DOI:
10.1039/D0RA08852H
(Paper)
RSC Adv., 2020,
10, 44551-44570
Heterogeneous activation of persulfate by ZnCoxFe2−xO4 loaded on rice hull carbon for degrading bisphenol A
Received
17th October 2020
, Accepted 25th November 2020
First published on 17th December 2020
Abstract
A new low-cost composite of ZnCoxFe2−xO4 loaded on rice hull carbon (ZnCoxFe2−xO4-RHC) was synthesized via waste ferrous sulfate (the industrial waste produced in the process of producing titanium dioxide) and rice hull as raw materials, which was applied for the degradation of bisphenol A (BPA) by heterogeneous activated peroxodisulfate (PS). A series of characterizations including XRD, SEM, FTIR, and BET analysis were carried out to analyze the structure and morphology of the materials. It is confirmed that the ZnCoxFe2−xO4-RHC composites show better catalytic activity and performance than other control samples, which can be attributed to the synergistic effect of Fe and Co, ZnCoxFe2−xO4 and RHC based on these analyses. The degradation rate of BPA by ZnCo1.3Fe0.7O4-50%RHC reached 100% within 15 min, and it can still maintain good catalytic efficiency after 5 cycles. ESR test and XPS results showed that free radical and non-free radical processes were involved in BPA degradation. These findings offer a novel, low cost and simple strategy for rational design and modulation of catalysts for the industrial degradation of organic pollutants, and provide a new idea for the utilization of waste ferrous sulphate in titanium dioxide industry.
1 Introduction
Bisphenol A (BPA), also known as diphenolic propane, is an important raw material used in the manufacture of polycarbonate plastics and epoxy resins, which widely exists in industrial wastewater.1 As a typical endocrine disruptor, BPA can mimic and interfere with the secretion of endogenous hormones, hinder the hormone's function, interfere with the hormone balance in the human body, and thus affect the endocrine, nerve, immune and reproductive systems of humans and animals, causing great harm to human health.2,3 In addition, BPA is not biodegradable and highly resistant to chemical degradation.4 Therefore, it is crucial to develop a cost-effective, low-consumption, green treatment technology to remove BPA in waste water.
In recent years, among the multiple technologies for removing BPA, the emerging persulfate oxidation technology based on sulfate radicals (SO4˙−) has attracted more and more attention.5 As a kind of strong oxidant, the sulfate radical degrades organic pollutants in the system remarkably. Compared with the traditional advanced oxidation method based on hydroxyl radical (˙OH), the use of SO4˙− has a number of advantages, such as higher oxidation potential (SO4˙−, Eθ = 2.5–3.1 V; ˙OH, Eθ = 1.8–2.7 V),6 higher selectivity and efficiency for organic pollutants with unsaturated bonds or aromatic rings, wider pH range (pH = 2–8), longer half-life.7 Persulfate oxidation technology can activate K2S2O8 (PS) through heat, ultraviolet light and transition metals (such as Fe2+, Cu2+, Co2+, Ag+) to form sulfate radicals (SO4˙−).8–10 The use of transition metal ions requires lower activation energy than other external energy. However, it is difficult for transitional ions to recover and may cause secondary pollution. Therefore, it is of great significance to choose an activating agent for PS that can improve the recycling rate and can be magnetically separated.
Spinel ferrite [AB2O4 (A = Mn2+, Co2+, Ni2+, Zn2+, etc. B = Fe3+)] is a class of important functional materials because of their unique electromagnetic, optical and catalytic properties, stable chemical properties, and excellent magnetic properties. Spinel zinc ferrite (ZnFe2O4) has attracted wide attention due to its low cost, free of precious or carcinogenic metals, and environmentally friendly.11 The synthesis methods of ZnFe2O4 mainly include solid phase method,12 co-precipitation method,13 hydrothermal method,14 sol–gel method15 and so on. Among them, the solid phase process is a simple and effective method because of operation to easy, environmentally friendly, low cost, short time, and suitable for industrialization. The current reported studies for the synthesis of ZnFe2O4 by solid phase method usually take analytically or chemically pure ferrous sulfate as raw material.16–18 Due to the process of producing titanium dioxide by sulfuric acid method, about 7 million tons of ferrous sulfate waste was produced in 2018, and it increases at a rate of more than 10% with the increasing demand for titanium dioxide year by year.19–21 Nowadays most of waste ferrous sulphate is still disposed as solid waste.22 Such vast amount of piled waste ferrous sulphate not only occupies the farm land but also causes serious pollution to the surrounding environment, and causes a great waste of renewable resources. This left us open to thinking that whether the waste ferrous sulphate could be adopted as raw materials to obtain ZnFe2O4 via facile solid state method, thus to realize the resource utilization of waste, and further reduce the cost of preparing ZnFe2O4. It is worth noting that the ZnFe2O4 nanoparticles prepared by the solid phase method are peculiarly prone to aggregate, which result in reduced active sites and unfavourable catalytic property.23 On the one hand, this can be improved by Co doping because of the excellent catalytic activity of Co. Zhong et al.24 confirmed that Co-doped bismuth ferrite increased the degradation rate of levofloxacin by 3.52 times compared with undoped BFO. Zhang et al.25 found that Co doping ZnFe2O4 have abundant oxygen vacancy, which can rapidly activate persulfate to remove organic pollutants. In addition, due to the synergistic effect of Zn and Co, the metal ion leaching rate of Co-doped zinc ferrite nanomaterials is reduced.26 On the other hand, the composite of ferrite nanoparticles and carbon matrix (graphene, carbon nanotubes, et al.) can deliver enhanced catalytic activity compared with ferrite nanoparticles, profiting from the inhibition of agglomeration by carbon matrix. It is important to choose a cheap carbon matrix from a practical industrial applications view point. For the past few years, rice hull carbon, which is a common food by-product, has been widely studied as biomass carbon source applied in many fields owing to the source cheap and sustainable.27 The derived rice hull carbon (RHC) has the feature of large specific surface area, strong adsorption capacity, and fast conduction rate, which can raise synergistic effect between ferrite and RHC, leading to higher catalytic activity of the ferrite/RHC composite.28
In this study, we designed and prepared a new composite of cobalt doped zinc ferrite loaded on rice hull carbon (ZnCoxFe2−xO4-RHC), via one-step solid phase method using waste by-product ferrous sulfate as iron source and rice hull as carbon source. The composite shows enhanced activity and property when used as a catalyst for bisphenol A degradation in persulfate system. The synthetic and degradation mechanisms and degrading BPA process were studied, the degradation conditions were optimized, and the reaction kinetics and cyclic stability of the composite were investigated systematically. This research put forward a thinking of “using waste to treat waste”, which not only provides an efficient and environmentally friendly way to degrade BPA, but also expands the resource utilization of waste by-product ferrous sulfate, and thus achieve good economic and environmental benefits.
2 Experimental section
2.1. Materials
Waste by-product ferrous sulphate from Panzhihua Iron and Steel Group Co. Ltd (Sichuan, China). Rice husks are taken from Sichuan agricultural production base (Sichuan, China). Bisphenol A(C15H16O2) was purchased from Aladdin Reagent Co. Ltd. Pyrite (FeS2) purchased from Beijing Hawke Technology Co. Ltd. Cobalt sulfate (CoSO4·7H2O), zinc sulfate(ZnSO4·7H2O), potassium peroxosulfate (K2S2O8), sodium hydroxide (NaOH), hydrochloric acid (HCl), ethanol (C2H6O, EtOH) were purchased from Chengdu Kelong Chemical Reagents Co. Ltd. tert-Butanol (C4H10O, TBA), 5,5-dimethyl-1-pyrroline-N-oxide (DMPO), 2,2,6,6-tetramethyl (TEMP), p-benzoquinone (BQ), potassium chloride (KCl), potassium nitrate (KNO3), potassium carbonate (KHCO3), potassium dihydrogen phosphate (KH2PO4) were purchased from Chengdu Jinshan Chemical Reagent Co. Ltd. Furfuryl alcohol (C5H6O2, FFA) was purchased from Makerlin Biochemical Co. Ltd. All the solutions were prepared using deionized water.
2.2. Preparation of catalyst
Rice husk is washed with deionized water for several times and put them in a dry place to dry naturally. The dried rice husks are put into a ball mill and ground for 1 h at a rotational speed of 500 rpm. Then, rice husk powder is put into a tubular furnace and calcines at 600 °C for 120 min under the protection of N2 atmosphere to obtain RHC. The cooled RHC is added to 3 M NaOH and stirrs continuously for 12 h to remove the silicon dioxide in the rice hull. The rice hull after desilication is washed many times in deionized water until it becomes neutral. Then, it is added to a solution of dilute hydrochloric acid and stirs continuously for 3 h to remove the metal oxides. Washing with deionized water several times until neutral, and then dry at 50 °C for 3 h. Store in a dryer for later use.
Using pyrite as the reducing agent and waste ferrous sulfate as the iron source, the ZnSO4·7H2O, the waste ferrous sulfate and pyrite are weighed by a certain molar ratio, and dried at 100 °C for 5 h. Then, Co molar ratios (x = 0, 0.7, 1.3, 1.6 and 2) (Table 1) and RHC mass ratios (y = 0, 20, 40, 50 and 70 wt%) (Table 2) were add into the mixture, and ball milled in a ball mill of 500 rpm for 2 hour. The mixture after homogenization was put into a tube furnace and calcined at 550 °C for 90 min under a protective atmosphere to synthesize ZnCoxFe2−xO4-y wt% RHC composite. Following cooling to room temperature, wash with deionized water and absolute ethanol 5 times to remove impurities. Finally, it was dried in a freeze dryer for 24 hours.
Table 1 Abbreviation for sample corresponding to the cobalt molar ratios in the course of synthesis (Zn/Co/Fe)
Co molar ratios (x) |
x = 0 |
x = 0.7 |
x = 1.3 |
x = 1.6 |
x = 2 |
ZnCoxFe2−xO4 |
ZnFe2O4 |
ZnCo0.7Fe1.3O4 |
ZnCo1.3Fe0.7O4 |
ZnCo1.6Fe0.4O4 |
ZnCo2O4 |
Table 2 Abbreviation for sample corresponding to the RHC mass ratios
RHC mass ratios (y wt%) |
y = 0 |
y = 20 |
y = 40 |
y = 50 |
y = 70 |
ZnCo1.3Fe0.7O4-y%RHC |
ZnCo1.3Fe0.7O4 |
ZnCo1.3Fe0.7O4-20%RHC |
ZnCo1.3Fe0.7O4-40%RHC |
ZnCo1.3Fe0.7O4-50%RHC |
ZnCo1.3Fe0.7O4-70%RHC |
2.3. Characterization of catalyst
Phase analysis of the catalyst is carried out using an X-ray diffractometer (XRD, Empyrean, PANalytical) at CuK ray (λ = 0.15401 nm), working current 300 mA, working voltage 40 kV, scanning speed 0.02° s−1, scanning range 5–80°. Model JSM-5900LV SEM-EDX is used to analyze the surface morphology and element composition of the samples. Model XSAM800 XPS (Kratos, UK) is used to study the surface composition of the sample. The morphology of the samples is observed by JEOL JEM-12100 transmission electron microscope (TEM). The surface properties of the samples are identified by Andor SR-500I Raman spectrum (RM) and Nicolet IS10 Fourier Transform spectrum (FTIR). The reaction mechanism of the mixed materials and the thermal stability of the samples are analyzed by TG-DSC (STA 449F3, Germany). N2 adsorption and desorption data are obtained by Mike 2460 Brunauer–Emmet–Teller technique (BET). DMPO and TEMP are used as trapping agents to analyze the free radicals generated by the reaction using electron paramagnetic resonance (ESR, JEOL JES-FA200). The Zn, Fe and Co ions released from the catalyst are detected by a plasma spectrometer (ICP-OES, Shimadzu, Japan).
