DOI:
10.1039/D0RA08693B
(Paper)
RSC Adv., 2020,
10, 43420-43424
Hydrated negative air ions generated by air–water collision with TiO2 photocatalytic materials†
Received
12th October 2020
, Accepted 11th November 2020
First published on 8th December 2020
Abstract
Photocatalytic materials are often used in the field of electrolysis of water for its competitive performance and low cost. This study describes the use of TiO2 for providing free electrons to prepare hydrated negative air ions together with the Lenard effect caused by air–water collision. The lifetime of HNAIs increased by 47.62% in comparison with the traditional corona discharge method; both the stability and the actual yield of the HNAIs increased significantly. The stability of HNAIs has a correlative relationship with the molecular weight and relative humidity. Lower mobility of the HNAIs with larger molecular weight results in low probability of collision with other air particles, making it relatively stable. Water molecules could form a water shell around the cluster ions in a high relative humidity environment, which can protect the ions, avoiding physical collision to extend the lifetime.
Introduction
Negative air ions (NAIs) are very important natural components of atmospheric air, which have attracted considerable attention due to their potential health benefits.1–3 In recent years, NAIs have been widely used in air cleaning,4,5 such as aerosol particle removal,6 including airborne microorganisms,7,8 and volatile organic compound degradation.9 Based on these advantages, numerous household commercial electric appliances are equipped with NAI generating modules as a propaganda gimmick. Usually, there are two types of NAI generating methods: one is natural preparation, such as thunder, cosmic radiation, waterfalls, and rains, and the other one is artificial preparation, which includes electrical corona discharge generation (ECD) and water-generated NAIs (WNAIs).10,11
Although corona discharge is the most common method for the NAI preparation and has been broadly applied to physiotherapy, the disadvantages of ECD cannot be ignored. Due to the successive molecule motion, electrons released from the electrical tip will bind with the air molecules rapidly and will quench in just a few seconds, resulting in short lifetime of NAIs and very unstable. Ozone and other by-products that have an adverse effect on health were produced during the preparation process. Nevertheless, the NAIs generated by water sheering have a long lifetime but without by-products. The mechanism of WNAIs can be explained by the Lenard effect, that droplets undergo charge redistribution forming ultrafine dipoles with negative charges. The NAIs generated by water-related methods are surrounded by dozens of water molecules, called as hydrated negative air ions (HNAIs), which have a low probability to collide with the other molecules in air. Thence, the HNAIs are more stable than the electrically generated NAIs (ENAIs), and the HNAIs can survive for several minutes but ENAIs disappear in just a few seconds.12
This research proposes a new method to generate NAIs by combining air–water collision with rosin-TiO2, as shown in Fig. 1. TiO2 has drawn increasing interest for its application towards energy and environmental areas, such as photo-catalysis, due to its low cost, high abundance, high chemical stability, and non-toxicity. In this study, TiO2 powder was fixed on the surface of rosin pedestal and 365 nm ultraviolet rays were focussed on TiO2 for exciting electrons from the valence band to the conduction band, and the free electrons were used for increasing the yield of HNAIs. Besides, airflow at a high speed was applied to split the droplets into ultrafine droplets. When these two phases of air and water liquid collide on the surface of the rosin-TiO2 pedestal, the extra electrons will combine with the ultrafine droplets to form hydrated negative air ions, such as O2−·(H2O)n, OH−·(H2O)n. These HNAIs have the following merits: (1) they can propagate for long distance and survive longer; (2) have relatively stable yields and without by-products. The influencing factors such as the molecular weight and relative humidity were also discussed in detail, which provide a reference for the sustainable improvement of indoor air quality in the future.
