Hydrophobicity or superhydrophobicity—which is the right choice for stabilizing underwater superoleophilicity?†
Received
6th September 2019
, Accepted 9th October 2019
First published on 10th October 2019
Abstract
Extremely water-repellent, bio-inspired superhydrophobic interfaces, which inherently display super-affinity for the oily phase underwater, provide a simple basis for the selective filtration of the bulk oil/oily phases from oil/water mixtures. However, the extreme water repellency in superhydrophobic interfaces appears as “Achilles' heel” for the separation of a practically more relevant and complex form of an oil/water mixture, which is an oil-in-water emulsion, as the suspended oil droplets in bulk aqueous phase are inaccessible to the selectively oil-absorbent superhydrophobic interface. Moreover, such underwater super oil-affinity inherently embedded in the superhydrophobic coating is known to be completely compromised over continuous exposure to either the aqueous phase for less than 2 days or at elevated temperatures (>50 °C) due to the spontaneous displacement of metastable-trapped air from the superhydrophobic interface. In this current report, a moderately hydrophobic (water contact angle ≤130°) multilayer coating, which inherently allows the coexistence of discontinuous trapped air and the aqueous phase, unusually displayed both underwater superoleophilicity and under-oil superhydrophobicity. The stability of underwater superoleophilicity in such hydrophobic multilayers was chemically tailored through a facile reaction between acrylate and selected alkylamines at ambient conditions, and the duration for a complete transition from superoleophilicity to superoleophobicity underwater significantly improved to 100 days in comparison to that of superhydrophobic multilayers (2 days). Moreover, this moderately hydrophobic interface, which displayed unusual and uninterrupted underwater superoleophobicity even at a highly elevated temperature (90 °C), was further explored in the successful separation of both bulk-oil spills and oil-in-water emulsions based on both the energy-efficient filtration and absorption principles; such dual-mode operations with a single interface are practically impossible to achieve with any existing biomimicked approaches. Thus, the performance of the moderately hydrophobic multilayers was superior to that of biomimicked superhydrophobic materials in mainly two important and relevant aspects, i.e., (a) unprecedented stability of underwater extreme oil-affinity and (b) ability for separating both oil-in-water emulsions and bulk oil/water mixtures in severe settings following energy-efficient and environmentally friendly separation processes.
Introduction
Nature-inspired superhydrophobic interfaces, which are formally defined as the surfaces with the ability of repelling a beaded aqueous phase extremely in air with an advancing water contact angle above 150° and contact angle hysteresis below 10°, are artificially synthesized by the appropriate co-optimization of both essential hierarchical topography and appropriate low surface energy chemistry.1–8 The metastable and continuous trapped air layer in the chemically modulated hierarchical topography is the primary basis for minimizing the contact between the beaded aqueous phase and the biomimicked superhydrophobic interfaces, which results in super water repellence with effortless rolling of the beaded water droplet on a slight tilting of these superhydrophobic interfaces.9–11 Such interfaces inherently possess another super liquid wettability, which is termed as underwater superoleophilicity, and the continuous trapped air layer is hypothesized to be the prime criterion for achieving such extreme oil affinity in superhydrophobic interfaces under water.12–14 Thus, the same biomimicked interface simultaneously displays extreme repellence for water and super-affinity for oil/oily phases underwater.12–14 These contrasting wettabilities towards two distinct liquids have been further successfully explored in the separation of the bulk oil phase from an oil/water mixture following either selective absorption or filtration principles.15–22 In addition to this, superhydrophobic interfaces with tailored adhesive properties are successfully extended for monitoring the underwater motion of an object.23 However, in superhydrophobic interfaces, the continuous layer of metastable trapped air extremely repels an oil-in-water emulsion, where the oil droplets are dispersed in a continuous aqueous phase. Thus, superhydrophobicity is inherently inappropriate for the separation of an oil-in-water emulsion, which is practically a more relevant and complex form of oil spillage that has severe and adverse impacts on the environment.23,24 In recent past, a superhydrophilic porous substrate was used strategically for the separation of oil-in-water emulsions;25 however, this principle is ineffective for the selective absorption-based removal of oil spillage from vast and open water reservoirs.
