DOI:
10.1039/D0RA04337K
(Paper)
RSC Adv., 2020,
10, 25952-25957
A ruthenium oxide and iridium oxide coated titanium electrode for pH measurement†
Received
15th May 2020
, Accepted 30th June 2020
First published on 9th July 2020
Abstract
This paper reports the pH sensing capability of a ruthenium oxide (RuO2) and iridium oxide (IrO2) coated titanium (ROIOT) electrode. The characterization results indicated that the ROIOT electrode had a cracked morphology. The RuO2 and IrO2 particles were decorated on the surface of the electrode. The ROIOT electrode showed near-Nernstian sensitivity of −50.8 mV pH−1, with a wide detection range of pH 2–12. The response time was 4.0–13.5 s, which was fast and very sensitive to the pH change. The ROIOT electrode also demonstrated great detection reversibility and stability in various pH conditions. In the long-term experiment of 30 d, potential measurements using the ROIOT electrode had a minor fluctuation of 1.5 mV d−1. The practical application of the ROIOT electrode was demonstrated by measuring the pH values of various buffer solutions and complex samples. With the advantages of low cost and simple production, it is believed that the ROIOT electrode could be a promising candidate for use as a sensing material for pH sensor development.
Introduction
The pH value is an important parameter of aqueous solutions, indicating the concentration of hydrogen ions.1 Accurate measurement of the pH value is a basic requirement in many applications, including scientific research and industrial production. First developed by Haber and Klemensiewicz in 1909,2,3 the glass electrode-based pH meter is the most conventional instrument for pH measurement and has excellent properties of high accuracy, good reliability, ease of use and long lifetime.4,5 However, some disadvantages still exist in the glass electrode-based pH sensor.6,7 Glass is fragile and rigid, which is unfavourable for miniaturization. The glass bulb should be stored in an aqueous solution to maintain the hydration of the outer surface. To overcome the drawbacks of the glass electrode, great effort has been devoted to searching for ideal candidates for pH sensing materials. In recent decades, people have reported many types of pH responsive materials,3 such as metal oxides,7,8 polymers9 and carbon-based materials.10 For the most pH responsive polymers and carbon-based materials, the change of pH greatly influences the conductivities. The pH condition determines the reduced or oxidized states of polymers and alters the electrical conductivities,9 and the adsorption of hydroxyl and hydroxonium ions can induce the change of conductivities in carbon-based materials.10 For the metal oxides, redox equilibrium with hydrogen ions between different metal valences is the main pH sensing mechanism. Compared with the other materials, metal oxides are regarded as promising alternatives to the glass electrode because of their potentiometric sensing capability, short response time, good Nernst response and high stability.3
Among the metal oxides, RuO2 and IrO2 have received great attention from researchers in pH measurement.6 The RuO2 and IrO2 showed superior sensitivity, a wide pH detection range and long-term stability, but the high cost of ruthenium and iridium are the major issues that limit their practical applications.11 To reduce the cost and facilitate the preparation, it is necessary to adopt an efficient method for the production of RuO2 and IrO2 electrodes. Many approaches have been reported for the preparation of RuO2 or IrO2 electrodes for pH measurement, such as electrochemical deposition,12 thermal oxidation,13 sputtering deposition14 and the sol–gel process.15 Compared with other methods, industrial production offered a low-cost and simple way for metal oxide electrode preparation, which formed a metal oxide thin film on the electrode surface via thermal decomposition of a metal precursor. The ROIOT electrode, which has been applied in wastewater treatment and the chlor-alkali industry, is fabricated by thermal decomposition of ruthenium and iridium chloride into ruthenium and iridium oxide.16–18 A simple and mature manufacturing technique endows the ROIOT electrode with the advantages of low cost and high quality, and it could be a reliable and promising material resource for pH sensor fabrication.
In the current work, the pH sensing capability of the ROIOT electrode was studied. The ROIOT electrode had linear potential readouts with pH values in the detection range of pH 2–12, with a sensitivity of −50.8 mV pH−1. The response time was found to be 4.0–13.5 s, and great detection reversibility and stability were also demonstrated with the ROIOT electrode. In the long-term study, the ROIOT electrode maintained a stable sensitivity with a potential drift of 1.5 mV d−1 for 30 d. The practical application of the ROIOT electrode was finally demonstrated by measuring the pH of complex samples and comparing the results with those obtained using a commercial pH meter.