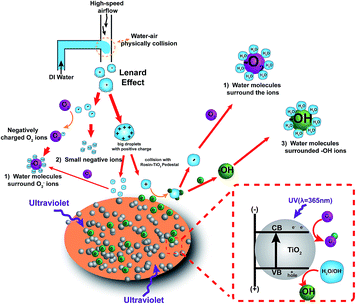 |
| Fig. 1 Schematic showing the rosin-TiO2 photocatalytic materials for HNAI generation. The HNAIs including three parts: (1) water molecules surrounded O2−, (2) small negative air ions, both (1) and (2) are come from Lenard effect; (3) water molecules surrounded OH−, part of (1) and (3) are generated by photoelectronics. | |
Results and discussion
Preliminary experimental results illustrated an obvious difference for the stability of NAIs generated by electrical corona discharge and this method, as shown in Fig. 2a. Millions of electrons released from the tip of the carbon fiber electrode combine with the air molecules to create negatively charged air ions. Molecules with strong electronegativity are more feasible to combine with the free electrons; the common ENAIs are O2−, O3−, and NO2−. Electrons can be transferred from one molecule to another during the molecular motion because free electrons bind to air molecules by weak interactions. During the transfer process, electrons are often quenched due to the loss of momentum, resulting in a decrease in the concentration of the NAIs. As the Brownian motion of the charged molecule is random, it is difficult to keep the intensity of the ENAIs stable during continuous detection.
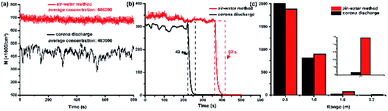 |
| Fig. 2 Comparison of physical properties of NAIs prepared by corona discharge and air–water collision with rosin-TiO2. (a) Average intensity and stability of ENAIs and HNAIs; (b) lifetime of ENAIs and HNAIs at same density; (c) straight propagation distance of ENAIs and HNAIs. All experiments were performed at room temperature in laboratory (March, 2018, Beijing). | |
However, when airflow with a high speed split the big droplets into numerous ultrafine droplets, numerous free-electrons are instantly arranged on the surface of the ultrafine droplets in a dipolar fashion. Due to the Lenard effect, the charged droplets or electrons combine with the active oxygen molecules to form HNAIs. Usually, HNAIs are surrounded by dozens of water molecules and can efficiently convert to cluster ions containing several water molecules, such as O2−·(H2O)n and OH−·(H2O)n.12 The inertia of the molecular motion is related to the weight of the cluster ions, as HNAIs are much heavier than ENAIs, thence, there is almost no probability to cause electron quenching due to the momentum consumption. It is hard for the electrons attached with the water molecules to transform between different cluster ions; therefore, HNAIs are more stable than ENAIs.
The lifetime of NAIs at same concentration prepared by the corona discharge and air–water method are compared in Fig. 2b. The concentration of ENAIs and HNAIs are maintained at 3 × 105 cm−3 approximately in a confined space (60 × 60 × 60 cm). We recorded the decay progress of two kinds of NAIs. ENAIs survived for only 42 s, while HNAIs lasted 62 s from 3 × 105 cm−3 to less than 100 cm−3. The lifespan of HNAIs is extended about 47.62% compared to ENAIs. These findings are consistent with the previous studies that HNAIs are more stable in air after generation and can even exist for several minutes, but ENAIs disappeared within few seconds.13 The lifetime of NAIs is positively related to the stability, and NAIs can survive longer if it can remain stable for long time.
Application of NAIs is always limited by the short lifetime and short distance of propagation. The transmission distance of NAIs is difficult to reach 2 m away unless the concentration of NAIs is enough for attenuation, but the harmful effects of the by-products far exceed the health influence of NAIs. According to the Standards of United Nations Health Organization, when the density of NAIs in air reaches 1500 cm−3, it can be defined as fresh air. The concentration of two kinds of NAIs at different detection distance of 0.5, 1.0, 1.5, and 2.3 m in a normal indoor environment were recorded as shown in Fig. 2c. Change in the concentration of ENAIs is more obvious than that of HNAIs. There was almost no ENAIs at 1.5 m; however, HNAIs could still maintain a concentration of about 2700 cm−3 even at 2.3 m, which means that the cluster ions effectively avoid charge loss during propagation. The most essential difference of the physical properties of ENAIs and HNAIs is different molecular weights and different humidity. It is very necessary to explore the influencing factors, which could affect the stability of negative air ions.