2.4. Degradation experiments
The degradation process of BPA is conducted in a beaker followed by stirring at room temperature. At the same time, all experiments are carried out by adding 200 mL of BPA solution (20 mg L−1), K2S2O8 (molar ratio of K2S2O8/biphenol A is 42.23), ZnCoxFe2−xO4-y wt% RHC catalyst (0.75 g L−1) to a 250 mL beaker, and the mixing paddle stirs the mixture at 400 rpm to start the catalytic degradation reaction. At each predetermined time interval (0, 3, 5, 10, 15, 20, and 30 minutes), 10 mL of the supernatant is taken out and Na2S2O3 solution (0.5 M) is added into to the supernatant to prevent further BPA degradation. After that, the solution is centrifuged at high speed for 5 min in a centrifuge, and takes the supernatant concentration to be measured. By adding NaOH (0.1 M) and H2SO4 (0.1 M) to adjust pH to 2, 5, 7, 9 and 11, the effect of pH value on degradation experiment is tested. Cl−, H2PO4−, HCO3−, NO3− are added to the solution to explore the effect of anions on catalytic oxidation of BPA. tert-Butyl alcohol (TBA), ethanol (EtOH), p-benzoquinone (BQ) and furfuryl alcohol (FFA) are used as scavengers for SO4˙−, ˙OH, 1O2 and O2˙−. The molar ratio of TBA to PS, EtOH to PS, BQ to PS and FFA to PS are set as 1000, 1000, 600, and 600 respectively to study the free radicals in the catalytic systems. BPA concentrations are measured at 277 nm with an ultraviolet spectrophotometer (V-5800).
3 Results and discussions
3.1. Synthesis and characterization of Zn,Co,Fe-RHC composites
The TG-DSC was used to analyze the formation mechanism of ZnCoFeO4 synthesized by solid phase reduction. As shown in Fig. 1, under the protection of nitrogen, the thermal decomposition of raw materials is divided into three processes. The first stage is at the temperature range between 71 and 95 °C, with a mass loss of 1.29% due to the loss of adsorbed water on the surface of raw materials. In the second stage of 267–375 °C, the mass loss is 9.26%, which is due to the loss of crystal water in waste by-product, the loss of crystal water in zinc sulfate and cobalt sulfate. The third stage is from 481 °C to 862 °C, with a mass loss of 39.89% due to the reaction of the feedstocks with each other and the release of sulfur dioxide gas. It can be inferred that ZnCoFeO4 is synthesized through the following reactions (eqn (1)–(4)): |
ZnSO4·7H2O → ZnSO4 + 7H2O(g)
| (1) |
|
FeSO4·H2O → FeSO4 + H2O(g)
| (2) |
|
CoSO4·7H2O → CoSO4 + 7H2O(g)
| (3) |
|
2FeSO4 + 3CoSO4 + 3ZnSO4 + FeS2 → 3ZnCoFeO4 + 10SO2
| (4) |
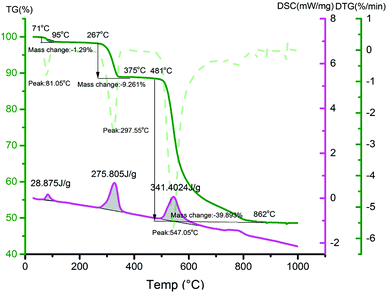 |
| Fig. 1 TG-DSC of the obtained mixtures by mixing zinc sulfate, cobalt sulfate, waste ferrous sulfate and pyrite in a molar ratio of 4 : 4 : 3.5 : 1. | |
Fig. 2a shows the XRD patterns of the waste ferrous sulfate, ZnCo1.3Fe0.7O4, ZnCo1.3Fe0.7O4-20%RHC, ZnCo1.3Fe0.7O4-50%RHC and RHC. It can be seen that the XRD pattern of the waste ferrous sulfate is basically consistent with the standard pattern of FeSO4·H2O (JCPDS Card No. 81-0019), indicating that the main component of the purified ferrous sulfate is FeSO4·H2O. From the XRD patterns of ZnCo1.3Fe0.7O4, ZnCo1.3Fe0.7O4-20%RHC and ZnCo1.3Fe0.7O4-50%RHC, it can be observed that the 2θ values of the diffraction peaks are 18.16°, 30.05°, 35.43°, 36.94°, 42.97°, 56.91° and 62.43°, corresponding to the crystal planes (111), (220), (311), (222), (400), (511) and (440) respectively for the as-obtained samples, which are in agreement well with the standard characteristic peaks for the cubic spinel ferrites with the conventional formula [Fe3+]A[M2+Fe3+]BO4 as a reference standard for ZnFe2O4 (JCPDS Card No. 77-0011) and CoFe2O4 (JCPDS Card No. 22-1086).29 RHC has a broad peak at 25.23°, which is indicative of amorphous carbon. The (002) crystal plane appeared in the XRD pattern of ZnCo1.3Fe0.7O4-50%RHC (JCPDS Card No. 41-1487), indicating that rice husk carbon was successfully doped into ZnCo1.3Fe0.7O4.30 The (002) crystal plane did not appear in the XRD pattern of ZnCo1.3Fe0.7O4-20%RHC because the doped biochar content is less, and the intensity of the diffraction peak is weak, which is covered by the strong peak of ZnCo1.3Fe0.7O4. These XRD results indicate that ZnCo1.3Fe0.7O4 nanoparticles were successfully interspersed on the rice husk carbon substrate.
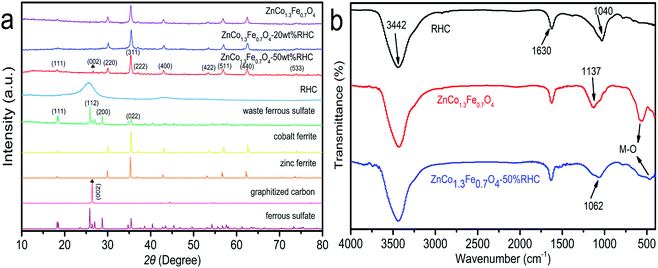 |
| Fig. 2 The XRD patterns (a), FTIR spectra (b) of samples. | |
The FTIR spectra of RHC, ZnCo1.3Fe0.7O4 and ZnCo1.3Fe0.7O4-50%RHC are shown in Fig. 2b. The absorption peaks of 1040, 1630 and 3442 cm−1 in the RHC spectrum can be attributed to C–O–C stretching vibration, C
O stretching vibration and O–H stretching vibration in water molecules, respectively.31 Comparing with the spectra of RHC and ZnCo1.3Fe0.7O4-50%RHC. We can observe the peaks corresponding to oxygen-containing functional groups in RHC with a slight shift for some of them, which indicates that RHC has been supported well on the ZnCo1.3Fe0.7O4 in ZnCo1.3Fe0.7O4-50%RHC. Besides, the absorption peak of 571 cm−1 observed in the spectrum of ZnCo1.3Fe0.7O4 can be attributed to the stretching vibration of Zn2+–O2− while the absorption peak of 413 cm−1 can be attributed to the stretching vibration of M3+–O2− (Co3+–O2−, Fe3+–O2−) in typical spinel ferrite.32 In the FTIR spectrum of ZnCo1.3Fe0.7O4-50%RHC, the peak value of metallic bond (M–O) corresponding to ZnCo1.3Fe0.7O4 varies. This means that ZnCo1.3Fe0.7O4-50%RHC is not a simple mixture of ZnCo1.3Fe0.7O4 and RHC.
Fig. 3a–c are SEM images of RHC, ZnCo1.3Fe0.7O4 and ZnCo1.3Fe0.7O4-50%RHC, respectively. It can be seen from Fig. 3a that the RHC has a smooth surface and a huge sheet structure. The ZnCo1.3Fe0.7O4 shows nano-structure with uniform spherical particles, and it occurs agglomeration of nanoparticles caused by electrostatic attraction and van der Waals force. From Fig. 3c, the ZnCo1.3Fe0.7O4 nanoparticles are interspersed in the RHC, which alleviates the agglomeration phenomenon to a certain extent. The EDX spectrum result shown in Fig. 3d indicates that the prepared sample is composed of Fe, Zn, O, Co and C. The element map confirms that the distribution of Zn, Co, Fe, O and C is relatively uniform, which is beneficial to enhance the catalytic activity.
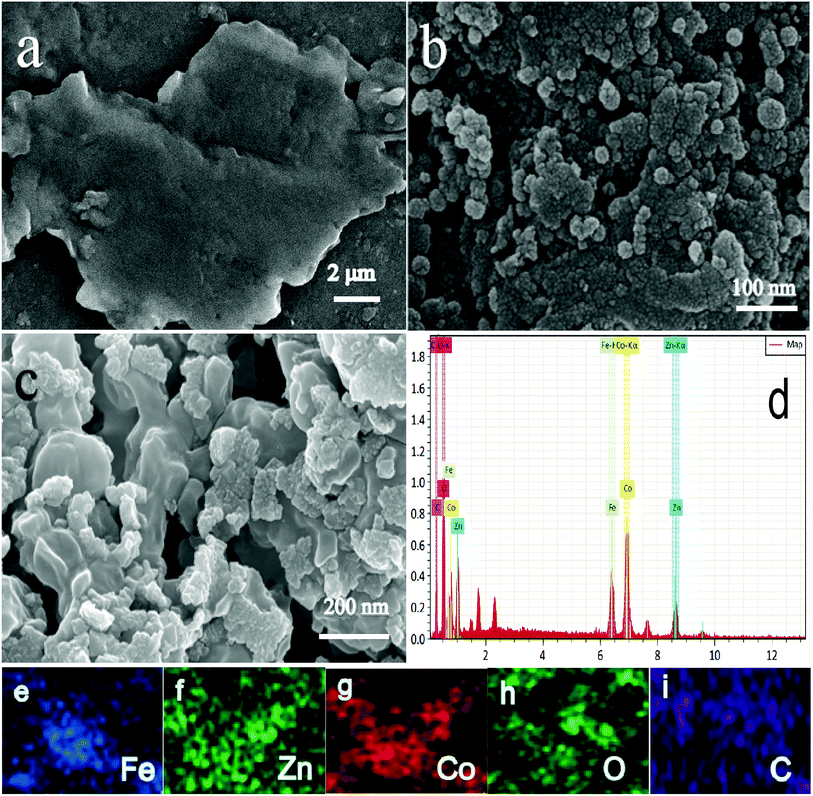 |
| Fig. 3 SEM images of RHC (a), ZnCo1.3Fe0.7O4 (b), ZnCo1.3Fe0.7O4-50%RHC (c). Energy dispersive X-ray spectroscopy (EDS) patterns of ZnCo1.3Fe0.7O4-50%RHC (d). EDS elemental mapping of Fe (e), Zn (f), Co (g), O (h) and C (i). | |
Fig. 4a and b display the TEM micrographs of ZnCo1.3Fe0.7O4-50%RHC and ZnCo1.3Fe0.7O4 composites. ZnCo1.3Fe0.7O4 shows serious aggregation state in Fig. 4a, which is consistent with SEM results. In addition, the ZnCo1.3Fe0.7O4 nanoparticles (Fig. 4b) are embedded in the biological carbon layer, indicating that the RHC acts as a carrier material and disperses ZnCo1.3Fe0.7O4 nanoparticles. It can be seen from Fig. 4c that the spacing of (311) crystal planes corresponding to ZnCo1.3Fe0.7O4 is 0.251 nm.33 What's more, the lattice fringe of 0.311 nm near ZnCo1.3Fe0.7O4 (311) crystal plane is attributed to the lattice surface of carbon (002).34
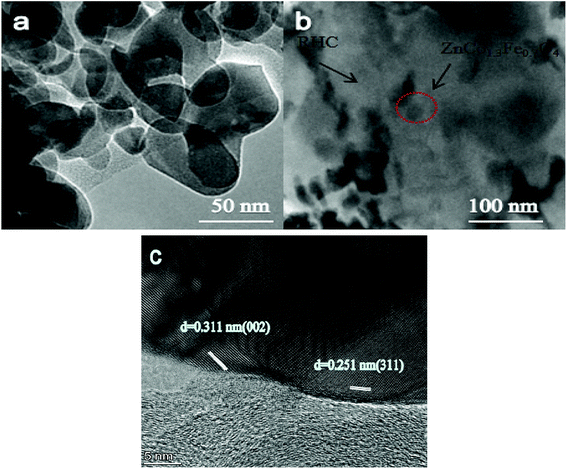 |
| Fig. 4 TEM images of ZnCo1.3Fe0.7O (a), ZnCo1.3Fe0.7O4-50%RHC (b). HRTEM images of ZnCo1.3Fe0.7O4-50%RHC (c). | |
The N2 adsorption–desorption isotherm is exhibited in Fig. 5. The total specific surface area and total pore volume of RHC are 133 m2 g−1 and 0.16 cm3 g−1. The total specific surface area of ZnCo1.3Fe0.7O4 is 10 m2 g−1, and the total pore volume is 0.011 cm3 g−1. The total specific surface area of ZnCo1.3Fe0.7O4-50%RHC is 37 m2 g−1, and the total pore volume is 0.08 cm3 g−1. The specific surface area of ZnCo1.3Fe0.7O4-50%RHC is 3.7 times larger than that of ZnCo1.3Fe0.7O4. It shows that the presence of RHC increases the specific surface area of the prepared nano-material and inhibits the aggregation of nanoparticles. Compared with RHC, the specific surface area of ZnCo1.3Fe0.7O4-50%RHC is reduced because ZnCo1.3Fe0.7O4 nanoparticles occupy the adsorption sites of activated biochar. The N2 adsorption–desorption isotherms of ZnCo1.3Fe0.7O4, ZnCo1.3Fe0.7O4-50%RHC and RHC show typical type IV isotherms, confirming that they are mesoporous materials.
