DP6-6OH and DP6-2OH), allowing the chemical space of heparin to be investigated. In these experiments the concentration ratio of OXT and the 4 ligands is the same, therefore the magnitude of the ΔCS can be compared directly. When using the 3 chemically-modified hexasaccharides it is noticeable that the unmodified hexasaccharide ([I2S-A6SNS]3) causes the greatest change in 15N–1H chemical shift perturbations (Fig. 8), indicating that the interaction between the peptide and DP6 is the strongest and that there is a charge component to the interaction, which is not unexpected. The 15N–1H chemical shift perturbation profiles for OXT
:
DP6-6OH, OXT
:
DP6-2OH and OXT
:
DP6-NAc are very similar, with the ΔCS for OXT
:
DP6-NAc being attenuated. The primary difference is seen at I3-NH and Q4-NH, suggesting that the hexasaccharide is interacting with these amino acids via the N-sulfate, located at position-2 of glucosamine.
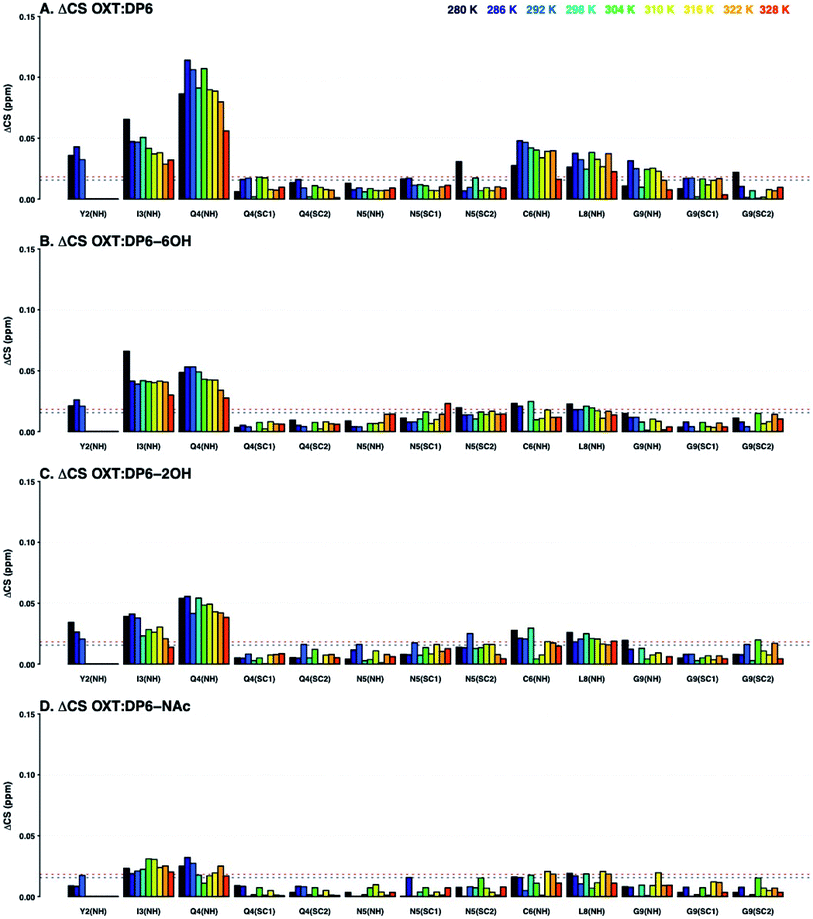 |
| Fig. 8 Change in 15N–1H chemical shifts of residues of OXT upon binding to DP6 (A), DP6-6OH (B), DP6-2OH (C) or DP6-NAc (D) at a range of temperatures (280–328 K in 6 K steps, dark blue to red, respectively). The Y axis shows ΔCS in ppm (see Materials and methods). The X axis indicates the atom in the amino acid, ‘SC’ stands for side-chain. The ΔCS are attenuated in the OXT : DP6-NAc complex (I3(NH) and Q4(NH)), indicating that N-sulfation of the glucosmine is important for the interaction with the peptide. The horizontal lines indicate ΔCS values larger than the mean (black dotted lined) and larger than the mean plus one standard deviation (red dotted line). | |
The 13C–1H chemical shift perturbation profiles are different between DP6 and the chemically-modified hexasaccharides (Fig. 9). Firstly, the fully sulfated hexasaccharide, DP6, affects the ring of the tyrosine residue at position-2 more than the chemically modified hexasaccharides. Secondly, in the interaction with the peptide's N-terminal residue, C1, the effect seen with DP6 is inverse to that observed in the presence of DP6-NAc, DP6-2OH or DP6-6OH. The inverse temperature effect indicates that as the temperature increases, the chemical environment experienced by OXT in the complex become more similar to that of OXT alone. Whereas, the effect of the interaction between DP2-2OH [I2OH-A6SNS] and DP2-NAc [I2S-A6SNAc] and OXT increases as the temperature increases. Sulfation at position-6 of glucosamine is important for the interaction between OXT and the heparin hexasaccharides, DP6-NAc and DP6-2OH greatly perturb the cysteine amino acid, which this is not seen in the OXT
:
DP6-6OH complex, as the 6-O-sulfate group is not present to interact with the N-terminus of the peptide. This effect is more specific than simple electrostatic forces causing the chemical-shift perturbations, as DP6-6OH and DP6-2OH have the same overall charge. Also, the effect DP6 has on Y2 and I3 is interesting, as neither of these amino acids is charged, and DP6 is the most charged of the heparin hexasaccharides.