In 2012, Wang and co-workers investigated the transition of underwater superoleophilicity to superoleophobicity in superhydrophobic interfaces,13 where the impact of a continuous layer of metastable trapped air on the spreading and soaking of an oil phase underwater was examined. In an earlier demonstration, the embedded underwater superoleophilicity was compromised within a few hours and gradually switched to superoleophobicity over 48 hours.13 Furthermore, this continuous trapped air layer in the superhydrophobic interface is known to be highly labile above 50 °C,26 which makes such biomimicked interfaces inherently inappropriate to perform at high temperatures.26,27 Here, in this current report, a very unusual property of a moderately hydrophobic (referred to the interface that displayed water wettability with minimum and maximum contact angles of 120° and 130°, respectively) multilayer (Scheme 1B) was compared with that of a superhydrophobic multilayer coating (Scheme 1A). Unlike, the superhydrophobic interface, the hydrophobic multilayer could coexist with both ‘discontinuous’ trapped air and infiltrated aqueous phase when submerged underwater; however, such interfaces are highly efficient for displaying underwater superoleophilicity (Scheme 1D) similar to a superhydrophobic interface. Furthermore, the complete switching of two extreme oil wettabilities underwater for hydrophobic multilayers was significantly delayed to 100 days (Scheme 1F) in comparison to that of the superhydrophobic multilayers (2 days). Even at a highly elevated temperature (90 °C), the hydrophobic multilayers continued to display uninterrupted underwater superoleophilicity, which is extremely rare to achieve with superhydrophobic interfaces. In addition to this, the as-synthesized hydrophobic multilayer coatings were highly efficient (6500 wt%) in selective absorption-based bulk oil phase separation from the aqueous phase similar to the superhydrophobic coating. The same interface could be further extended for filtration-based bulk oil/water mixtures. Moreover, unlike superhydrophobic interfaces, this moderately hydrophobic multilayer coating, which was filled with discontinuous trapped air and inherently allowed the selective infiltration of the aqueous phase, was unprecedentedly extended for oil-in-water emulsion separations following the selective absorption principle even under challenging conditions, including extreme pH, temperatures, and salinity.
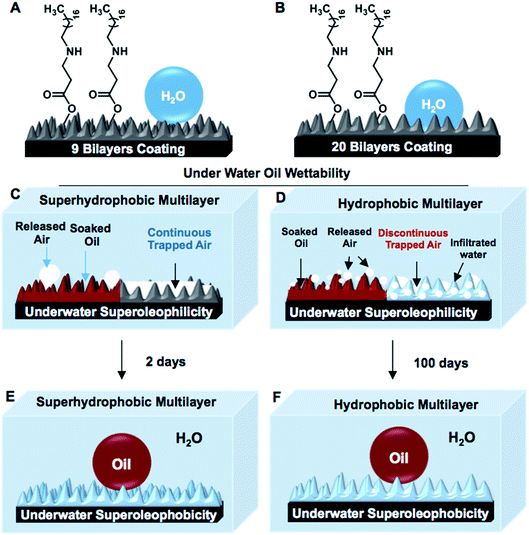 |
| Scheme 1 (A and B) Schematic illustration of two distinct octadecylamine (ODA)-modified multilayers that displayed superhydrophobicity ((A) 9 bilayers constructed in the presence of sodium chloride (NaCl) salt) and hydrophobicity ((B) 20 bilayered coating fabricated in the absence of salt). (C and D) The underwater oil wettability on both the superhydrophobic ((C) having continuous trapped air) and hydrophobic ((D) having discontinuous trapped air) multilayers. (E and F) The complete and extreme switching of underwater oil wettability (from superoleophilic to superoleophobic) in superhydrophobic (E) and hydrophobic (F) multilayers after continuous submersion of the respective multilayers for 2 days and 100 days, respectively. | |
Results and discussions
Comparative underwater oil wettability study on superhydrophobic and hydrophobic multilayers
The synthesis of superhydrophobic coatings on geometrically complex objects is challenging, and there are few reports of superhydrophobic coatings on geometrically complex objects.28,29 Recently, a catalyst-free and facile 1,4-conjugate addition reaction between amine and acrylate groups at ambient conditions was explored for synthesizing biodegradable polymers, amplification of desired functional groups and various functional interfaces.30–33 This simple and robust chemistry was extended for building chemically reactive multilayer coatings of a reactive-nanocomplex prepared by reacting branched polyethylenimine (BPEI) and dipentaerythritol penta-acrylate (5Acl) through 1,4-conjugate addition reaction in both the presence and the absence of sodium chloride (NaCl, 0.5 mg ml−1) salt.34 In the presence of the salt (in methanol), the growth of the multilayer (9 bilayers, where the deposition of BPEI followed by the nanocomplex of 5Acl/BPEI is referred to as a single bilayer) coating was highly accelerated and provided the essential hierarchical topography to display superhydrophobicity, as shown in Fig. 1A, after the post-covalent modification of the multilayer with selected alkylamine (i.e., octadecylamine (ODA)).34 However, a chemically reactive multilayer (9 bilayers) prepared in absence of the salt was found to be only hydrophobic with a water contact angle of 104° after identical post-covalent modification.