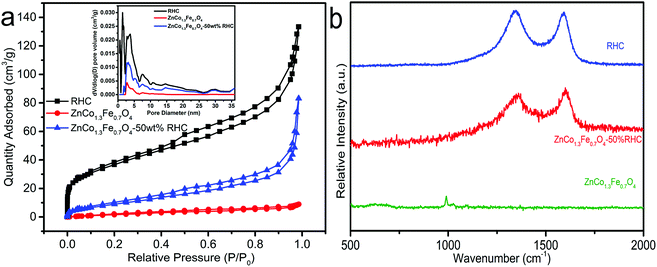 |
| Fig. 5 N2 adsorption–desorption isotherms (a), Raman spectra (b). | |
It can be seen from the Raman spectrum that RHC and ZnCo1.3Fe0.7O4-50%RHC have two characteristic peaks at near 1349 cm−1 and 1585 cm−1. Among them, 1585 cm−1 corresponds to the graphite structure (sp2), and the other peak at 1349 cm−1 corresponds to amorphous and polyphase carbon materials.35 The ID/IG of RHC and ZnCo1.3Fe0.7O4-50%RHC are 1.03 and 0.93, respectively. Compared with RHC, the ID/IG of ZnCo1.3Fe0.7O4-50%RHC is significantly reduced. That may be because at high temperatures, the catalytic action of Fe and Co causes the unstable carbon atoms to transform from the chaotic layer structure to the graphite crystal structure.
The surface chemical compositions of ZnCo1.3Fe0.7O4-50%RHC complex were determined by XPS. From the wide-scan XPS spectrum of ZnCo1.3Fe0.7O4-50%RHC, the binding energies of 285, 533, 712, 781 and 1023 eV, demonstrate the coexistence of C, O, Fe, Co and Zn in the sample. The C 1s spectrum of ZnCo1.3Fe0.7O4-50%RHC (Fig. 6b) shows three convolution peaks of 284.7, 285.6 and 287.0 eV, corresponding to C–OH (60.2%), C–O–C (22.2%) and C
O (17.6%).36 The Fe 2p spectrum is depicted in Fig. 6c. The two main peaks with binding energies of 711.0 eV and 724.5 eV appear on the energy levels of Fe 2p3/2 and Fe 2p1/2, which are a typical feature of the ferrite Fe3+ respectively.37 In addition, the Co 2p spectrum (Fig. 6d) at 780.4 and 796.4 eV prove the presence of Co3+ in ZnCo1.3Fe0.7O4-50%RHC.38 In the activation process of PS, Co has higher catalytic activity than Fe. Therefore, it is possible that the substitution of Co3+ for Fe3+ ions in the spinel ZnCoxFe2−xO4-50%RHC lattice can improve its catalytic performance.
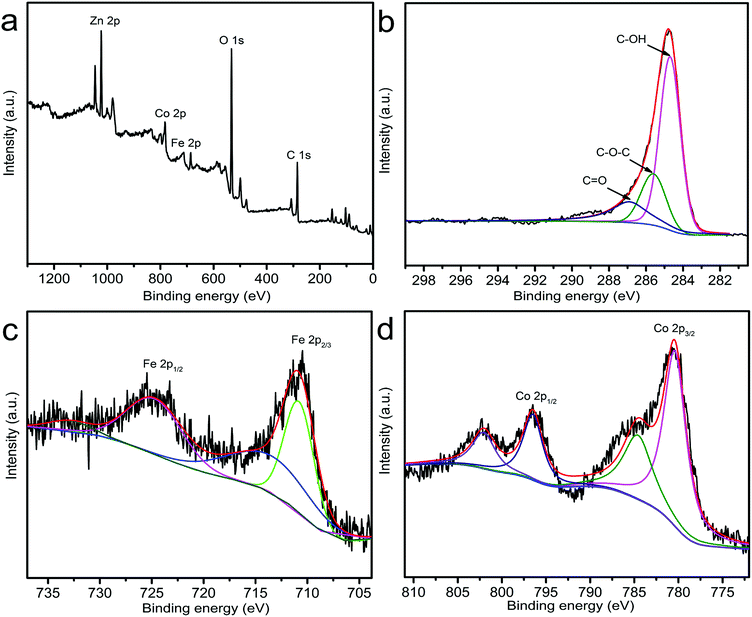 |
| Fig. 6 XPS spectrum of wide-scan (a), C 1s (b), Fe 2p (c) and Co 2p (d) of ZnCo1.3Fe0.7O4-50%RHC composites. | |
3.2. Catalytic properties of systems based on ZnCo1.3Fe0.7O4
Fig. 7a shows the effect of RHC, ZnCo1.3Fe0.7O4 and ZnCo1.3Fe0.7O4-RHC on BPA degradation with and without PS. The degradation rate of BPA by PS within 60 min is 6.0% without any catalyst, indicating that it is difficult to degrade BPA when PS exists only. In addition, RHC, ZnCo1.3Fe0.7O4 and ZnCo1.3Fe0.7O4-RHC degrade 28.4%, 12.6% and 22.3% of BPA within 60 min without PS addition, respectively, which is attributed to the adsorption of catalyst on BPA. However, after adding PS to ZnCo1.3Fe0.7O4 and ZnCo1.3Fe0.7O4-RHC, the degradation efficiency of BPA is significantly accelerated and the degradation rate of ZnCo1.3Fe0.7O4-RHC/PS reaches 96.3% within 10 min. It is more effective than RHC/PS (21.2%) and ZnCo1.3Fe0.7O4/PS (58.9%) systems in BPA degradation, showing that ZnCo1.3Fe0.7O4-RHC can activate PS more effectively than RHC and ZnCo1.3Fe0.7O4 for degrading BPA. The adsorption of ZnCo1.3Fe0.7O4-RHC on BPA degradation is not an important factor.
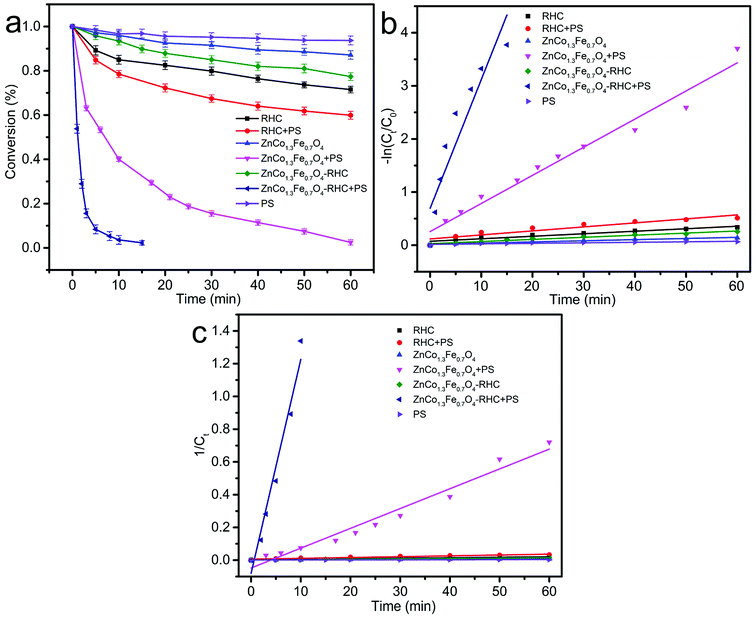 |
| Fig. 7 BPA degradation in different systems (a). Pseudo-first order kinetics model in different systems (b). Pseudo-second order kinetics model in different systems (c). Reaction conditions: [catalyst] = 0.75 g L−1; [PS] = 1.00 g L−1; [BPA] = 20 mg L−1, initial pH = 7, T = 25 °C. | |
In addition, the ZnCo1.3Fe0.7O4 has synergistic effect with RHC when BPA is degraded by ZnCo1.3Fe0.7O4-RHC system. This is because the degradation efficiency of ZnCo1.3Fe0.7O4-RHC system on BPA (Fig. 7a) is greater than that of RHC/PS and ZnCo1.3Fe0.7O4/PS system on BPA at 10 min, which confirms that RHC plays an important role in improving the catalytic activity of ZnCo1.3Fe0.7O4-RHC composite material. Synergistic effect is shown in: (1) RHC has can prevent the aggregation of part of ZnCo1.3Fe0.7O4 nanoparticles and a large specific surface area during the reaction. (2) RHC and ferrite materials accelerate the electron transfer rate, thus improving the catalytic performance of ZnCo1.3Fe0.7O4 nanoparticles.
The relationship between reaction rate and reaction time of different systems is analyzed by pseudo-first-order and second-order kinetics models. The pseudo-first-order kinetics model (eqn (5)) and second-order kinetics model (eqn (6)) are expressed as follows:39
|
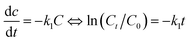 | (5) |
|
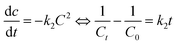 | (6) |
where
C0 is the initial concentration of BPA,
Ct is the concentration of BPA at time
t,
k1 is a pseudo-first-order kinetic degradation rate constant, and
k2 is a second-order kinetic degradation rate constant. The experimental data of BPA degradation are fitted, and the results are shown in
Table 3. As shown in
Fig. 7b and c, the correlation coefficient of the experimental data fitted with the second-order kinetic model (
R22 = 0.9104–0.9659) is higher than that of the pseudo-first-order kinetic model (
R12 = 0.8550–0.9617), so the degradation process of BPA should be more in line with the pseudo-second-order kinetic equation. Meanwhile, it can be observed that ZnCo
1.3Fe
0.7O
4-RHC has higher catalytic activity than ZnCo
1.3Fe
0.7O
4 and RHC to degrade BPA in the persulfate system.
Table 3 Kinetic parameters on BPA degradation of different catalysts
|
Pseudo-first-order kinetics |
Pseudo-second-order kinetics |
k1 (10−2 min−1) |
R12 |
k2 (10−2 L mg−1 min−1) |
R22 |
RHC |
0.47 |
0.8786 |
0.028 |
0.9127 |
ZnCo1.3Fe0.7O4 |
0.22 |
0.9442 |
0.012 |
0.9526 |
ZnCo1.3Fe0.7O4-RHC |
0.40 |
0.9527 |
0.023 |
0.9659 |
RHC + PS |
0.75 |
0.8594 |
0.051 |
0.9104 |
ZnCo1.3Fe0.7O4 + PS |
5.29 |
0.9617 |
1.21 |
0.9651 |
ZnCo1.3Fe0.7O4-RHC + PS |
25.25 |
0.8550 |
13.07 |
0.9599 |
PS |
0.097 |
0.9126 |
0.005 |
0.9497 |
3.3. Catalytic properties of Zn,Co,Fe-RHC composites
3.3.1 Effect of the molar ratio of Co to Fe in ZnCoxFe2−xO4 on the catalytic degradation of BPA. In order to comprehensively evaluate the catalytic performance of ZnCo1.3Fe0.7O4 for BPA degradation, as shown in Fig. 8a, we conducts a series of parallel experiments with ZnO, Fe3O4, Fe2O3, Co3O4 and ZnCo1.3Fe0.7O4 as catalysts. Within 30 minutes, the degradation efficiencies of ZnO, Fe3O4, Fe2O3, Co3O4 and ZnCo1.3Fe0.7O4 to BPA are 19.9%, 29.9%, 35.5%, 56.5% and 82.6%, respectively. Among them, ZnCo1.3Fe0.7O4 has the highest degradation rate of BPA, which shows that the coexistence of Co and Fe improves the catalytic activity of the catalyst. This indicates that in the reaction system, Co and Fe may have a synergistic effect, so that ZnCo1.3Fe0.7O4 has good catalytic activity.