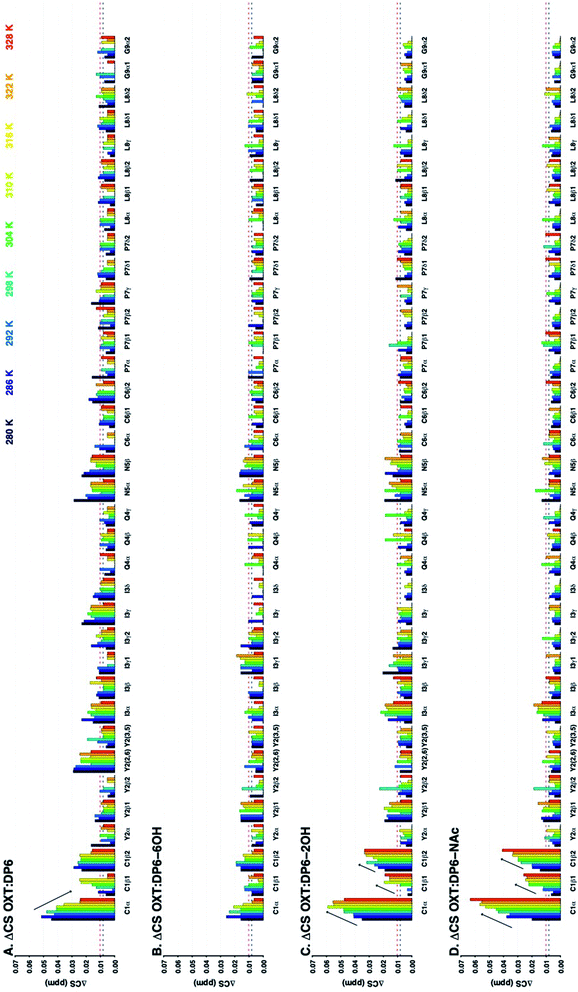 |
| Fig. 9 Change in 13C–1H chemical shifts of residues of OXT residues upon binding to DP6 (A), DP6-6OH (B), DP6-2OH (C) or DP6-NAc (D) at a range of temperatures (280–328 K in 6 K steps, dark blue to red, respectively). The Y axis shows ΔCS in ppm (see Materials and methods). The X axis indicates the atom in the amino acid. The ΔCS are attenuated in the OXT : DP6-6OH complex (C1), indicating that 6-O-sulfation of the glucosamine is important for the interaction with the peptide. In addition there is an inverse temperature effect observed at the N-terminus amino acids of the peptide for the OXT : DP6 complex (see arrows), which is not observed in the interactions between OXT and DP6-NAc, DP6-2OH or DP6-6OH. The horizontal lines indicate ΔCS values larger than the mean (black dotted lined) and larger than the mean plus three standard deviations (red dotted line). | |
The combination of measuring 15N–1H and 13C–1H chemical shift perturbations while the OXT:hexasaccharide complexes undergo a thermal gradient illustrates that all four hexasaccharides interact with OXT. It was observed that N-sulfation and 6-O-sulfation of glucosamine are important for the interaction between heparin and OXT. Removing the N-sulfate group and replacing it with an N-acetyl moiety attenuated the ΔCS at I3 and Q4 (Fig. 8), while de-6-O-sulfation diminished the ΔCS of C1 (Fig. 9). These two sulfate groups have two different effects on the polysaccharide chain. 6-O-Sulfation is a charge in space and de-6-O-sulfation causes only a local effect. This is not the case for N-sulfation, replacing the N-sulfate with an N-acetly group affects the adjacent linkage, as well as, the conformation of the neighbouring uronic acid residue.26,31,32
3.3 Binding constant determination
The binding constant (KD) was determined by analysing 15H–1H HSQC titration experiments where either DAL or FP were added in a step-wise manner to a sample of OXT. Titration experiments were carried out at 280 K, where the interaction is at its tightest and the changes in chemical shift are largest, thus providing more accurate analysis of results.