To verify the effect of molecular weight on the stability of NAIs, two essential oils were added into the water solution: one fat-soluble essential oil (FSEO) and one water-soluble essential oil (WSEO). The main components of these two essential oils were detected by gas chromatography-mass spectrometry (GCMS-QP2010, SHIMADZU, Japan), as shown in Fig. 3a. The molecular weight of siloxanes contained in FSEO is mainly between 300 and 450, which has a low solubility. Under the same conditions, the intensity of HNAIs prepared from the aqueous solution containing two essential oils was measured, as shown in Fig. 3b. Although the molecular weight of FSEO is heavier than WSEO, the total concentration of HNAIs generated by the FSEO solution is less than that generated by the WSEO solution. Few organic-siloxane molecules combined with the HNAIs and the Lenard effect mainly occurs between water and airflow. In contrast, the WSEO is mainly composed of some oxygen-containing organic substances, which can be dissolved in water. Organic molecules would be wrapped in cluster ions to increase the molecular weight when the HNAIs were generated. WSEO dissolved in the HNAIs results in the increase of surface tension of the ultrafine droplets. Therefore, it is difficult for the water molecules to volatilize from the surface of the droplets, resulting in more stable HNAIs.14
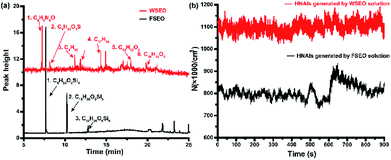 |
| Fig. 3 Effect of molecule weight on the stability and yield of HNAIs. (a) GC-MS spectrum of two essential oils; (b) concentration of HNAIs generated from two solutions. | |
Subsequently, the effect of air humidity on the stability of HNAIs was investigated due to the structural characteristics of hydrated-NAIs. Fig. 4 depicts the average intensity of HNAIs versus different relative humidity at 17.1 ± 1.1 centigrade. In a relatively dry environment (humidity ≤ 40%), the concentration of HNAIs is positively correlated with air humidity. The saturated vapour pressure of water molecules in a dry environment is low. The water molecules in HNAIs have a trend to volatilize into the surrounding environment, which reduces the molecular weight of HNAIs, resulting in its instability. At lower levels of relative humidity, a decrease in the concentration of water molecules shifts the equilibrium of the hydrated NAIs toward smaller cluster ions. With the increase in air humidity, free water molecules gradually increase, inhibiting the water molecules in HNAIs from diffusing into the air to keep them stable. When the air humidity reaches 45%, the concentration of HNAIs becomes relatively stable. A reasonable explanation for the relatively stable intensity of HNAIs is that the hydrated NAIs reached dynamic equilibrium with water molecules. The lifetime of O2−(H2O)n is about 1.1 × 104 times that of O2−, and this is why HNAIs can remain stable for a relatively long time.
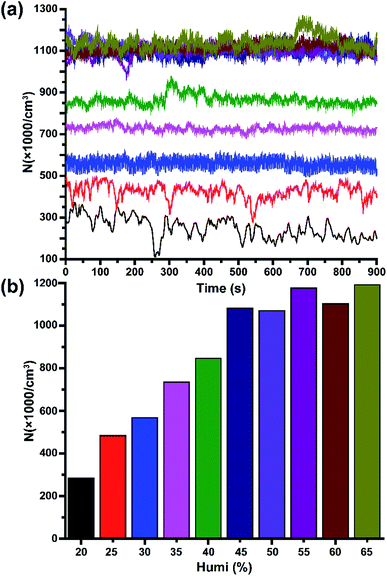 |
| Fig. 4 Effect of relative humidity on the concentration and stability of HNAIs. (a) Spectrum of HNAI concentration; (b) average HNAI intensity at different humidity. | |
The formation mechanism of the hydrated negative air ions as the terminal product is described in Fig. 5. TiO2 is usually used as a photocatalytic material in the field of photolysis of water, with high efficiency, low energy consumption, green and non-toxic, etc.15 Combining the Lenard effect and photocatalytic materials to prepare hydrated NAIs is a new attempt with the advantages of high stability and long life span. The HNAIs generated in this experiment consist mainly of two parts, part one is prepared by air–water interaction. Low pressure will be formed locally due to the driving effect of the airflow when the airflow passes through the water outlet at high-speed, thereby pressing the water flows outside. Before the water droplets and airflow hit the pedestal at high speed, active air molecules acquired electrons to generate intermediates such as superoxide radicals.16
|
O2− + n1H2O → O2−·(H2O)n1
| (2) |
|
OH− + nH2O → OH−(H2O)n
| (4) |
|
OH˙ + nH2O → OH·(H2O)n
| (5) |
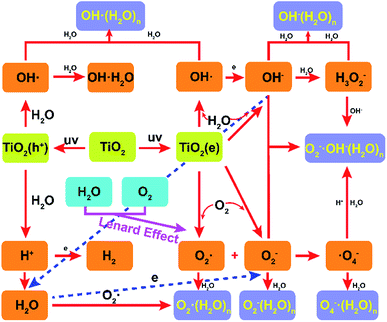 |
| Fig. 5 Formation mechanism of hydrated-NAIs by air–water two phase collision with the rosin-TiO2 photocatalytic material. | |
When moving droplets is sprayed on the hard pedestal, the droplets undergo charge redistribution forming ultrafine droplets with negatively charged surfaces. The charge separation occurs as a consequence of the disruption of the droplet surface by splashing and bubbling, and OH− is the dominant ion combining with the water molecules in the smaller fragments.17
However, bigger droplets with positive charge recirculate into smaller droplets. HNAIs are constantly generated according to physical–chemical reactions, such as:
|
OH− + n2H2O → OH−(H2O)n2
| (8) |
Due to the versatility of hydrogen bonds, water molecules formed a shell around OH−, increasing the abundance of HNAIs. Even in a complex environment, HNAIs are more stable than the free ions released by corona discharge or other radiated excitation. Similar to the waterfall effect, this part is a miniaturized simulation of the waterfall.18 An atomization phenomenon was obtained by increasing the air velocity, which has a relationship with the yield of HNAIs. In addition to contributing to increase in the concentration of HNAIs, water atomization can improve the relative humidity significantly as well.