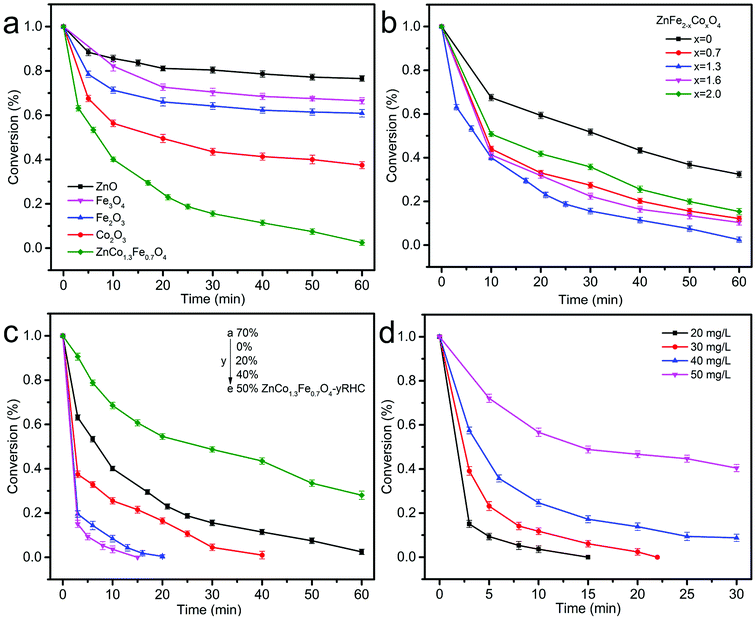 |
| Fig. 8 Degradation of BPA using different catalyst (ZnO, Fe3O4, Fe2O3, Co2O3 and ZnCo1.3Fe0.7O4, respectively) (a). Effect of the molar ratio of Co to Fe in ZnCoxFe2−xO4 (x = 0, 0.7, 1.3, 1.6 and 2, respectively) on the catalytic degradation of BPA using PS as oxidant (b). Effect of RHC content in ZnCo1.3Fe0.7O4-y%RHC (y = 70, 0, 20, 40 and 50, from a to e, respectively) on catalytic oxidation of BPA (c). Effect of bisphenol A concentration on catalytic degradation (d). ([catalyst] = 0.75 g L−1, [BPA] = 20 mg L−1, [PS] = 1.00 g L−1, initial pH = 7, T = 25 °C). | |
In addition, Fig. 8b shows the effect of Co content on BPA degradation. With the increase of Co content, the catalytic activity of ZnFe2−xCoxO4 for BPA first increased and then decreased. When x = 0, 0.7 and 1.3, the degradation efficiency of ZnFe2−xCoxO4 to BPA are 67.7%, 87.9% and 97.4% within 60 min, respectively. The results show that a proper proportion of Co doping can promote the activity of ZnFe2−xCoxO4. However, in the range of 1.3–2, the increase in the molar ratio of Co is inversely proportional to the degradation efficiency. This may be due to the introduction of a large amount of Co in the zinc ferrite to cause the formation of hematite, which has poor catalytic activity for PS.40,41 Therefore, the Co molar ratio x = 1.3 is an appropriate ratio of ZnFe2−xCoxO4.
3.3.2 Effect of RHC content on the catalytic degradation of BPA. Fig. 8c shows the effect of RHC content on the catalytic degradation of BPA. When RHC content is 0%, 20%, 40% and 50% in ZnCo1.3Fe0.7O4 nanoparticles, the degradation rate of BPA reaches 61.9%, 74.5%, 96.3% and 91.5% within 10 min. It shows that the introduction of RHC increases the surface area of ZnCo1.3Fe0.7O4 nanoparticles, increases the active area between catalyst and reactants during the reaction, and accelerates the reaction process.42 The unique synergy between ferrite nanoparticles and RHC enhances catalytic activity. However, as the content of RHC increased to 70%, the degradation rate of BPA decreased, and the degradation rate decreased to 31.5% within 10 minutes. This is because the increase of RHC content will inevitably lead to the decrease of ZnCo1.3Fe0.7O4 content, so the catalytic activity of ZnCo1.3Fe0.7O4-y%RHC decreases. Therefore, 50 wt% is selected as the optimal content of RHC.
3.3.3 Effect of bisphenol A concentration on catalytic degradation of BPA. Fig. 8d shows the effect of the initial concentration of BPA on the catalytic performance of ZnCo1.3Fe0.7O4-50%RHC. The concentration of BPA increased from 20 mg L−1 to 50 mg L−1, and the degradation rate of BPA decreased from 96.3% to 48.0% within 10 minutes. However, there is a lower concentration of BPA in the industrial waste water. So it is more meaningful to set a lower concentration of BPA from the perspective of practical application.
3.3.4 Effect of the catalyst dosage on the catalytic degradation of BPA. The effect of the ZnCo1.3Fe0.7O4-50%RHC dosages on BPA degradation is shown in Fig. 9a. When the dosage of ZnCo1.3Fe0.7O4-50%RHC is 0.25 g L−1, 0.40 g L−1, 0.50 g L−1 and 0.75 g L−1, the catalytic rates of BPA reach 74.1%, 82.6%, 94.85% and 100% within 15 minutes. However, when the dosage of ZnCo1.3Fe0.7O4-50%RHC is increased to 1.00 g L−1, ZnCo1.3Fe0.7O4-50%RHC has an inhibitory effect on the degradation of BPA, which is due to excessive catalytic active sites (Fe2+, Co2+) quenching effect on SO4˙− (eqn (7) and (8)).43 |
Co2+ + SO4˙− → SO42− + Co3+
| (7) |
|
Fe2+ + SO4˙− → SO42− + Fe3+
| (8) |
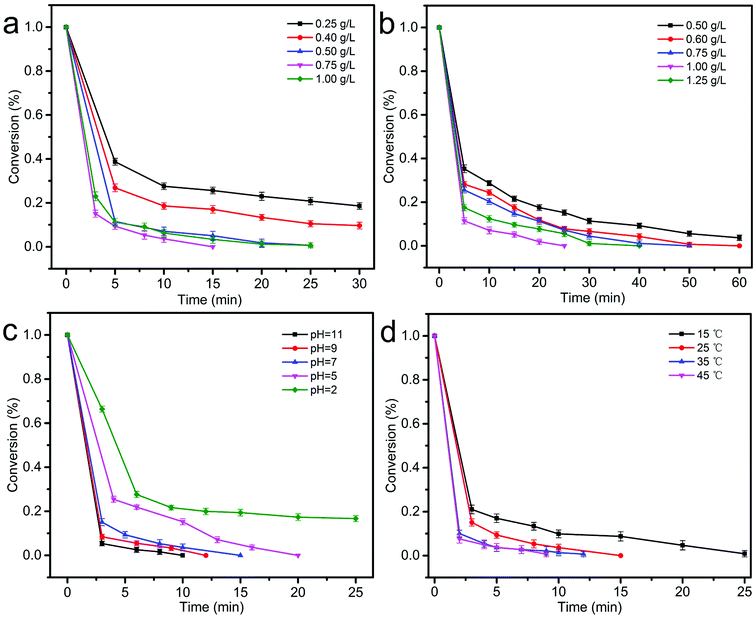 |
| Fig. 9 Effect of the catalyst dosage on the catalytic degradation of BPA (a). Effect of the PS dosage on the catalytic degradation of BPA (b), effect of pH on the catalytic performance of ZnCo1.3Fe0.7O4-50%RHC (c). Effect of temperature on the catalytic performance of ZnCo1.3Fe0.7O4-50%RHC (d). ([catalyst] = 0.75 g L−1, [BPA] = 20 mg L−1, [PS] = 1.00 g L−1, initial pH = 7, T = 25 °C). | |
3.3.5 Effect of the PS dosage on the catalytic degradation of BPA. The effect of PS concentration on BPA degradation in ZnCo1.3Fe0.7O4-50%RHC/PS system is studied, and as shown in Fig. 9b. When the concentration of PS increased from 0.5 g L−1 to 1.00 g L−1, the BPA degradation rate of ZnCo1.3Fe0.7O4-50%RHC/PS system increased from 78.6% to 94.9% within 15 min. However, when the concentration of PS further increased to 1.25 g L−1, the degradation rate of BPA decreased to 90.0% within 15 min. It suggests that higher PS concentration does not lead to better BPA degradation rates. This may be caused by the auto-coupling reaction of SO4˙− (eqn (9) and (10)).44,45 |
SO4˙− + SO4˙−→ S2O82−
| (9) |
|
SO4˙−+ S2O82− → S2O8˙−+ SO42−
| (10) |
3.3.6 Effect of pH on the catalytic performance of ZnCo1.3Fe0.7O4-50%RHC. Solution pH has a significant impact on the performance of various redox systems. Fig. 9c shows the effect of solution pH on the catalytic oxidation of BPA. As the pH value increases, the degradation rate of BPA also increases, indicating that the ZnCo1.3Fe0.7O4-50%RHC composite can increase the degradation rate of BPA under alkaline conditions. Because Co2+ on the surface of ZnCo1.3Fe0.7O4-50%RHC reacts with H2O to form CoOH+ (eqn (11)). CoOH+ activates PS to generate SO4˙− (eqn (12)).46 SO4˙− has a high redox potential and can efficiently oxidize BPA. Under alkaline conditions, SO4˙− can be transformed into surface-bound hydroxyl radicals (˙OH) (eqn (13)).47 Under acidic conditions, the production of CoOH+ is limited.48 In addition, under acidic conditions, excess hydrogen ions can scavenge sulfate radicals and hydroxyl radicals to adversely affect catalysis (eqn (14)). And strong acid conditions inhibit the activation of persulfate through acid catalyzing persulfate (eqn (15) and (16)).49 Therefore, alkaline conditions are conducive to the degradation of BPA. |
CoOH+ + S2O82− → CoOH2+ + SO42− + SO4˙−
| (12) |
|
SO4˙− + OH− + H+ → ˙OH + SO42− + H+
| (13) |
|
SO4˙−/˙OH + H+ + e− → HSO4˙−/H2O
| (14) |
|
H+ + S2O82− → HS2O8−
| (15) |
|
HS2O8− + H2O → H2SO5 + HSO4−
| (16) |
3.3.7 Effect of temperature on the catalytic performance of ZnCo1.3Fe0.7O4-50%RHC. The effect of temperature on BPA degradation is shown in Fig. 9d. It can be seen from that the degradation rate is positively correlated with the reaction temperature. When the temperature drops from 25 °C to 15 °C, the time for complete BPA degradation increase from 15 min to 25 min. When the temperature increases to 35 °C and 45 °C, the complete BPA degradation reaction time are 12 min and 9 min. With the temperature from 15 °C to 45 °C, k of BPA degradation increases greatly from 0.0896 to 0.3448 min−1. It has been reported50 that high temperature could promote PS decomposition to form active radicals and also speed up the collision frequency between PS and pollutants and catalysts. According to Arrhenius equation
, where k is the rate constant, universal gas constant R = 8.314 J mol−1 K−1, and A is a constant. The Ea of the ZnCo1.3Fe0.7O4-50%RHC activated PS system is 34.24 kJ mol−1, which is lower than previously reported Ea of BPA degradation activated only by heating (133.5 kJ mol−1).51 However, due to the high requirements for high temperature energy input, high temperature is not suitable for large-scale applications for considering actual production. In addition, the catalytic performance of ZnCo1.3Fe0.7O4-50%RHC is compared with the recently reported catalysts (Table 4), and the catalytic performance of ZnCo1.3Fe0.7O4-50%RHC is significantly better than that of other catalysts.
Table 4 Comparison of catalytic performance of ZnCo1.3Fe0.7O4-50%RHC with recently reported catalysts toward the degradation of BPAa
Catalyst |
mcat (g L−1) |
mPS (mg L−1) |
CBPA (mg L−1) |
Time (min) |
R (%) |
Ref. |
mcat is the dosage of catalyst, mPS is the dosage of PS, CBPA is the concentration of BPA, R is the removal efficiency. |
ZnCo1.3Fe0.7O4-50%RHC |
0.75 |
1.00 |
20 |
15 |
100 |
This work |
Fe3S4 |
0.2 |
0.22 |
5 |
120 |
95 |
52 |
CuFe2O4/kaolinite |
0.5 |
0.5 |
50 |
60 |
100 |
53 |
Mn0.6Zn0.4Fe2O4 |
0.2 |
0.5 |
10 |
60 |
100 |
54 |
3.4. Effect of anions
In general, inorganic salts are abundant in water bodies. The effects of Cl−, NO3−, HCO3− and H2PO4− 4 inorganic anions on BPA degradation in ZnCo1.3Fe0.7O4-RHC/PS system are explored.