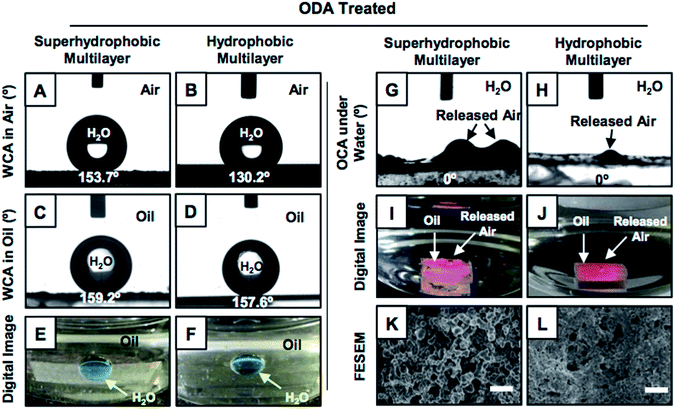 |
| Fig. 1 (A–F) Contact angles (A–D) and digital images of (E and F) beaded water (A–F) droplets on both superhydrophobic (A, C and E) and hydrophobic (B, D and F) multilayers in air (A and B) and under oil (C–F), respectively. (G–J) Contact angle images (G and H) and digital (I and J) images depicting the soaking of beaded oil on both superhydrophobic (G and I) and hydrophobic (H and J) multilayers followed by the release of continuous and discontinuous trapped air, respectively, under water. (K and L) FESEM images of the superhydrophobic (K) and hydrophobic (L) multilayers (scale bar 6 μm). | |
This hydrophobicity in the multilayer (constructed in the absence of a salt) was further improved by increasing the layer-by-layer dipping cycles from 9 to 20 times (in ethanol). The synthesized multilayer coating (20 bilayered) displayed moderate hydrophobicity with a water contact angle (WCA) of 130° after post-covalent modification with the same alkylamine (i.e., ODA; Fig. 1B). Interestingly, the moderately hydrophobic multilayer displayed extreme water repellency under oil with WCA of 157° (Fig. 1D and F) similar to the superhydrophobic multilayer (Fig. 1C and E), which was likely due to the appropriate confinement of an external oil phase in both the hydrophobic and superhydrophobic multilayers. The post-chemical modification of the multilayer coatings that were prepared in both presence (9 bilayers) and absence (20 bilayers) of the salt were characterized with standard and widely accepted Fourier transform infrared spectroscopy (FTIR) analysis; here, a significant depletion of the IR peak intensity at 1409 cm−1 (corresponding to the C–H deformation of the β-carbon of vinyl group) with respect to the IR peak intensity for the carbonyl stretching at 1739 cm−1, after treatment with selected alkylamine (ODA; Fig. S1K and L†), revealed the successful post-covalent reaction between the primary amines of ODA and the residual acrylate groups present in the synthesized multilayers.34 Furthermore, the experiment was designed to examine the relation between the water wettability (in air) and oil wettability (underwater) of the multilayer coatings. The multilayer (20 bilayers) coatings were individually post-modified with different alkylamines (i.e., propylamine, butylamine, pentylamine, hexylamine, heptylamine, octylamine, decylamine, dodecylamine and octadecylamine) through the 1,4-conjugate addition reaction for tailoring the water wettability (in air) over a wide range (starting from 71° (propylamine-modified) to 130° (ODA-modified)). A variation of the underwater oil wettability was noted for the multilayers having different water wettabilities, as shown in Fig. S2.† The multilayers having water contact angle ≥120° displayed underwater superoleophilicity with an oil contact angle of 0°, as shown in Fig. S2.†
A very similar result was obtained for the superhydrophobic multilayers, where the oily phase beaded with the oil contact angle (OCA) of 0°, as shown in Fig. 1G and I. At the end, both the continuous and discontinuous trapped meta-stable air layers, which were located in the superhydrophobic and hydrophobic interfaces, respectively, were released from the respective multilayer coatings (Fig. 1I, J, S3 and S4†). The release of the continuous trapped air from the superhydrophobic interfaces was observed to be faster compared to that for the hydrophobic interfaces that were packed with discontinuous trapped air, which is evident from Fig. 2A, S4 and Movies 1–6.