The focus of the second part is the utilization of the free electrons generated by TiO2 to increase the yield of HNAIs. Since the first publication of TiO2 for water splitting by FUJISHIMA and HONDA, TiO2 has been widely used for H2 generation.19 When the visible light radiates on TiO2, the electrons in TiO2 are excited from the valence band (VB) to the conduction band (CB), while the electron holes remain in the VB, causing separation of electrons and holes, and then reduce water to hydrogen or oxidize water to oxygen at different locations of TiO2. In this experiment, 365 nm high-energy UV light was focused on the surface of fixed TiO2 to continuously produce photocatalytic electrons. Rosin was used as the fixing agent for TiO2 to improve the yield of electrons due to its excellent light transmission characteristic. Ultrafine droplets react on the surface of rosin-TiO2 pedestal as follows:20–22
|
TiO2 + UV → e− + TiO2(hole+)
| (9) |
|
TiO2(hole+) + H2O → ·OH + H+
| (10) |
|
e− + 2H2O → OH− + ·OH + 2H˙
| (12) |
|
OH− + n3H2O → OH−(H2O)n3
| (14) |
|
O2− + nH2O → O2−(H2O)n
| (15) |
Exciting TiO2 as an energy source can continuously provide free electrons to ensure the yield of HNAIs. To verify the reaction mechanism of water droplets on the TiO2 surface, we recorded the pH of the circulating large droplets, as shown in Fig. S2.† The results showed that the yield of HNAIs is closely related to the pH of the water solution. Three different initial pH water solutions (deionized water, pH = 6.05; adjust WaHaHa pH with acetic acid, pH = 4.91; Nongfu spring with NaOH, pH = 7.78) were used to prepare HNAIs. The pH of water is constantly changing due to the ionization reaction on the surface of TiO2. Corresponding to the low pH, the proton content in the water solution is high, which will consume the free electrons generated by TiO2 and reduce the relative yield of HNAIs. The pH of water solution is corresponds to the concentration of OH−, which will change the reaction equilibrium and increase the yield of HNAIs. As shown in Fig. S3,† the HNAI yield decreased obviously with the increase in pH (WHH water, pH value from 5.86 to 6.21), proving that the protons consume he photo-generated electrons and reduced the yield of HNAIs. It is better to prepare HNAIs with neutral water or weakly alkaline water, which helps to increase the actual yield of HNAIs. Under optimal condition, we obtained an average concentration of 1.2 × 106 cm−3 of HNAIs (detection distance is 0.8 m) with enough stability, which can meet indoor use.
In summary, a new strategy for preparing hydrated negative air ions by combining the Lenard effect and photocatalytic water electrolysis was proposed. TiO2 significantly increased the yield of HNAIs and effectively extended the lifetime of HNAIs by about 47.62% compared with NAIs prepared by corona discharge. The stability of HNAIs has a correlative relationship with the molecule weight and relative humidity. HNAIs with lower mobility has larger molecule weight and low probability to collide with other air particles so that it can remain relatively stable. Water molecules form a water shell around cluster ions in the high relative humidity environment, which protects the ions avoiding collision to extend the lifetime of NAIs. This method has the advantages of low cost, high yield, non-toxicity, and can be used to improve the home environment conveniently.