3.4.1 Effect of Cl− on the catalytic performance of ZnCo1.3Fe0.7O4-50%RHC. Fig. 10a shows the effect of Cl− concentration on catalytic degradation of BPA. When the Cl− concentration increases from 0 mM to 10 mM, the degradation rate of BPA decreases with the increase of Cl− concentration. However, when the Cl− concentration increases from 10 mM to 30 mM, the degradation rate of BPA increases with the increase of Cl− concentration. This may be under the condition of low Cl− concentration, both SO4˙− or ˙OH are likely to oxidize Cl− to the less reactive Cl˙ and HOCl− (E0(Cl2/Cl−) = 1.36 V, E0(HOCl/Cl−) = 1.36 V) (eqn (17)–(19)).55 At a high Cl− concentration, excessive Cl− in ZnCo1.3Fe0.7O4-RHC/PS system will react with PS to form highly oxidizing HOCl and Cl2 (eqn (20) and (21)).56 Therefore, Cl− can increase the degradation rate of BPA in a certain range. |
HOCl˙− + H+ → Cl˙ + H2O
| (18) |
|
Cl− + SO4˙− → Cl˙ + SO42−
| (19) |
|
Cl− + HSO5− → SO42− + HOCl
| (20) |
|
2Cl− + HSO5− + H+ → SO42− + Cl2 + H2O
| (21) |
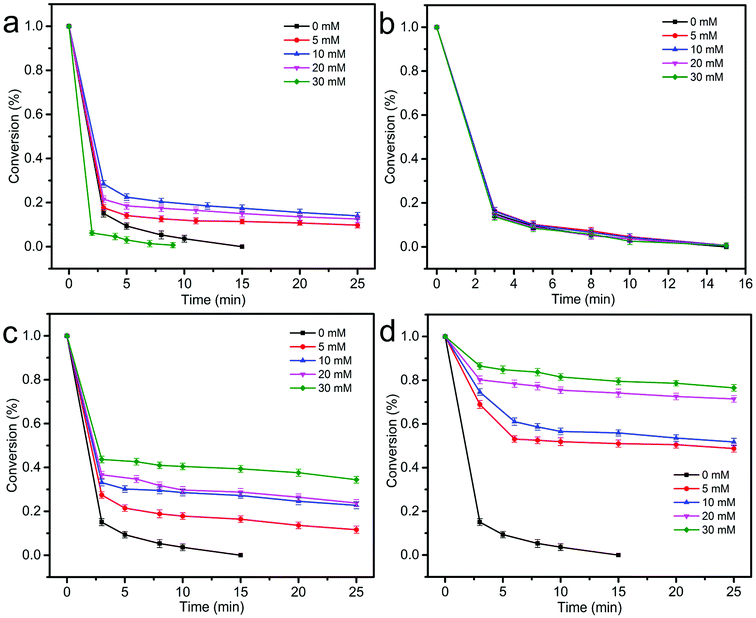 |
| Fig. 10 Effect of various co-anion on BPA degradation: Cl− (a), NO3− (b), HCO3− (c), H2PO4− (d). ([catalyst] = 0.75 g L−1, [BPA] = 20 mg L−1, [PS] = 1.00 g L−1, initial pH = 7, T = 25 °C). | |
3.4.2 Effect of NO3− on the catalytic performance of ZnCo1.3Fe0.7O4-50%RHC. Fig. 10b shows the effect of NO3− concentration on catalytic BPA degradation. It can be seen that NO3− has almost no effect on the degradation of BPA in ZnCo1.3Fe0.7O4-RHC/PS system, and the degradation curves of BPA by different concentrations of NO3− are almost identical. This is due to the reaction rate of NO3− and SO4˙− is slow (k = (5.6 ± 0.5) × 104 M−1 s−1) and the weak reactivity of
(eqn (22)).57 In addition, NO3− does not generate other free radicals with PS. Moreover, ZnCo1.3Fe0.7O4-RHC/PS system has good stability and is not easily affected by changes of external environment. |
 | (22) |
3.4.3 Effect of HCO3− on the catalytic performance of ZnCo1.3Fe0.7O4-50%RHC. As shown in Fig. 10c, the influence of different concentrations of HCO3− on the catalytic oxidation of BPA by ZnCo1.3Fe0.7O4-RHC is investigated. As can be seen from the figure, with the concentration of HCO3− increasing from 0 mM to 30 mM, the BPA degradation rate decreases from 96.3% to 59.6% within 10 min. Because HCO3− and CO32− react with strongly oxidizing SO4˙− to produce less oxidizing CO3˙− and
(eqn (23)–(27)), so HCO3− inhibits BPA degradation.58 |
 | (23) |
|
 | (24) |
|
CO32− + SO4˙− → SO42− + CO3˙−
| (25) |
|
˙OH + HCO3− → CO32− + H2O
| (26) |
|
CO32− + ˙OH → OH− + CO3˙−
| (27) |
3.4.4 Effect of H2PO4− on the catalytic performance of ZnCo1.3Fe0.7O4-50%RHC. As shown in Fig. 10d, the effect of different concentrations of H2PO4− on the catalytic oxidation of BPA by ZnCo1.3Fe0.7O4-RHC is investigated. Fig. 10d shows that the degradation rate of BPA decreases with the increase of H2PO4− concentration, indicating that the presence of H2PO4− inhibits the degradation of BPA. The effect of H2PO4− on catalytic oxidation of BPA by ZnCo1.3Fe0.7O4-RHC was far greater than that of Cl−, NO3− and HCO3−. This is due to the affinity of H2PO4− to ZnCo1.3Fe0.7O4-RHC, and binds to the Co–OH on the surface of ZnCo1.3Fe0.7O4 through a chelation reaction, reducing the catalytic activity of Co (eqn (28)–(30)). Therefore, the inhibition of H2PO4− on the degradation of BPA by ZnCo1.3Fe0.7O4-RHC can be considered to be related to the substitution of hydroxyl groups on the catalyst surface.59 |
Co–OH + H2PO4− ↔ CoH2PO4 + OH−
| (28) |
|
Co–OH + H2PO4− ↔ CoHPO4− + H2O
| (29) |
|
Co–OH + H2PO4− → CoPO42− + H2O + H+
| (30) |
3.5. Stability and reusability
The stability and cyclability of ZnCo1.3Fe0.7O4-RHC are very important to its practical application. In order to determine the cyclability and stability of ZnCo1.3Fe0.7O4-RHC in PS oxidation system, the particles are recovered (such as centrifugation and magnetic adsorption), washed multiple times with deionized water and ethanol, and dried at 70 °C. ZnCo1.3Fe0.7O4-RHC still maintained good catalytic activity, and BPA can be completely degraded within 40 min after 5 consecutive uses (Fig. 11a). The catalytic activity of ZnCo1.3Fe0.7O4-RHC does not decrease significantly. The slight decrease in BPA degradation rate after 5 cycles is due to the slight aggregation of ZnCo1.3Fe0.7O4-RHC, resulting in the reduction of catalytic active sites. As shown in the inset of Fig. 11a, the homogeneously dispersed ZnCo1.3Fe0.7O4-50%RHC is collected by simply applying a magnetic field. The result strongly indicates that ZnCo1.3Fe0.7O4-50%RHC has considerable magnetic. In addition, comparing the XRD and FTIR patterns of ZnCo1.3Fe0.7O4-RHC before and after the reaction (Fig. 11b and c), no significant changes are found, indicating that ZnCo1.3Fe0.7O4-RHC composite maintains good reuse performance and long service life. Meanwhile, the leaching concentration of metal ions in ZnCo1.3Fe0.7O4-RHC after each cycle was measured by ICP (Fig. 11d). The leaching concentrations of Zn, Fe and Co are all less than 53.3 μg L−1, 31.2 μg L−1 and 48.7 μg L−1, which are lower than Chinese industrial wastewater discharge standards (GB 8978-2002).60 Compared with cobalt ferrite with no Zn added (Table 5), the leaching rate of each element decreased. This may be due to the smaller ion radius of Zn2+, the electrostatic coulomb force increases, so that Zn and Co attract more closely.26 After the reaction, the metal content of ZnCo1.3Fe0.7O4-RHC composite material did not change much, indicating that ZnCo1.3Fe0.7O4-RHC composite material has good stability.
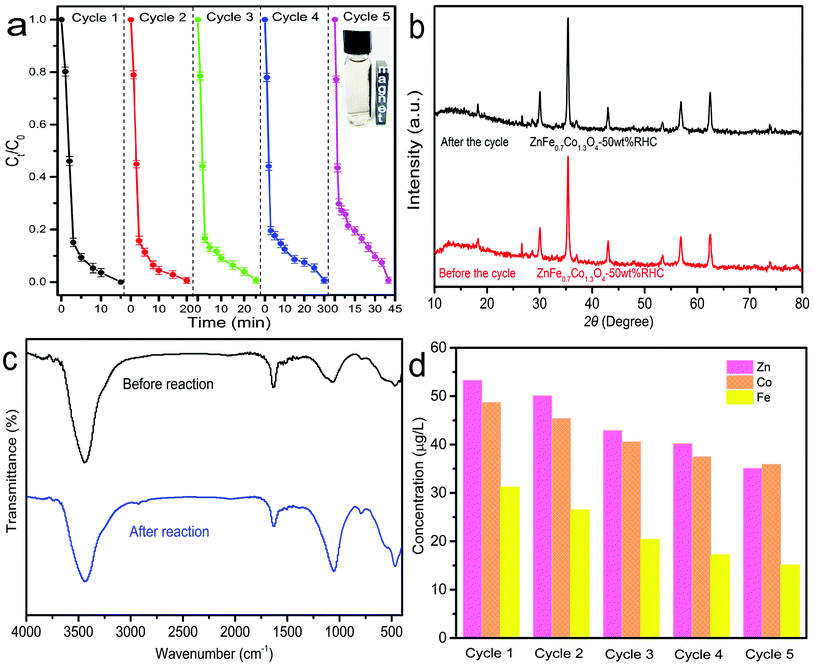 |
| Fig. 11 The reusability of ZnCo1.3Fe0.7O4-RHC for BPA degradation in presence of PMS (a). XRD patterns of ZnCo1.3Fe0.7O4-RHC before and after the reaction (b). FTIR patterns of ZnCo1.3Fe0.7O4-RHC before and after the reaction (c). The leakage of Fe, Co and Zn ions after five cycles of BPA degradation by ZnCo1.3Fe0.7O4-RHC and PMS system (d). ([catalyst] = 0.75 g L−1, [BPA] = 20 mg L−1, [PS] = 1.00 g L−1, initial pH = 7, T = 25 °C). | |
Table 5 Comparison of leaching rate of each element of ZnCo1.3Fe0.7O4-50%RHC with recently reported CoFe2O4 catalysts after degradation of BPA
Catalyst |
Co (μg L−1) |
Fe (μg L−1) |
Ref. |
ZnCo1.3Fe0.7O4-50%RHC |
48.7 |
31.2 |
This work |
CoFe2O4 |
410 |
50 |
61 |
CoFe2O4@graphene |
430 |
160 |
60 |
CoFe2O4-SAC |
570 |
250 |
62 |
CoFe2O4 |
763 |
68 |
63 |
3.6. Radical identification and catalytic mechanism
The early report has confirmed that PS could be activated to generate free radicals to oxidize organic pollutants, that is, degradation through free radical pathway.64 Therefore, the degradation efficiency of organic pollutants was inhibited after adding free radical capture agent. However, Duan et al.65 used nitrogen-doped carbon nanotubes to degrade phenol through a non-radical process. The degradation rate of organic pollutants is not completely inhibited by the addition of high concentration of free radical trapping agents. Therefore, it is necessary to determine main active substances and reaction pathway in ZnCo1.3Fe0.7O4-RHC/PS system.
The main active substances are determined by the corresponding free radical capture experiment in ZnCo1.3Fe0.7O4-RHC/PS system.66 Adding EtOH and TBA to the reaction system can distinguish the contribution of SO4˙− and ˙OH to the ZnCo1.3Fe0.7O4-RHC/PS system. Because EtOH can quickly react with SO4˙− (k = 1.6 × 107 M−1 s−1) and ˙OH (1.9 × 109 M−1 s−1). Besides, TBA can react quickly with ˙OH (k = 6.0 × 107 M−1 s−1), and react slowly with SO4˙− (k = 4.0 × 105 M−1 s−1).63 As shown in Fig. 12a, with the increase of TBA concentration, the degradation rate of BPA decreases from 100% to 26.8% within 15 minutes (n[TBA/PS] = 1000). TBA inhibited the degradation of BPA, confirming the existence of ˙OH in the system. Compared with TBA, EtOH further inhibits the degradation of BPA, and the degradation rate of BPA is reduced from 100% to 9.1% within 15 minutes (n[EtOH/PS] = 1000, Fig. 12b). It shows that besides ˙OH, there is also SO4˙− in ZnCo1.3Fe0.7O4-RHC/PS system. BQ and FFA are used as capture agents for O2˙− (k = 9.6 × 108 M−1 s−1) and 1O2 (k = 2 × 109 M−1 s−1).67 Fig. 12c shows that in the presence of BQ, the degradation efficiency of BPA can still reach 100%. The result shows that O2˙− does not participate in the oxidative degradation of BPA. However, the degradation rate of BPA is reduced, because BQ is adsorbed on the surface of the catalyst through the interaction with RHC, which hinders the activation of PS by the catalyst. After adding FFA, the degradation rate of BPA within 50 minutes is only 51.3% (n[FFA/PS] = 600). It shows that 1O2 plays an important role in the degradation of BPA in the ZnCo1.3Fe0.7O4-RHC/PS system.