† For the further investigation of this fact, FESEM imaging of both the multilayers was carried out; it confirmed the existence of completely distinct topography in both the superhydrophobic and hydrophobic multilayers (Fig. S5†) even though both multilayers (20 bilayers and 9 bilayers) were post-modified following the same chemistry (i.e., ODA, Fig. 1K and L). Furthermore, atomic force microscopic (AFM) imaging (Fig. S6†) was conducted to estimate the roughnesses in both the hydrophobic and superhydrophobic multilayers; the values were calculated to be 423.5 ± 15.7 nm and 735.2 ± 20.8 nm, respectively. This change in the roughness for the hydrophobic and superhydrophobic multilayers has a significant impact on minimizing the contact area between the beaded aqueous phase and the respective multilayer coatings. The fraction of contact area was measured following the Cassie–Baxter equation (eqn (1)),1–3,35 where θ and θr are the static water contact angles on the ODA-treated smooth (80.7°, 2 bilayered coatings) and rough hydrophobic (130.2°) and superhydrophobic (153.7°) multilayer coatings.
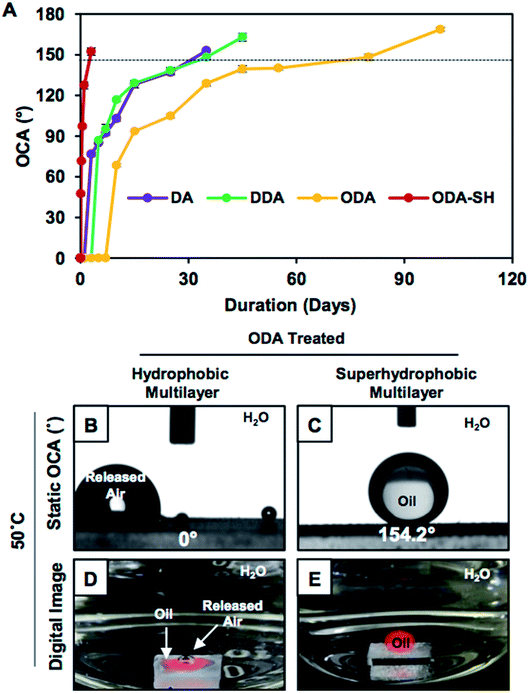 |
| Fig. 2 (A) The plot accounting the change in underwater oil wettability with time (days) for both the hydrophobic multilayers that are post-modified with decylamine (DA, purple), dodecylamine (DDA, green), octadecylamine (ODA, yellow) and the superhydrophobic multilayers (red, denoted as ODA-SH). (B–E) Underwater oil contact angles (B and C) and digital (D and E) images of the beaded oil droplets on hydrophobic ((B and D) modified with ODA) and superhydrophobic (C and E) multilayers at 50 °C; the hydrophobic multilayer readily soaked oil and released trapped air; however, the superhydrophobic multilayer failed to soak oil and rather extremely repelled beaded oil droplet with contact angle 154° at 50 °C. | |
The fractions of contact area for the beaded water droplet with both multilayer coatings (hydrophobic and superhydrophobic) and the trapped air were denoted as f1 and f2, respectively, and the total fraction of contact area (f1 + f2) between the beaded water droplet with multilayer coatings and trapped metastable air was considered to be 1.35
Smooth and featureless multilayers were built from the same chemical ingredient following a previously reported procedure.34 The dominant hierarchical roughness in the superhydrophobic multilayer allowed the trapping of a continuous layer of metastable air, which minimized the fraction of contact area between the aqueous phase and the multilayer coating to 0.089. In contrast, the hydrophobic multilayers that were infused with discontinuous trapped air allowed the beading of a liquid water droplet with a higher fraction (0.305) of contact area than that of the superhydrophobic coating. However, at the same time, the hydrophobic multilayer displayed underwater superoleophilicity very similar to that of the superhydrophobic interface (Fig. 1G–J). In fact, the stability of the underwater superoleophilicity in the hydrophobic multilayers was noticed to be exceptionally superior over that of the superhydrophobic interface, as clearly evidenced in Fig. S7 and S8.† The inherent extreme oil affinity underwater in the superhydrophobic multilayers was noticed to be compromised within a few hours and resulted in altering the superoleophilicity to superoleophobicity of the same multilayer underwater after continuous exposure to the aqueous phase for 2 days, as shown in Fig. 2A and S8.