Results and discussion
Characterization of the ROIOT electrode
The production of the ROIOT electrode was simple and cost-effective. In the routine fabrication, the first step was to polish and clean a titanium substrate. Ruthenium and iridium chloride salts were dissolved in alcohol to form the precursor solution, and the titanium substrate was treated by applying the precursor solution with a brush. After the coating solution was air dried, heat treatment at temperatures above 350 °C were conducted to decompose the precursor solution into RuO2 and IrO2. The previous cycle of precursor solution coating and heat treatment was repeated multiple times to control the deposition amounts of RuO2 and IrO2.16,19 Thus, a layer of RuO2 and IrO2 was formed on the surface of the titanium substrate.
The morphology of the ROIOT electrode was characterized with a scanning electron microscope (SEM), and the results are shown in Fig. 1a. The ROIOT electrode showed mud-flat cracking morphology with crystals decorated on the surface, which should be RuO2 or IrO2 particles according to the literature.17,18 Energy dispersive X-ray (EDX) analysis was also used to examine the element compositions of the electrodes (Fig. S1, ESI†). Compared with the uncoated titanium electrode, the EDX spectrum of the ROIOT electrode contained the new peaks of the ruthenium and iridium elements, indicating the existence of RuO2 or IrO2 on the electrode surface. The X-ray diffraction (XRD) analysis was used to study the crystalline structure of the ROIOT electrode (Fig. 1b). In the XRD pattern of the ROIOT electrode, diffraction peaks marked (c) were attributed to titanium (PDF# 44-1294, P63/mmc). Due to their similar crystal structures, RuO2 (PDF# 40-1290, P42/mnm) and IrO2 (PDF# 43-1019, P42/mnm) had overlapped diffraction peaks (peaks a + b), which indicated the presence of RuO2 and IrO2 on the ROIOT electrode.
 |
| Fig. 1 (a) The SEM image of the ROIOT electrode. (b) The XRD pattern of the ROIOT electrode. | |
Electrochemical characterization
The RuO2 and IrO2 were the sensing materials, which were responsible for the pH sensing capability of ROIOT electrode. Hydrogen ions were involved in the redox equilibriums of RuOx or IrOx at different metal valences, as shown in the following reactions:11
RuOx: 2RuO2 + 2H+ + 2e− = Ru2O3 + H2O |
IrOx: 2IrO2 + 2H+ + 2e− = Ir2O3 + H2O |
The electrode potential E can be obtained from the Nernst-equation:
where
E0 is the standard electrode potential,
T is the absolute temperature,
R is gas constant, and
F is Faraday's constant.
E0 was determined by the amount of the redox couple species and their degree of hydration. The drift of
E0 could be generated from the change of surface oxidation state, which greatly influenced the sensing stability and accounted for the potential variation.
7,20 According to reports in the literature, the number of the electron transferred,
z, was 1 in the redox mechanisms for both RuO
2 and IrO
2.
7 Therefore, the theoretical Nernstian sensitivity was calculated to be −59 mV pH
−1 when the temperature,
T, was 298 K.
Fig. 2a shows the cyclic voltammogram (CV) of the ROIOT electrode in 0.5 M H2SO4 at a scan rate of 50 mV s−1. Two redox peaks were observed over the scan range. This experimental result was consistent with previously reported work.21 The anodic peak was located at +0.67 V, and the cathodic peak appeared at +0.55 V. According to the literature, redox peaks of RuO2 and IrO2 in acid solution appeared at about +0.5 V and +0.7 V, respectively.11,20,22 The redox peaks of the ROIOT electrode covered broad potential ranges, which might result from the overlap of the redox peaks for RuO2 and IrO2.
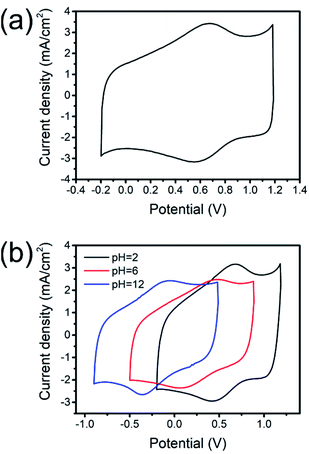 |
| Fig. 2 Cyclic voltammograms of the ROIOT electrode in different solutions. (a) 0.5 M H2SO4, (b) pH = 2, 6 and 12 buffer solutions. Scan rate: 50 mV s−1. | |
The cyclic behaviour of the ROIOT electrode was further investigated by comparing the CVs in different pH buffer solutions (Fig. 2b). The positions of the redox peaks were associated with the pH conditions. The redox peaks of the ROIOT electrode shifted to a negative potential with the increase of pH, indicating the pH sensing ability of the ROIOT electrode.