3.3.1 Three OXT residues. I3, Q4 and C6 which exhibited the biggest changes in chemical shift upon binding both DAL and FP, were chosen for the analysis. The weighted changes in chemical shifts (ΔCSHN) between free and bound OXT at each addition point were calculated using eqn (2) (Materials and methods) and plotted as a function of carbohydrate concentration (Fig. 10). The curves were fit globally to eqn (4) (Materials and methods). The fitted binding constants are Kd = 0.33 ± 0.01 mM for the interaction between OXT and FP and Kd = 0.15 ± 0.01 mM for the interaction between OXT and DAL. That is, the strength of interaction between OXT and DAL is of the same order of magnitude as the interaction between OXT and FP.
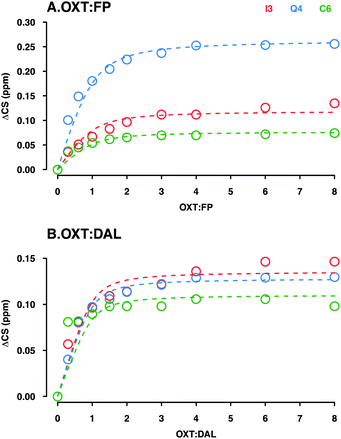 |
| Fig. 10 Determination of the KD for the interaction between OXT : FP (A) and DAL (B). Plots illustrate the ΔCS of OXT residues (I3, Q4 and C6 in red, blue and green, respectively) as a function of carbohydrate concentration. Oxytocin was titrated with either FP (A) or DAL (B). The weighted changes in chemical shifts (ΔCS) in the 15N–1H HSQC spectra were calculated using eqn (2) (Materials and methods). The curves were fit globally to ΔCS as a function of added concentration according to eqn (3) (Material and methods) using the OriginLab software. | |
As DAL contains the FP sequence, it is perhaps to be expected that the strength of interaction is on the same scale. On the other hand, DAL is a heterogeneous mixture of chains with an average length 4 times that of FP (FP is a penta-saccharide while the average chain length of DAL is 19 saccharides, sufficient to interact with several OXT molecules), which might lead to the expectation of tighter interaction with DAL as a result of more interacting regions being available. In the case of the OXT interaction, however, such reasoning might become nullified as OXT itself is very small and of comparable size to the 5-ring polysaccharide FP. If the interaction of OXT with a pentasaccharide saturates OXT, then elongation of the polysaccharide chain will not have the same effect on interaction strength as can be estimated purely by reasoning a larger number of interacting moieties or a larger interaction face.
4. Conclusions
The data shown here illustrate that OXT, a cyclic nonapeptide that is used to induce uterine contractions, interacts with the LMWH DAL and a series of oligosaccharides; FP and DP6 that mimic the major components found in DAL. This is an important observation as it has previously been shown that when OXT is administered to a patient that has previously been infused with DAL, the time taken for child birth is reduced.13 This is the first time that this interaction has been studied by NMR spectroscopy.
Using a combination of 13C–1H and 15N–1H HSQC NMR experiments, while subjecting the complex to a temperature gradient, allowed the residues in OXT that interacted with the oligosaccharides to be identified. Using 15N–1H HSQC NMR experiments Y2, I3, Q4, C6 and L8 were identified (Fig. 3 and 8) and the 13C–1H HSQC NMR experiments further identified C1 and N5 as taking part in the interaction (Fig. 4 and 9). A schematic of the binding epitope of OXT can be found in Fig. 11, with the carbohydrates interacting with the peptide across the face of the cyclic ring. These perturbations were observed for the shortest oligosaccharide investigated, the FP, indicating that the pentasaccharide can interact with the entirety of the peptide.