Conflicts of interest
There are no conflicts to declare.
Acknowledgements
This work was supported by National Natural Science Foundation of China (No. 21727814, 21775086, and 21621003).
Notes and references
- A. P. Krueger and R. F. Smith, J. Gen. Physiol., 1960, 44, 269–276 CrossRef CAS.
- A. P. Krueger and E. J. Reed, Science, 1976, 193, 1209–1213 CrossRef CAS.
- M. Kondrashove, E. V. Grigorenko, A. Tikhonov, T. V. Sirota, A. V. Temnov, I. G. Stavrovskaja, N. I. Kosyakova, N. V. Lange and V. P. Tikhonov, IEEE Trans. Plasma Sci., 2000, 28, 230–237 CrossRef.
- K. Nishikawa and H. Nojima, Jpn. J. Appl. Phys., Part 2, 2001, 40(8A), L835 CrossRef CAS.
- J. Ford, U.S. Pat. 6,464,754[P], 2002-10-15.
- J. M. Roux, R. Sarda-Estève, G. Delapierre, M. H. Nadal and L. Olmedo, Environ. Sci. Pollut. Res. Int., 2015, 23, 8175–8183 CrossRef.
- L. Fan, J. Song, P. Hildebrand and C. Forney, Eur. J. Appl. Microbiol., 2002, 93, 144–148 CrossRef CAS.
- A. Boumail, S. Salmieri and M. Lacroix, Postharvest Biol. Technol., 2016, 118, 134–140 CrossRef CAS.
- L. Ling, L. Yu, K. Mashooq, S. Jiashu and L. Jin-Ming, Chem. Commun., 2018, 54(76), 10687–10690 RSC.
- K. Cai, X. Liu, Y. Xu, C. Ren, H. Chen, J. Xu and Z. Yu, Sci. Total Environ., 2008, 401, 176–183 CrossRef CAS.
- R. Yamada, S. Yanoma, M. Akaike, A. Tsuburaya, Y. Sugimasa, S. Takemiya, H. Motohashi, Y. Rino, Y. Takanashi and T. Imada, Cancer Lett., 2006, 239, 0–197 CrossRef CAS.
- A. Sakoda, K. Hanamoto, N. Haruki, T. Nagamatsu and K. Yamaoka, Appl. Radiat. Isot., 2009, 65, 50–56 CrossRef.
- K. Nagato, Y. Matsui, T. Miyata and T. Yamauchi, Int. J. Mass Spectrom., 2007, 248, 142–147 CrossRef.
- C. C. Wu, G. W. M. Lee, S. Yang, K.-P. Yu and C. L. Lou, Sci. Total Environ., 2004, 370, 245–253 CrossRef.
- K. Nagato, Y. Matsui and T. Miyata, Int. J. Mass Spectrom., 2006, 248(3), 142–147 CrossRef CAS.
- M. Hayyan, M. A. Hashim and I. M. Alnashef, Chem. Rev., 2017, 116, 3029–3085 CrossRef.
- P. Kolarz, M. Gaisberger, P. Madl, W. Hofmann, M. Ritter and A. Hartl, Atmos. Chem. Phys., 2012, 12(8), 3687 CrossRef CAS.
- A. Luts, T.-E. Parts, L. Laakso, A. Hirsikko, T. Grnholm and M. Kulmala, Atmos. Res., 2009, 91, 237 CrossRef.
- A. Fujishima and K. Honda, Nature, 1972, 238(5358), 37–38 CrossRef CAS.
- J. P. Kehrer, T. E. Tipple, J. D. Robertson and C. V. Smith, Free Radical Biol. Med., 2010, 32, 982–990 Search PubMed.
- C. M. Jones, A. Lawrence, P. Wardman and M. J. Burkitt, Free Radical Biol. Med., 2002, 32, 982–990 CrossRef CAS.
- R. F. Mathist and W. R. Snow, J. Chem. Phys., 1974, 61, 4274–4278 CrossRef.
Footnote |
† Electronic supplementary information (ESI) available. See DOI: 10.1039/d0ra08693b |
|
This journal is © The Royal Society of Chemistry 2020 |
Click here to see how this site uses Cookies. View our privacy policy here.