Sensing performance
The pH response capability of the ROIOT electrode was studied by measuring the potential in a gradient of pH buffer solutions (Fig. 3a). The ROIOT electrode and uncoated titanium electrode were tested in the pH range of 2–12, and the potential responses are shown in Fig. 3b. The titanium electrode showed weak potential responses with pH, and the pH response capability of the titanium electrode might result from the existence of TiO2 species on the surface.23,24 The sensitivity of the titanium electrode was −16.9 mV pH−1, and the potential-pH correlation coefficient was 0.977. Compared with the titanium electrode, the ROIOT electrode had a higher pH sensitivity of −50.8 mV pH−1, with a better correlation coefficient of 0.998. The introduction of the RuO2 and IrO2 layer could significantly enhance the pH sensing performance of the electrode. The potential responses of three other ROIOT electrodes were also measured in pH = 2–12 buffer solutions. As shown in Fig. S2 (ESI),† the consistent sensitivities and response behaviours indicated the sensing reproducibility of the ROIOT electrode. Compared with the theoretical Nernstian sensitivity of −59 mV pH−1 at 298 K, the ROIOT electrode demonstrated a near-Nernstian pH response capability with high accuracy.
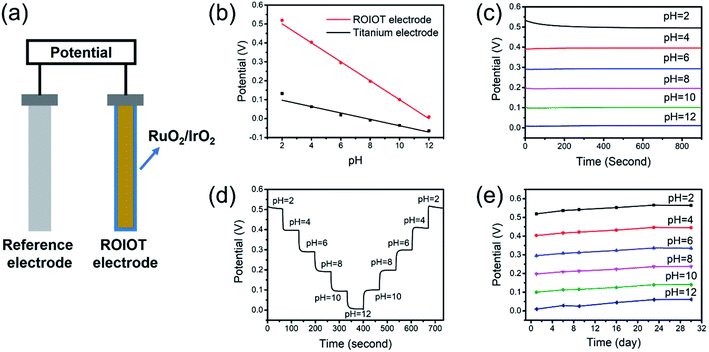 |
| Fig. 3 (a) Schematic illustration of experimental setup. (b) Potential responses of the ROIOT electrode and the titanium electrode in a gradient of pH buffer solutions. (c) Real-time potential responses of the ROIOT electrode in pH 2–12 for 15 min. (d) Potential response of the ROIOT electrode to pH changes. (e) Long-term stability of ROIOT electrode for 30 days. | |
Fig. 3c shows the short-term responses of the ROIOT electrode in the range of pH 2–12. The ROIOT electrode demonstrated stable potential responses in pH 2–12 buffer solutions. No obvious fluctuation occurred during the 15 min of measurement, which indicated the high stability of the ROIOT electrode. The response times of the ROIOT electrode were investigated by dipping the ROIOT electrode between acidic and alkaline solutions, as shown in Fig. S3 (ESI).† The potential of the ROIOT electrode gradually became stable after the change of pH. Potential variation between initial potential and final stable potential was regarded as the total potential change. Response time was defined as the time taken for the ROIOT electrode reaching 90% of total potential change, starting from the initial potential.15,25 The response times of the ROIOT electrode were 13.0 s, 4.0 s, 13.5 s and 6.7 s for the change of pH 2–12, pH 12–2, pH 4–10 and pH 10–4 respectively, which demonstrated the fast response of the ROIOT electrode to pH change. In addition, the ROIOT electrode showed shorter response times for switching from alkaline solution to acid solution than from acid to alkaline.
Response reversibility was evaluated by conducting a pH cycling test on the ROIOT electrode. The ROIOT electrode was tested consecutively in a gradient of pH solutions from 2 to 12 and then back to 2 without rinsing and cleaning. As shown in Fig. 3d, the ROIOT electrode presented quick and precise potential responses upon every pH change, and reproducible potential responses were observed at every pH level, which indicated the capability of the ROIOT electrode for repeatable and wide-range pH measurement.