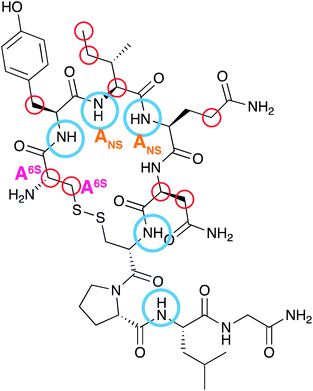 |
| Fig. 11 A schematic highlighting the collated atoms within OXT that were identified to interact with heparin. The atoms highlighted in blue were identified in the 15N–1H ΔCS experiments and the red identified in the 13C–1H ΔCS measurements. The schematic indicates the atoms that were affected by specific substituent groups of heparin. The N-terminus residue C1, was affected by de-6-O-sulation (magenta) of the heparin hexasaccharide. While the amines of I3 and Q4 were affected by de-N-sulfation (orange) of the heparin hexasaccharide. The depiction above illustrates that the interaction with heparin occurs across the face of the cyclic nonapeptide, with even the smallest oligosaccharide, FP, being able to interact with the entire length of oxytocin. | |
To investigate whether one specific substituent was important for the interaction between heparin and OXT, a series of hexasaccharides were investigated; the aforementioned DP6 with 3 chemically-modified hexasaccharides, DP6-2OH, DP6-6OH and DP6-NAc. The de-sulfation affected the interaction of all three chemically derived hexasaccharides, with DP6-NAc and DP6-6OH having the greatest effect, indicating that 6-O-sulfation and N-sulfation of glucosamine is important for the interaction between OXT and heparin. This is an interesting observation as de-N-sulfation and then N-acetylation is one mechanism for decreasing the anticoagulation activity of heparin.33 This strategy concentrates on the sulfate driven interactions between heparin and peptides/proteins and the conformational consequences of de-sulfating the carbohydrate.
Here we also investigated the interaction between OXT and FP using lower-temperature NMR experiments (258.5 K), allowing hydroxyl and amine groups to be observed. A number of these signals changed in position and/or intensity on interaction with OXT, highlighting that in addition to the sulfates, the hydroxyl and amine residues are vital components in the interaction with proteins and peptides. Furthermore, the acid groups of glucuronic and iduronic acid were observed to be perturbed on interaction with OXT, this was observed in DAL, DP6 and FP. In the chemically defined FP, the acid moieties were observed to have a positive ΔCS as the temperature increases, it is hypothesised here that this counterintuitive behaviour could be explained by a strong hydrogen-bond network, which is perturbed as the temperature is changed. These observations illustrate the multi-faceted nature of the interaction between heparin-based oligosaccharides and OXT.
Conflicts of interest
There are no conflicts to declare.
Notes and references
- T. W. Barrowcliffe, Handb. Exp. Pharmacol., 2012, 3–22, DOI:10.1007/978-3-642-23056-1_1
. - B. Mulloy, J. Hogwood, E. Gray, R. Lever and C. P. Page, Pharmacol. Rev., 2016, 68, 76–141 CrossRef PubMed
. - J. Y. van der Meer, E. Kellenbach and L. J. van den Bos, Molecules, 2017, 22, 1025 CrossRef PubMed
. - D. L. Rabenstein, Nat. Prod. Rep., 2002, 19, 312–331 RSC
. - U. Lindahl, G. Backstrom, M. Hook, L. Thunberg, L. A. Fransson and A. Linker, Proc. Natl. Acad. Sci. U. S. A., 1979, 76, 3198–3202 CrossRef CAS PubMed
. - E. Gray, B. Mulloy and T. W. Barrowcliffe, Thromb. Haemostasis, 2008, 99, 807–818 CrossRef CAS PubMed
. - J. Amiral, F. Bridey, M. Wolf, C. Boyer-Neumann, E. Fressinaud, A. M. Vissac, E. Peynaud-Debayle, M. Dreyfus and D. Meyer, Thromb. Haemostasis, 1995, 73, 21–28 CrossRef CAS
. - H. J. Lee, A. H. Macbeth, J. H. Pagani and W. S. Young III, Prog. Neurobiol., 2009, 88, 127–151 CAS
. - H. H. Dale, J. Physiol., 1906, 34, 163–206 CrossRef PubMed
. - E. J. Hayes and L. Weinstein, Am. J. Obstet. Gynecol., 2008, 198, 622.e1–622.e7 CrossRef PubMed
. - F. A. Antoni and S. E. Chadio, Biochem. J., 1989, 257, 611–614 CrossRef CAS PubMed
. - G. Gimpl, J. Reitz, S. Brauer and C. Trossen, Prog. Brain Res., 2008, 170, 193–204 CAS
. - G. Ekman-Ordeberg, M. Hellgren, A. Akerud, E. Andersson, A. Dubicke, M. Sennstrom, B. Bystrom, G. Tzortzatos, M. F. Gomez, M. Edlund, U. Lindahl and A. Malmstrom, Acta Obstet. Gynecol. Scand., 2009, 88, 984–989 CrossRef CAS PubMed
. - U. Lindahl and L. Kjellen, J. Intern. Med., 2013, 273, 555–571 CrossRef CAS PubMed
. - A. Bisio, E. Urso, M. Guerrini, P. de Wit, G. Torri and A. Naggi, Molecules, 2017, 22, 1051 CrossRef PubMed
. - M. Guerrini, S. Guglieri, A. Naggi, R. Sasisekharan and G. Torri, Semin. Thromb. Hemost., 2007, 33, 478–487 CrossRef CAS PubMed
. - L. D. Wikingsson and G. Ekman-Ordeberg, Effect of Tafoxiparin on Cervical Ripening and Induction of Labor in Term Pregnant Women With an Unripe Cervix, https://clinicaltrials.gov/ct2/show/NCT04000438, accessed 21st April, 2020 Search PubMed.