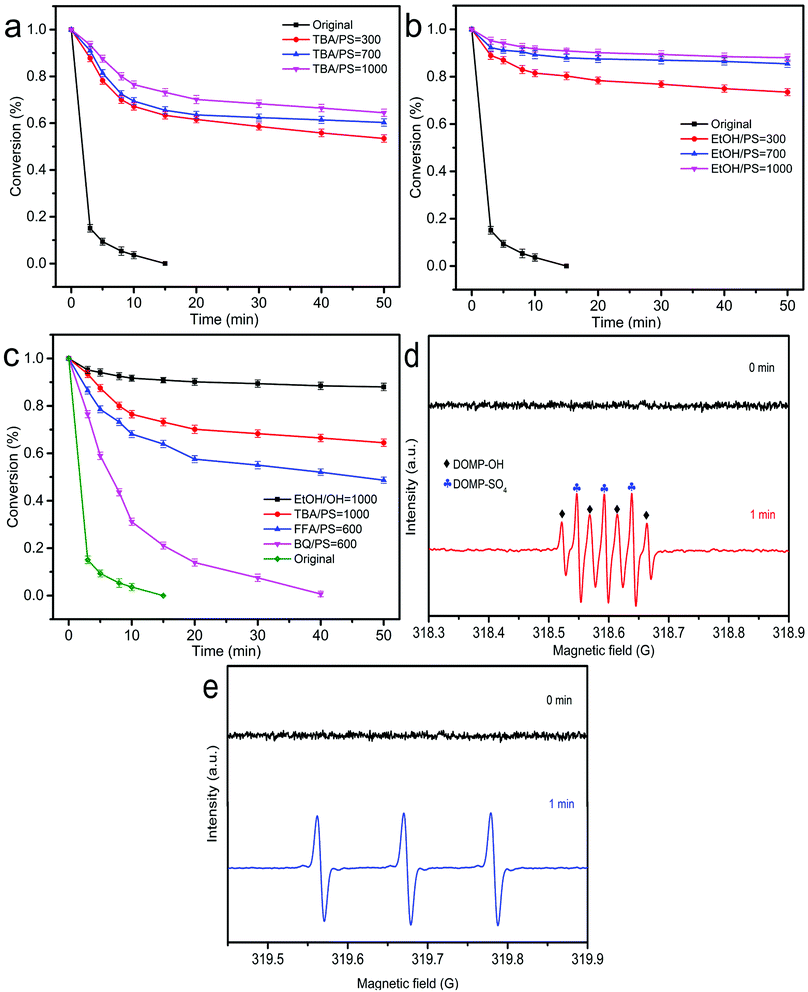 |
| Fig. 12 Effect of TBA (a), EtOH (b) (with different molar ratio to PS) on the degradation of BPA. Effect of BQ and FFA (with fixed molar ratio to PS) on the degradation of BPA (c). ESR tests of SO4˙−, ˙OH (d) and 1O2 (e). ([catalyst] = 0.75 g L−1, [BPA] = 20 mg L−1, [PS] = 1.00 g L−1, initial pH = 7, T = 25 °C). | |
The types of free radicals produced during the reaction are further determined by ESR. Fig. 12d shows that ZnCo1.3Fe0.7O4-RHC activates PS with 7 typical DMPO-X peaks, and shows strong ˙OH and SO4˙− radical signals, indicating the presence of ˙OH and SO4˙− in the reaction system of ZnCo1.3Fe0.7O4-RHC. The signal of SO4˙− is stronger than that of ˙OH. Since ˙OH is produced by the reaction of SO4˙− with H2O (eqn (32)), and SO4˙− determines the amount of ˙OH. It further illustrates that SO4˙− plays a dominant role in degradation. Fig. 12e shows the ESR test results using TEMP as the capture agent. It shows a typical ternary peak with an intensity of 1
:
1
:
1, confirming the presence of 1O2. Based on the results of free radical capture experiment and ESR test, SO4˙− dominates the ZnCo1.3Fe0.7O4-RHC/PS degradation system, and ˙OH and 1O2 play important roles.
Even when EtOH, TBA, and FFA are added to the ZnCo1.3Fe0.7O4-RHC/PS degradation system at the molar ratio of EtOH/PS = 1000, TBA/PS = 1000, and FFA/PS = 6000, BPA can not be completely degraded. This indicates that there may be a non-radical process in the ZnCo1.3Fe0.7O4-RHC/PS system.65
In this study, the synergy between ZnCo1.3Fe0.7O4 nanoparticles and RHC also plays a crucial role in the excellent catalytic performance of ZnCo1.3Fe0.7O4-RHC/PS. Studies68 have been reported that catalyst with graphitized structure could induce non-radical process, by using the carbon-based graphitized structure as a bridge to transfer electrons between the target pollutant and PS. And in this study, the electrons in Co(II) and Fe(II) can be easily transferred to the sp2 hybridized carbon in the graphitized structure, so that RHC has a large number of free-flowing delocalized π electrons. RHC with a large number of free-flowing delocalized electrons promotes the transfer of electrons from BPA (electron donor) adsorbed on the surface of ZnCo1.3Fe0.7O4-RHC to PS to generate reactive complexes (electron acceptors). Furthermore, Fig. 8c also shows that when the RHC content increases from 0% to 50%, the degradation rate of BPA increases by 36.4% within 15 minutes, indicating that part of BPA degradation is realized through the non-free radical pathway of RHC.
By comparing the XPS spectra of ZnCo1.3Fe0.7O4-RHC before and after the reaction, the activation mechanism of PS and transition metals in ZnCo1.3Fe0.7O4-RHC system is investigated (Fig. 13). The change of Co 2p3/2 in ZnCo1.3Fe0.7O4-RHC after reaction is observed in Fig. 13c, showing that 44.4% of Co(III) is converted to Co(II). Moreover, compared with the binding energy of 780.4 eV of Co before catalytic reaction, the binding energy of Co after reaction is converted to a higher 780.9 eV. S2O82− reacts with H2O to produce HSO5− (eqn (31)). Since the redox potential of Co(III)/Co(II) (1.81 V) is higher than that of HSO5−/SO5˙− (1.10 V), HSO5−/SO5˙− can reduce Co(III) to Co(II) (eqn (32)).69 Then, Co(II) is oxidized by S2O82− to produce Co(III), which realizes the cyclic regeneration of Co(III) and Co(II) (eqn (33)). Fe 2p also shows similar changes to Co 2p. In the used ZnCo1.3Fe0.7O4-RHC catalyst, Fe(III) accounts for 52.8% and Fe(II) accounts for 47.2%. H2O can be dissociated on Fe3+ to form FeOH2+ (eqn (34)). FeOH2+ can easily interact with Co(II) to form CoOH+ (eqn (35)). Besides, the highly reactive Co(II) reacts with H2O to form CoOH+ (eqn (36)). CoOH+ reacts directly with S2O82− to generate SO4˙− (eqn (9)). SO4˙− can react with H2O to generate ˙OH (eqn (11)). At the same time, Fe(III) is reduced by S2O82− to produce Fe(II) (eqn (37)). However, the redox potential of Fe(III)/Fe(II) (0.77 V) is lower than that of HSO5−/SO5˙− (1.10 V), so Fe(III) cannot be reduced by HSO5−. Then, Fe(II) is oxidized by SO4˙− or ˙OH to produce Fe(III) (eqn (38)), achieving Fe(III) regeneration. These results indicate that both redox pairs Co(III)/Co(II) and Fe(III)/Fe(II) are involved in the activation of PS by ZnCo1.3Fe0.7O4-RHC. In addition, FFA free radical capture experiment and TEMP capture experiment have confirmed that 1O2 plays an important role in BPA degradation process. Compared with the C XPS spectra of unused and used ZnCo1.3Fe0.7O4-RHC, the content of C
O functional group decreases from 17.6% to 6.2%, while the content of C–O–C functional group increases from 22.2% to 32.8%, and the content of C–OH functional group increases from 60.2% to 61.0%. It has been reported that C
O could promote the self-decomposition of PS, thereby producing produce 1O2.70 The C XPS spectrum further confirms the importance of 1O2 in the degradation system of ZnCo1.3Fe0.7O4-RHC/PS. The HSO5− is produced in the reaction self-decomposes to produce SO52− (eqn (39)). What's more, SO5˙− interacts with HSO5− to generate 1O2 (eqn (40)). And SO5˙− can react with itself or H2O to generate 1O2 (eqn (41)–(43)). Finally, ZnCo1.3Fe0.7O4-RHC is used to activate PS to produce SO4˙−, ˙OH and 1O2 to decompose BPA into CO2 and H2O (eqn (44)). The mechanism of ZnCo1.3Fe0.7O4-RHC activated PS to degrade BPA is shown in Fig. 14.
|
S2O82− + 2H2O − 2e− → 2HSO5− + 2H+
| (31) |
|
Co3+ + HSO5− → Co2+ + SO5˙− + H+
| (32) |
|
S2O82− + Co2+ → Co3+ + SO42− + SO4˙−
| (33) |
|
Fe3+ + H2O → FeOH2+ + H+
| (34) |
|
Co2+ + FeOH2+ → CoOH+ + Fe3+
| (35) |
|
SO4˙− + H2O → HSO4− + ˙OH
| (36) |
|
Fe3+ + S2O82− → Fe2+ + S2O8˙−
| (37) |
|
Fe2+ + SO4˙−/˙OH → Fe3+ + SO42−/OH−
| (38) |
|
HSO5− + SO52− → SO42− + HSO4− + 1O2
| (40) |
|
2SO5˙− + H2O → 3/21O2 + 2HSO4−
| (41) |
|
2SO5˙− → 2SO42− + 1O2
| (42) |
|
2SO5˙− → S2O82− + 1O2
| (43) |
|
SO4˙−/˙OH/1O2 + BPA → intermediates → CO2 + H2O
| (44) |
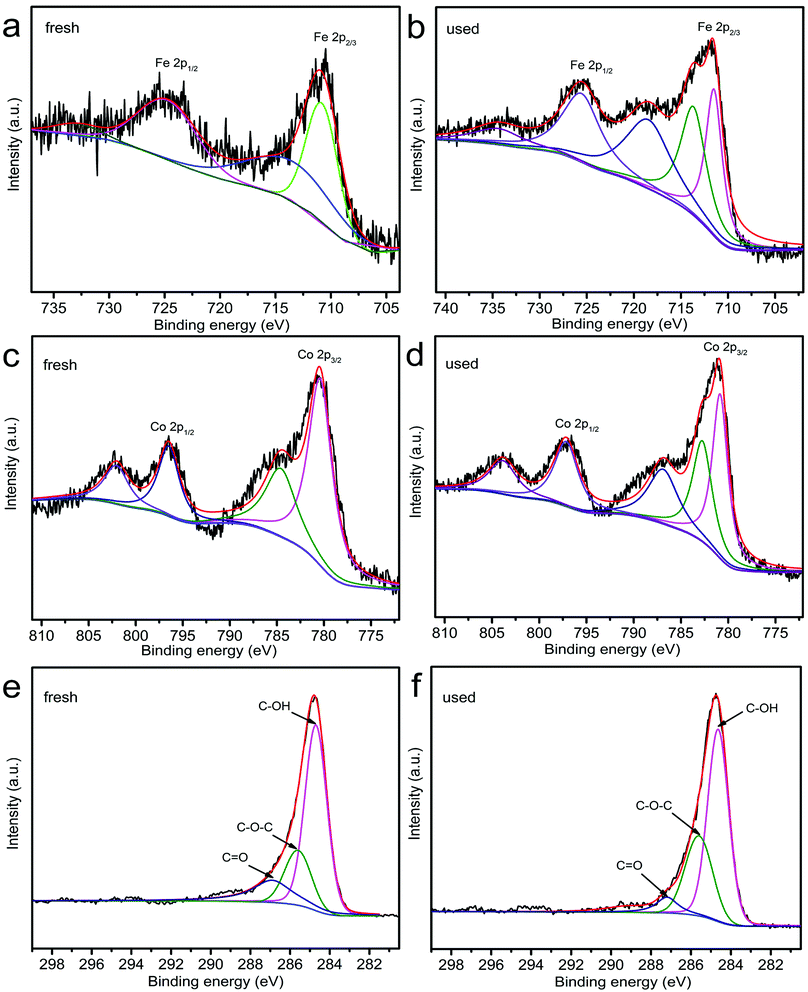 |
| Fig. 13 XPS spectra of Fe 2p, Co 2p and C for fresh (a, c, e) and used (b, d, f) ZnCo1.3Fe0.7O4-RHC. | |
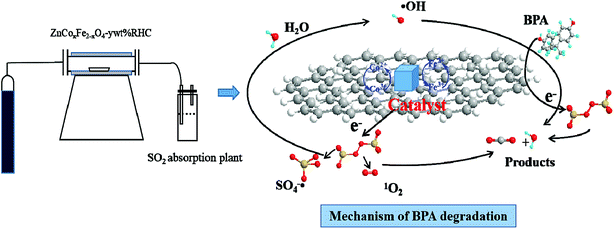 |
| Fig. 14 Mechanism of the activation on PS by ZnCo1.3Fe0.7O4-RHC for the degradation of BPA. | |
4 Conclusions
In this study, a novel composite of ZnCo1.3Fe0.7O4 spherical nanoparticles with primary particle size of about 13 nm interspersed on biological carbon base (RHC) was designed and prepared by solid phase method, using waste ferrous sulfate as the iron source. When used as a polyphase catalyst to activate PS to degrade BPA, the as-obtained ZnCo1.3Fe0.7O4-50%RHC composite material could completely degrade BPA in 15 min, delivering better catalytic activity compared with the home-made ZnCo1.3Fe0.7O4, ZnFe2O4 and ZnCo2O4. A series of experiment exploring the effects of several parameters and anions on BPA degradation demonstrated that the ZnCo1.3Fe0.7O4-50%RHC possess excellent comprehensive performances including for PS activation. Stability and reusability tests substantiated that it still maintain excellent catalytic performance for BPA degradation after 5 cycles, and the leaching of metal ions is much lower than the discharge standard of wastewater. Based on free radical capture experiment, ESR test and XPS analysis, it is determined that there are free radical and non-radical pathways in the process of ZnCo1.3Fe0.7O4-50%RHC activation of PS to degrade BPA. We believe that this “use waste to treat waste” idea and these findings provide an important guiding role for the development of magnetic separation catalyst for wastewater treatment process, as well as expand a new way with potential application prospect for the resource utilization of industrial by-product ferrous sulfate.