Long-term accuracy and stability are important properties for pH sensors in practical applications. The potential response variations of the ROIOT electrode were investigated at the pH level of 2–12 for 30 d. The potential stability of the Ag/AgCl reference electrode was first studied with use of another Ag/AgCl electrode. Fig. S4 (ESI)† shows the stability potential of the Ag/AgCl reference electrode in the pH 2–12 range, and the potential drift of Ag/AgCl was less than 3 mV in 7 d, which indicated the potential drift of Ag/AgCl reference electrode was small and negligible. Fig. 3e shows the potential values of the ROIOT electrode in different pH conditions over 30 d, and the corresponding potential calibration curves are shown in Fig. S5 (ESI).† The sensitivity of the ROIOT electrode was found to be stable with a tiny variation, ranging from −50.4 to −51.5 mV pH−1. The potential drifts of about 1.5 mV d−1 were observed in the test pH gradients during the 30 d experiment. After long-time usage, pH re-calibration was suggested to eliminate the potential shift and to obtain more accurate measurements. Overall, the ROIOT electrode could maintain a stable and high sensitivity in the long-term experiment.
Practical application of the ROIOT electrode
The ROIOT electrode was used to measure the pH values of complex samples for demonstration of its use in practical applications. The pH results were obtained by transforming the potential readouts of the ROIOT electrode using a calibration curve. A glass electrode-based pH meter was used as a comparison to evaluate the accuracy. Table 1 lists the experimental results from the ROIOT electrode and the glass electrode-based pH meter. The ROIOT electrode demonstrated satisfactory pH measurements in the practical application. Based on the ROIOT electrode measurement, the pH values of conventional buffer solutions were found to be 4.19, 7.16 and 8.75 for potassium biphthalate, phosphate and sodium tetraborate buffer solutions, respectively, with no more than 0.3 pH unit variation with the glass electrode-based pH meter. The measurement of complex samples was also carried out as a further demonstration. The test samples covered a wide pH range with an even pH distribution. The ROIOT electrode gave consistent pH measurements with the glass electrode-based pH meter. The pH values obtained were found to be 2.24, 3.37, 4.32, 6.54, 7.64, 9.09 and 10.73 for vinegar, apple juice, beer, milk, tap water, soapy water and washing detergent, respectively. The ROIOT electrode was also used to monitor the pH adjustment of a wastewater sample. Alkaline degreaser and acidic rust remover from electroplating were used as examples to demonstrate the practical application. The results are shown in Fig. S6 (ESI).† The potential of the ROIOT electrode showed rapid responses to the pH variation of the sample solutions, and the pH results from the ROIOT electrode were consistent with those from the glass electrode-based pH meter, which further showed the practical pH sensing capability of the ROIOT electrode. Thus, it is believed that the ROIOT electrode could be used as a practical pH sensing electrode based on the following reasons. Firstly, the chemical stability provided the ROIOT electrode with a wide pH detection range, which could meet the needs of pH measurement in most situations. Secondly, the high sensitivity allowed the ROIOT electrode to give reliable and accurate measurements, which were comparable with the results from the glass electrode-based pH meter. Thirdly, the ROIOT electrode could be used for pH measurements multiple times with high sensing stability and long service life.
Table 1 Practical pH measurements from the ROIOT electrode and the glass electrode-based pH meter
Samples |
ROIOT electrode (pH) |
pH meter (pH) |
Potassium biphthalate buffer |
4.19 |
4.06 |
Phosphate buffer |
7.16 |
6.86 |
Sodium tetraborate buffer |
8.75 |
9.00 |
Vinegar |
2.24 |
2.65 |
Apple juice |
3.37 |
3.31 |
Beer |
4.32 |
4.43 |
Milk |
6.54 |
6.64 |
Tap water |
7.64 |
7.05 |
Soapy water |
9.09 |
8.98 |
Washing detergent |
10.73 |
10.62 |
Comparison with the reported works
To evaluate the pH sensing capability of the ROIOT electrode, the results obtained from the ROIOT electrode for IrO2 and RuO2 were compared with those reported in the literature. Table 2 summarizes the critical pH sensing parameters from the reports in the literature and from the present work. The ROIOT electrode showed a similar and comparable pH sensing performance with IrO2 or RuO2 with results reported in the literature. Due to the chemical stability of IrO2 and RuO2, the pH detection range was 2–12, which was wide and could meet the needs of most applications. The ROIOT electrode also had a near-Nernstian sensitivity of −50.8 mV pH−1 and second-scale response times, and was capable of fast and accurate pH measurement. In terms of stability, potential drift commonly existed in previously reported measurements of IrO2 and RuO2. These experimental results obtained in the present work demonstrated stable potential readouts in short-term measurements. For long-term stability, the ROIOT electrode showed a reasonable and minor potential drift of 1.5 mV d−1. Compared with the results reported in the literature, the simple preparation and low cost were the main advantages for the ROIOT electrode. Ruthenium oxide or iridium oxide electrode preparation via electrodeposition or sputtering required expensive instruments and complicated procedures. Thermal decomposition of metal precursors on the titanium substrate offers a simple way for ROIOT electrode fabrication, and the use of a noble metal precursor was much more efficient and cost-effective.