- B. Casu, P. Oreste, G. Torri, G. Zoppetti, J. Choay, J. C. Lormeau, M. Petitou and P. Sinay, Biochem. J., 1981, 197, 599–609 CrossRef CAS PubMed
. - J. Choay, M. Petitou, J. C. Lormeau, P. Sinay, B. Casu and G. Gatti, Biochem. Biophys. Res. Commun., 1983, 116, 492–499 CrossRef CAS PubMed
. - M. Petitou, P. Duchaussoy, I. Lederman, J. Choay, P. Sinay, J. C. Jacquinet and G. Torri, Carbohydr. Res., 1986, 147, 221–236 CrossRef CAS PubMed
. - C. N. Beecher, R. P. Young, D. J. Langeslay, L. J. Mueller and C. K. Larive, J. Phys. Chem. B, 2014, 118, 482–491 CrossRef CAS PubMed
. - T. L. Hwang and A. J. Shaka, J. Magn. Reson., Ser. A, 1995, 112, 275–279 CrossRef CAS
. - A. Ohno, N. Kawasaki, K. Fukuhara, H. Okuda and T. Yamaguchi, Magn. Reson. Chem., 2010, 48, 168–172 CAS
. - M. Hricovini and G. Torri, Carbohydr. Res., 1995, 268, 159–175 CrossRef CAS PubMed
. - A. Pervin, C. Gallo, K. A. Jandik, X. J. Han and R. J. Linhardt, Glycobiology, 1995, 5, 83–95 CrossRef CAS PubMed
. - E. A. Yates, F. Santini, M. Guerrini, A. Naggi, G. Torri and B. Casu, Carbohydr. Res., 1996, 294, 15–27 CrossRef CAS PubMed
. - M. P. Williamson, Prog. Nucl. Magn. Reson. Spectrosc., 2013, 73, 1–16 CrossRef CAS PubMed
. - M. P. Williamson, in Modern Magnetic Resonance, ed. G. A. Webb, Springer International Publishing, Cham, 2018, pp. 995–1012, DOI:10.1007/978-3-319-28388-3_76
. - E. Schnur, N. Kessler, Y. Zherdev, E. Noah, T. Scherf, F. X. Ding, S. Rabinovich, B. Arshava, V. Kurbatska, A. Leonciks, A. Tsimanis, O. Rosen, F. Naider and J. Anglister, FEBS J., 2013, 280, 2068–2084 CrossRef CAS PubMed
. - D. Xu and J. D. Esko, Annu. Rev. Biochem., 2014, 83, 129–157 CrossRef CAS PubMed
. - T. R. Rudd and E. A. Yates, Mol. Biosyst., 2010, 6, 902–908 RSC
. - E. A. Yates, F. Santini, B. De Cristofano, N. Payre, C. Cosentino, M. Guerrini, A. Naggi, G. Torri and M. Hricovini, Carbohydr. Res., 2000, 329, 239–247 CrossRef CAS PubMed
. - S. J. Patey, E. A. Edwards, E. A. Yates and J. E. Turnbull, J. Med. Chem., 2006, 49, 6129–6132 CrossRef CAS PubMed
.
|
This journal is © The Royal Society of Chemistry 2020 |
Click here to see how this site uses Cookies. View our privacy policy here.