Authors contribution
Yirui Shu: conceptualization, data curation,fFormal analysis, methodology, software, validation, writing – original draft, writing – review & editing. Pan Zhang: investigation, visualization, software. Yanjun Zhong: writing – review & editing. Xiangyang Xu: writing – review & editing. Genkuan Ren: methodology, resources. Wei Wang: validation. Hengli Xiang: supervision. Zhiye Zhang: funding acquisition, investigation. Xinlong Wang: visualization, investigation, project administration.
Conflicts of interest
The authors declare that they have no known competing financial interests or personal relationships that could have appeared to influence the work reported in this paper.
Acknowledgements
This work was funded by the financial supported from Sichuan University-Panzhihua City Science and Technology Cooperation Special Fund for Titanium White by-product Ferrous Sulfate Preparation 500 tons/year Nanometer iron Red Pigment and Co-production Sulfuric Acid Pilot Study Project (No. 2018CDPZH-5) and Sichuan Provincial Science and Technology Planning Project (No. 2019YFH0149).
References
- Y. Zhou, M. Chen, F. R. Zhao, D. Mu, Z. B. Zhang and J. Y. Hu, Ubiquitous occurrence of chlorinated byproducts of bisphenol A and nonylphenol in bleached food contacting papers and their implications for human exposure, Environ. Sci. Technol., 2015, 49, 7218–7226 Search PubMed.
- E. N. D. Freitas, G. A. Bubna, T. Brugnari, C. G. Kato, M. Nolli, T. G. Rauen, R. D. P. M. Moreira, R. A. Peralta and A. Bracht, Removal of bisphenol A and evaluation of ecotoxicity of degradation products by laccases from Pleurotus ostreatus, and Pleurotus pulmonarius, Chem. Eng. J., 2017, 330, 1361–1369 Search PubMed.
- E. J. Eio, M. Kawai, K. Tsuchiya, S. Yamamoto and T. Toda, Biodegradation of bisphenol A by bacterial consortia, Int. Biodeterior. Biodegrad., 2014, 96, 166–173 Search PubMed.
- C. M. Liu, Z. H. Diao, W. Y. Huo, L. J. Kong and J. J. Du, Simultaneous removal of Cu2+ and bisphenol A by a novel biocharsupported zero valent iron from aqueous solution: Synthesis, reactivity and mechanism, Environ. Pollut., 2018, 239, 698–705 Search PubMed.
- M. J. Xu, J. Li, Y. Yan, X. G. Zhao, J. F. Yan, Y. H. Zhang, B. Lai, X. Chen and L. P. Song, Catalytic degradation of sulfamethoxazole through peroxymonosulfate activated with expanded graphite loaded CoFe2O4 particle, Chem. Eng. J., 2019, 369, 403–413 Search PubMed.
- D. Yang and C. M. Ezyske, Sulfate radical-advanced oxidation process (SR-AOP) for simultaneous removal of refractory organic contaminants and ammonia in landfill leachate, Water Res., 2011, 45, 6189–6194 Search PubMed.
- J. Wang and S. Wang, Activation of persulfate (PS) and peroxymonosulfate (PMS) and application for the degradation of emerging contaminants, Chem. Eng. J., 2018, 334, 1502–1517 Search PubMed.
- Y. C. Lee, S. L. Lo, P. T. Chiueh and D. G. Chang, Efficient decomposition of perfluorocarboxylic acids in aqueous solution using microwave-induced persulfate, Water Res., 2009, 43, 2811–2816 Search PubMed.
- X. Zhang, M. Feng, R. Qu, H. Liu, L. S. Wang and Z. Y. Wang, Catalytic degradation of diethyl phthalate in aqueous solution by persulfate activated with nano-scaled magnetic CuFe2O4/MWCNTs, Chem. Eng. J., 2016, 301, 1–11 Search PubMed.
- G. P. Anipsitakis and D. D. Dionysiou, Radical generation by the interaction of transition metals with common oxidants, Environ. Sci. Technol., 2004, 38, 3705–3712 Search PubMed.
- Y. Huang, C. Han, Y. Liu, M. N. Nadagouda, L. Machala, K. E. O Shea, V. K. Sharma and D. D. Dionysiou, Degradation of atrazine by ZnxCu1-xFe2O4 nanomaterial-catalyzed sulfite under UV-vis light irradiation: Green strategy to generate SO4˙−, Appl. Catal., B, 2018, 221, 380–392 Search PubMed.
- M. D. Hossain, M. N. Khan, S. Sikder, M. N. I. Khan and M. A. Hakim, Frequency and temperature dependent magnetic properties with structural Rietveld refinement of Co0.25Zn0.75YxFe2-xO4 ferrite, J. Magn. Magn. Mater., 2020, 493, 165696 Search PubMed.
- S. Debnath, D. Krishna and B. Saha, X-ray diffraction analysis for the determination of elastic properties of zinc-doped manganese spinel ferrite nanocrystals (Mn0.75Zn0.25Fe2O4), along with the determination of ionic radii, bond lengths, and hopping lengths, J. Phys. Chem. Solids, 2019, 134, 105–114 Search PubMed.
- U. Wongpratat, P. Tipsawat, J. Khajonrit, E. Swatsitang and S. Maensir, Effects of Nickel and Magnesium on electrochemical performances of partial substitution in spinel ferrite, J. Alloys Compd., 2020, 831, 154718–154731 Search PubMed.
- H. Aali, N. Azizi, F. Kermani, M. Mashreghi, A. Youssefi, S. Mollazadeh, J. V. Khaki and H. Nasiri, High antibacterial and photocatalytic activity of solution combustion synthesized Ni0.5Zn0.5Fe2O4 nanoparticles: Effect of fuel to oxidizer ratio and complex fuels, Ceram. Int., 2019, 45, 19127–19140 Search PubMed.
- X. S. Rong, H. F. Chen, J. Rong, X. Y. Zhang, J. Wei, S. Liu, X. T. Zhou, J. C. Xu, F. X. Qiu and Z. R. Wu, An all-solid-state Z-scheme TiO2/ZnFe2O4 photocatalytic system for the N2 photofixation enhancement, Chem. Eng. J., 2019, 371, 286–293 Search PubMed.
- Q. R. Zhang, Z. W. Li, X. H. Li, L. G. Yu, Z. J. Zhang and Z. S. Wu, Zinc ferrite nanoparticle decorated boron nitride nanosheet: Preparation, magnetic field arrangement, and flame retardancy, Chem. Eng. J., 2019, 356, 680–692 Search PubMed.
- P. X. Liu, Y. M. Ren, W. J. Ma, J. Ma and Y. C. Du, Degradation of shale gas produced water by magnetic porous MFe2O4 (M = Cu, Ni, Co and Zn) heterogeneous catalyzed ozone, Chem. Eng. J., 2019, 345, 98–106 Search PubMed.
- J. N. Cui and H. L. Ren, Development and comparison of titanium dioxide production technology in China, Rare Met. Cem. Carbides, 2013, 41, 14–17 Search PubMed.
- H. Q. Li, A brief analysis of 20 years development of titanium dioxide sulphuric acid process, China Coat., 2019, 34, 7–10 Search PubMed.
- S. Bi, Status and development of Titanium dioxide industry in China in 2018, Iron, Steel, Vanadium, Titanium, 2018, 39, 1–4 Search PubMed.
- C. H. He, R. L. Shao and X. G. Liu, Progress in production technology of titanium dioxide, Eng. Technol., 2016, 5, 70–71 Search PubMed.
- Y. Wang, H. Sun, H. M. Ang, M. O. Tadé and S. Wang, Facile Synthesis of Hierarchically Structured Magnetic MnO2/ZnFe2O4 Hybrid Materials and Their Performance in Heterogeneous Activation of Peroxymonosulfate, ACS Appl. Mater. Interfaces, 2014, 6, 19914–19923 Search PubMed.
- X. Zhong, Z. S. Zou, H. L. Wang, W. Huang and B. X. Zhou, Enhanced Activation of Persulfate by Co-Doped Bismuth Ferrite Nanocomposites for Degradation of Levofloxacin Under Visible Light Irradiation, Mater. Sci., 2019, 12, 3952 Search PubMed.
- H. X. Zhang, C. W. Li, L. Lyu and C. Hu, Surface oxygen vacancy inducing peroxymonosulfate activation through electron donation of pollutants over cobalt-zinc ferrite for water purification, Appl. Catal., B, 2020, 270, 1873–1883 Search PubMed.
- S. N. Divya and K. Manju, Heterogeneous catalytic oxidation of persistent chlorinated organics over cobalt substituted zinc ferrite nanoparticles at mild conditions: Reaction kinetics and catalyst reusability studies, J. Environ. Chem. Eng., 2017, 5, 964–974 Search PubMed.
- J. C. C. Freitas, M. A. Schettino, A. G. Cunha, F. G. Emmerich, A. C. Bloise and E. R. Azevedo, NMR investigation on the occurrence of Na species in porous carbons prepared by NaOH activation, Carbon, 2007, 45, 1097–1104 Search PubMed.
- F. Y. Han, X. S. Xu, Y. S. Fu and X. Wang, Synthesis of Rice-Husk-Carbon-Supported Nickel Ferrite Catalyst for Reduction of Nitrophenols, J. Nanosci. Nanotechnol., 2019, 19, 5838–5846 Search PubMed.
- A. Arunkumar, D. Vanidha, R. Kannan and M. Shanmugam, Unusual Transition of Electrical and Magnetic Properties in the Nano to Bulk Transformation of Co-Zn Ferrites, J. Supercond. Novel Magn., 2020, 33, 1–15 Search PubMed.
- Y. Gong, D. Li, C. Luo, Q. Fu and C. Pan, Highly porous graphitic biomass carbon as advanced electrode materials for supercapacitors, Green Chem., 2017, 19, 4132–4140 Search PubMed.