Table 2 Summary of the sensing performance in iridium oxide, ruthenium oxide and in this work
Sensing materials |
Detection range (pH) |
Sensitivity (mV pH−1) |
Response time (s) |
Iridium oxide15 |
1.5–12 |
51.1–51.7 |
<2 |
Iridium oxide12 |
2.38–11.61 |
59.5 |
<2 |
Ruthenium oxide14 |
2–12 |
54.83–68.63 |
3–73.5 |
Ruthenium oxide26 |
2–10 |
58 |
<2 |
This work |
2–12 |
50.8 |
4.0–13.5 |
Conclusions
In conclusion, a pH sensor based on the ROIOT electrode is reported. The ROIOT electrode showed a cracked morphology, with RuO2 and IrO2 particles, which accounts for the pH sensing capability. Linear potential responses were found in the pH range of 2–12, and the sensitivity was −50.8 mV pH−1. The sensing stability and reversibility of the ROIOT electrode were proved under different pH conditions. The ROIOT electrode showed fast potential responses upon the change of pH values, with response times of 4.0–13.5 s. In the long-term study, the sensitivity of the ROIOT electrode remained stable without great fluctuation, and the ROIOT electrode presented a potential drift of 1.5 mV d−1. Practical application of the ROIOT electrode was demonstrated in the pH measurement of buffer solutions and complex samples, which yielded consistent experiment results when compared with the conventional glass electrode-based pH meter. The ROIOT electrode had a good pH sensing capability with the advantages of low cost and stability. It is believed that the ROIOT electrode could be a reliable and promising candidate material in pH sensor development.
Experimental
Materials
The ROIOT electrodes were from the Zhongrui Electrode Industrial Technology Company, Dongguan, China. Sodium phosphate tribasic, sodium tetraborate and sodium acetate anhydrous were purchased from the Damao Chemical Reagent Factory, Tianjin, China. Sodium hydroxide (NaOH) was obtained from the Fuchen Chemical Reagents Co. Ltd., Tianjin, China. Hydrochloric acid (HCl) was obtained from Donghong Chemical Factory, Guangzhou, China.
A series of standard pH buffer solutions (pH = 2, 4, 6, 8, 10 or 12) were prepared by adjusting the pH value of 0.04 M H3PO4–HAc–H3BO3 with NaOH or HCl solution. A glass electrode-based pH meter (PHS-3E, INESA Scientific Instrument Co. Ltd., China) was used to measure the pH values of buffer solutions.
Cyclic voltammetry study
Cyclic voltammetry experiments were carried out using a potentiostat (VersaSTAT 4, Princeton Applied Research). The potential of the ROIOT electrode was cycled between −0.2 V to 1.2 V vs. an Ag/AgCl reference electrode (with 3 M KCl internal solution) in 0.5 M H2SO4 at a scan rate of 50 mV s−1. The CV behaviour of the ROIOT electrode in pH buffer solutions (pH = 2, 6 and 12) was also determined for comparison.
Potentiometric measurements
The pH sensing property of the ROIOT electrode was studied by measuring the open-circuit potentials against a Ag/AgCl reference electrode (with a 3 M KCl internal solution) in different pH solutions. Potential readouts were measured and recorded by a digital multimeter (DMM6500, Keithley Instruments). All the experiments were conducted at room temperature.
The sensing stability of the ROIOT electrode was investigated in over short-term and long-term periods. In the short-term experiment, the potential responses were recorded for 15 min while the ROIOT electrode and the Ag/AgCl reference electrode were immersed in pH = 2–12 buffer solutions. In the long-term experiment, the ROIOT electrode and the Ag/AgCl reference electrode were immersed in buffer solutions to obtain the potential responses at the time of the data acquisition. A buffer solution of pH 8 and a 3 M KCl solution were used to store the ROIOT electrode and the Ag/AgCl reference electrode, respectively, after the measurements.
For the practical application measurement of the ROIOT electrode, a pH calibration curve was first obtained by measuring the potentials over a gradient of pH buffer solutions (pH 2–12). Test samples included conventional buffer solutions and practical samples. After measuring the potential of the ROIOT electrode in complex samples, the pH values were calculated based on the calibration curve. For the pH adjusting experiment, HCl and NaOH solution were added into degreaser and rust remover, respectively, to induce the pH variation, and the potential of the ROIOT electrode was monitored and recorded during six additions of HCl or NaOH solution.
Conflicts of interest
There are no conflicts to declare.
Acknowledgements
This research was supported by Shenzhen Angel Drinking Water Industrial Group Corporation (Shenzhen, China).
Notes and references
- M. Yuqing, C. Jianrong and F. Keming, J. Biochem. Biophys. Methods, 2005, 63, 1–9 CrossRef PubMed.
- F. Haber and Z. Klemensiewicz, Z. Phys. Chem., 1909, 67, 385–431 CAS.
- Y. Qin, H. J. Kwon, M. M. R. Howlader and M. J. Deen, RSC Adv., 2015, 5, 69086–69109 RSC.
- G. A. Perley, Anal. Chem., 1949, 21, 559–562 CrossRef CAS.
- G. Johansson, B. Karlberg and A. Wikby, Talanta, 1975, 22, 953–966 CrossRef CAS PubMed.
- W. Vonau and U. Guth, J. Solid State Electrochem., 2006, 10, 746–752 CrossRef CAS.
- P. Kurzweil, Sensors, 2009, 9, 4955–4985 CrossRef CAS PubMed.
- S. Głáb, A. Hulanicki, G. Edwall, F. Folke, I. Ingman and W. F. Koch, Crit. Rev. Anal. Chem., 1989, 21, 29–47 CrossRef PubMed.
- O. Korostynska, K. Arshak, E. Gill and A. Arshak, Sensors, 2007, 7, 3027–3042 CrossRef CAS PubMed.
- P. Salvo, B. Melai, N. Calisi, C. Paoletti, F. Bellagambi, A. Kirchhain, M. G. Trivella, R. Fuoco and F. Di Francesco, Sens. Actuators, B, 2018, 256, 976–991 CrossRef CAS.
- R. H. G. Mingels, S. Kalsi, Y. Cheong and H. Morgan, Sens. Actuators, B, 2019, 297, 126779 CrossRef CAS.
- T. Y. Kim and S. Yang, Sens. Actuators, B, 2014, 196, 31–38 CrossRef CAS.
- M. Wang, S. Yao and M. Madou, Sens. Actuators, B, 2002, 81, 313–315 CrossRef CAS.
- A. Sardarinejad, D. K. Maurya and K. Alameh, Sens. Actuators, A, 2014, 214, 15–19 CrossRef CAS.
- W. D. Huang, H. Cao, S. Deb, M. Chiao and J. C. Chiao, Sens. Actuators, A, 2011, 169, 1–11 CrossRef CAS.
- R. F. Savinell and J. A. Adams, J. Electrochem. Soc., 1990, 137, 489–494 CrossRef CAS.
- T. Arikawa, Y. Takasu, Y. Murakami, K. Asakura and Y. Iwasawa, J. Phys. Chem. B, 1998, 102, 3736–3741 CrossRef CAS.
- K. Kameyama, J. Electrochem. Soc., 1994, 141, 643 CrossRef CAS.
- L. D. Burke and O. J. Murphy, J. Electroanal. Chem., 1979, 96, 19–27 CrossRef CAS.
- M. L. Hitchman and S. Ramanathan, Electroanalysis, 1992, 4, 291–297 CrossRef CAS.
- C. C. Hu, Y. H. Huang and K. H. Chang, J. Power Sources, 2002, 108, 117–127 CrossRef CAS.
- P. Shakkthivel and S. M. Chen, Biosens. Bioelectron., 2007, 22, 1680–1687 CrossRef CAS PubMed.
- M. J. Avena, O. R. Cámara and C. P. De Pauli, Colloids Surf., 1993, 69, 217–228 CrossRef CAS.
- R. Zhao, M. Xu, J. Wang and G. Chen, Electrochim. Acta, 2010, 55, 5647–5651 CrossRef CAS.
- S. Yao, M. Wang and M. Madou, J. Electrochem. Soc., 2001, 148, H29 CrossRef CAS.
- E. Tanumihardja, W. Olthuis and A. van den Berg, Sensors, 2018, 18, 2901 CrossRef PubMed.
Footnote |
† Electronic supplementary information (ESI) available. See DOI: 10.1039/d0ra04337k |
|
This journal is © The Royal Society of Chemistry 2020 |
Click here to see how this site uses Cookies. View our privacy policy here.