- P. Laokul, V. Amornkitbamrung, S. Seraphin and S. Maensiri, Characterization and magnetic properties of nanocrystalline CuFe2O4, NiFe2O4, ZnFe2O4 powders prepared by the Aloe vera extract solution, Curr. Appl. Phys., 2011, 11, 101–108 Search PubMed.
- S. Patil, K. S. Anantharaju, D. Rangappa, Y. S. Vidya, S. C. Sharma, L. Renuka and H. Nagabhushana, Magnetic Eu-doped MgFe2O4 nanomaterials: An investigation of their structural, optical and enhanced visible-light-driven
photocatalytic performance, Environ. Nanotechnol. Monit. Manage., 2020, 13, 100268 Search PubMed.
- Z. Shao, T. Zeng, Y. He, D. Zhang and X. Pu, A novel magnetically separable CoFe2O4/Cd0.9Zn0.1S photocatalyst with remarkably enhanced H2 evolution activity under visible light irradiation, Chem. Eng. J., 2019, 359, 485–495 Search PubMed.
- Y. Zhao, Y. Xu, J. Zeng, B. Kong, X. Geng, D. Li, X. Gao, K. Liang, L. Xu, J. Lian, S. Huang, J. Qiu, Y. Huang and H. Li, Low-crystalline mesoporous CoFe2O4/C composite with oxygen vacancies for high energy density asymmetric supercapacitors, RSC Adv., 2017, 7, 55513–55522 Search PubMed.
- C. J. Li, B. Y. Ma and X. X. Huo, Characterization of graphitization degree of carbon/carbon composites, New Carbon Mater., 1999, 14, 19–25 Search PubMed.
- W. Xiaobo, Q. Yanlei, Z. Lihua and T. Heqing, Nitrogen-Doped Reduced Graphene Oxide as a Bifunctional Material for Removing Bisphenols: Synergistic Effect between Adsorption and Catalysis, Environ. Sci. Technol., 2015, 49, 6855–6864 Search PubMed.
- M. Feng, Y. Liu, Z. Zhao, H. Huang and Z. Peng, The preparation of Fe doped triclinic-hexagonal phase heterojunction WO3 film and its enhanced photocatalytic reduction of Cr (VI), Mater. Res. Bull., 2019, 109, 168–174 Search PubMed.
- S. Wang, J. Pu, Y. Tong, Y. Y. Cheng, Y. Gao and Z. H. Wang, ZnCo2O4 nanowire arrays grown on nickel foam for high-performance pseudocapacitors, J. Mater. Chem. A, 2014, 2, 5434–5440 Search PubMed.
- M. M. Wang, H. H. Wu, C. R. Shen, S. P. Luo, D. Wang and L. He, Seaweed-like 2D-2D Architecture of MoS2/rGO Composites for Enhanced Selective Aerobic Oxidative Coupling of Amines, Chemcatchem, 2019, 7, 1935–1942 Search PubMed.
- Z. Liu, S. Yang, Y. Yuan, J. Xu, Y. F. Zhu, J. J. Li and F. Wu, A novel heterogeneous system for sulfate radical generation through sulfite activation on a CoFe2O4 nanocatalyst surface, J. Hazard. Mater., 2017, 324, 583–592 Search PubMed.
- M. Kermani, F. Mohammadi and B. Kakavandi, Simultaneous catalytic degradation of 2,4-D and MCPA herbicides using sulfate radical-based heterogeneous oxidation over persulfate activated by natural hematite (α-Fe2O3/PS), J. Phys. Chem., 2018, 117, 49–59 Search PubMed.
- S. J. Olusegun, E. T. F. Freitas, L. R. S. Lara and N. D. S. Mohallem, Synergistic effect of a spinel ferrite on the adsorption capacity of nano bio-silica for the removal of methylene blue, Environ. Technol., 2019, 45, 1–33 Search PubMed.
- W. D. Oh and T. T. Lim, Design and application of heterogeneous catalysts as peroxydisulfate activator for organics removal: An overview, Chem. Eng. J., 2019, 358, 110–133 Search PubMed.
- J. Li, Y. Ren, F. Ji and B. Lai, Heterogeneous catalytic oxidation for the degradation of p -nitrophenol in aqueous solution by persulfate activated with CuFe2O4 magnetic nano-particles, Chem. Eng. J., 2017, 324, 63–73 Search PubMed.
- M. Li, Y. Xiong, X. Liu, X. Bo, Y. Zhang, C. Han and L. Guo, Facile synthesis of electrospun MFe2O4 (M = Co, Ni, Cu, Mn) spinel nanofibers with excellent electrocatalytic properties for oxygen evolution and hydrogen peroxide reduction, Nanoscale, 2015, 7, 8920–8930 Search PubMed.
- S. Su, W. Guo, Y. Leng, C. L. Yi and Z. M. Ma, Heterogeneous activation of oxone by CoxFe3−xO4 nanocatalysts for degradation of rhodamine B, J. Hazard. Mater., 2013, 244, 736–742 Search PubMed.
- C. Liang and H. W. Su, Identification of sulfate and hydroxyl radicals in thermally activated persulfate, Ind. Eng. Chem. Res., 2009, 48, 5558–5562 Search PubMed.
- M. Ahmadi, F. Ghanbari, A. Alvarez and S. S. Martinez, UV-LEDs assisted peroxymonosulfate/Fe2+ for oxidative removal of carmoisine: The effect of chloride ion, Korean J. Chem. Eng., 2017, 34, 1–8 Search PubMed.
- D. A. House, Kinetics and mechanism of oxidations by peroxydisulfate, Chem. Rev., 1962, 62, 185–203 Search PubMed.
- C. T. Chekem, S. Chiron, J. M. Mancaux, G. Plantard and V. Goetz, Thermal activation of persulfates for wastewater depollution on pilot scale solar equipment, Sol. Energy, 2020, 205, 372–379 Search PubMed.
- W. Zhang, Z. Zhou, X. Shan, R. Xu, Q. Chen, G. He, X. Sun and H. Chen, Solvent-thermal preparation of CuCo2O4/RGO heterocomposite: an efficient catalyst for the reduction of p-nitrophenol, New J. Chem., 2016, 40, 4769–4774 Search PubMed.
- X. Lin, K. Shih, J. Chen, X. Xie, Y. L. Zhang, Y. Chen, Z. Chen and Y. Li, Insight into flower-like greigite-based peroxydisulfate activation for effective bisphenol A abatement: performance and electron transfer mechanism, Chem. Eng. J., 2020, 391, 123558 Search PubMed.
- X. B. Dong, B. X. Ren, Z. M. Sun, C. Q. Li, X. W. Zhang, M. H. Kong, S. L. Zheng and D. D. Dionysiou, Monodispersed CuFe2O4 nanoparticles anchored on natural kaolinite as highly efficient peroxymonosulfate catalyst for bisphenol A degradation, Appl. Catal., B, 2019, 253, 206–217 Search PubMed.
- H. Lin, S. M. Li, B. Deng, W. H. Tan, R. M. Li, Y. Xu and H. Zhang, Degradation of bisphenol A by activating peroxymonosulfate with Mn0.6Zn0.4Fe2O4 fabricated from spent Zn-Mn alkaline batteries, Chem. Eng. J., 2019, 364, 541–551 Search PubMed.
- R. Yuan, S. N. Ramjaun, Z. Wang and J. Liu, Effects of chloride ion on degradation of Acid Orange 7 by sulfate radical-based advanced oxidation process: implications for formation of chlorinated aromatic compounds, J. Hazard. Mater., 2011, 196, 173–179 Search PubMed.
- Z. Wang, R. Yuan, Y. Guo, L. Xu and J. Liu, Effects of chloride ions on bleaching of azo dyes by Co2+/oxone regent: Kinetic analysis, J. Hazard. Mater., 2011, 190, 1083–1087 Search PubMed.
- P. Villegas-Guzman, F. Hofer, J. Silva-Agredo and R. A. Torres-Palma, Role of sulfate, chloride, and nitrate anions on the degradation of fluoroquinolone antibiotics by photoelectro-Fenton, Environ. Sci. Pollut. Res. Int., 2017, 24, 1–15 Search PubMed.
- C. Tan, N. Gao, D. Fu, D. Jing and D. Lin, Efficient degradation of paracetamol with nanoscaled magnetic CoFe2O4 and MnFe2O4 as a heterogeneous catalyst of peroxymonosulfate, Sep. Purif. Technol., 2017, 175, 47–57 Search PubMed.
- Y. H. Guan, J. Ma, Y. M. Ren, Y. L. Liu, J. Y. Xiao, L. Q. Lin and C. Zhan, Efficient degradation of atrazine by magnetic porous copper ferrite catalyzed peroxymonosulfate oxidation via the formation of hydroxyl and sulfate radicals, Water Res., 2013, 47, 5431–5438 Search PubMed.
- X. Yu, J. Qu, Z. Yuan, P. Min, S. Hao, Z. Zhu, X. Li, D. Yang and Z. Yu, Anisotropic CoFe2O4@Graphene Hybrid Aerogels with High Flux and Excellent Stability as Building Blocks for Rapid Catalytic Degradation of Organic Contaminants in a FlowType Setup, ACS Appl. Mater. Interfaces, 2019, 11, 34222–34231 Search PubMed.
- Q. Song, Y. Feng, Z. Wang, G. Liu and W. Lv, Degradation of triphenyl phosphate (TPhP) by CoFe2O4-activated peroxymonosulfate oxidation process: Kinetics, pathways, and mechanisms, Sci. Total Environ., 2019, 681, 331–338 Search PubMed.
- Z. Q. Yang, Y. Li, X. Y. Zhang, X. D. Cui, S. He, H. Liang and A. Ding, Sludge activated carbon-based CoFe2O4-SAC nanocomposites used as heterogeneous catalysts for degrading antibiotic
norfloxacin through activating peroxymonosulfate, Chem. Eng. J., 2020, 384, 123319 Search PubMed.
- Y. Fan, Y. Zhou, Y. Feng, P. Wang, X. Y. Li and K. Shih, Fabrication of reactive flat-sheet ceramic membranes for oxidative degradation of ofloxacin by peroxymonosulfate, J. Membr. Sci., 2020, 611, 118302 Search PubMed.
- M. Xu, J. Li, Y. Yan, X. Zhao, J. Yan, Y. Zhang, B. Lai, X. Chen and L. Song, Catalytic degradation of sulfamethoxazole through peroxymonosulfate activated with expanded graphite loaded CoFe2O4 particles, Chem. Eng. J., 2019, 369, 403–413 Search PubMed.
- X. Duan, H. Sun and Y. Wang, N-Doping-Induced Nonradical Reaction on Single-Walled Carbon Nanotubes for Catalytic Phenol Oxidation, ACS Catal., 2015, 5, 553–559 Search PubMed.
- X. Q. Zhou, C. G. Luo, M. Y. Luo, Q. L. Wang, J. Wang, Z. W. Liao, Z. L. Chen and Z. Q. Chen, Understanding the synergetic effect from foreign metals in bimetallic oxides for PMS activation: A common strategy to increase the stoichiometric efficiency of oxidants, Chem. Eng. J., 2020, 381, 122587 Search PubMed.
- G. X. Huang, C. Y. Wang, C. W. Yang, P. C. Guo and H. Q. Yu, Degradation of bisphenol A by peroxymonosulfate catalytically activated with Mn1.8Fe1.2O4 nanospheres: Synergism between Mn and Fe, Environ. Sci. Technol., 2017, 51, 12611–12618 Search PubMed.
- H. Fu, S. Ma, P. Zhao, S. Xu and S. Zhan, Activation of peroxymonosulfate by graphitized hierarchical porous biochar and MnFe2O4 magnetic nanoarchitecture for organic pollutants degradation: Structure dependence and mechanism, Chem. Eng. J., 2019, 360, 157–170 Search PubMed.
- W. Tian, H. Zhang, Z. Qian, T. Ouyang, H. Sun, J. Qin, M. O. Tadé and S. Wang, Bread-making synthesis of hierarchically Co@C nanoarchitecture in heteroatom doped porous carbons for oxidative degradation of emerging contaminants, Appl. Catal., B, 2018, 225, 76–83 Search PubMed.
- H. Sun, X. Peng, S. Zhang, S. Liu, Y. Xiong, S. Tian and J. Fang, Activation of peroxymonosulfate by nitrogen-functionalized sludge carbon for efficient degradation of organic pollutants in water, Bioresour. Technol., 2017, 241, 244251 Search PubMed.
|
This journal is © The Royal Society of Chemistry 2020 |
Click here to see how this site uses Cookies. View our privacy policy here.