† In contrast, moderately hydrophobic multilayers, which were optimized with discontinuous trapped air, remained as underwater superoleophilic with OCA of 0° even after submerging the interface for consecutive 7 days. Moreover, in case of the hydrophobic multilayers, the duration for a complete transition between the two extreme oil wettabilities from superoleophilicity to superoleophobicity was significantly delayed to 100 days (Fig. 2A), which was remarkably higher than that for the superhydrophobic interface (2 days). However, the hydrophobic multilayers, which were post-chemically optimized with the lower analogues (e.g., DDA and DA) of ODA, displayed less stability of underwater superoleophilicity with respect to the ODA-treated hydrophobic interface, as noted in Fig. 2A, and the release of trapped air was also slower for such multilayers, as noted in Fig. S3.† Thus, both the chemistry and topography in multilayer coatings played a crucial role in modulating the stability of underwater superoleophilicity. Furthermore, the continuous trapped air layer in the superhydrophobic interfaces is known to be labile at elevated temperatures and eventually, the superhydrophobic multilayer readily and completely failed to display underwater superoleophilicity at 50 °C, rather displayed underwater superoleophobicity as shown in Fig. 2C and E. In contrast, the ODA-treated hydrophobic multilayers remained superoleophilic at 50 °C, as shown in Fig. 2B and D, and this extreme oil affinity of the hydrophobic multilayers remained unperturbed even at 90 °C, as illustrated in Fig. S9.† The exact reason for this superior thermal stability of hydrophobic multilayers has yet to be revealed experimentally. The discontinuous trapped air layer in the hydrophobic multilayers played a crucial role in such superior thermal stability. In the past, experiments were designed to reveal the reason behind the failure of superhydrophobicity at high temperatures.36 It was experimentally validated that superhydrophobicity was readily compromised when the temperature of the superhydrophobic surface was lower than that of the beaded aqueous phase due to the condensation process at the interface.36 In general, at high temperatures, the thermally insulating continuous trapped air layer in the superhydrophobic coating was expected to keep the superhydrophobic surface cooler than the aqueous phase as the continuous metastable trapped air prevented the transport of heat across the solid/liquid interface. Eventually, the superhydrophobic multilayer coatings failed to perform at high temperatures. In contrast, in the hydrophobic multilayer coatings, the discontinuous trapped air layer allowed the infiltration of the aqueous phase into the hydrophobic coating, as evident from Fig. 3A–D. Thus, the heat transported across the interface of the hydrophobic coating and the aqueous phase remained continuous, and this process was likely to prevent the difference in temperatures at the solid/liquid interface for the hydrophobic coating. Eventually, the hydrophobic coatings continued to perform at high temperatures in reality. Thus, the hydrophobic multilayer was inherently endowed with superior stability of embedded superoleophilicity underwater even at elevated temperatures in comparison to the superhydrophobic multilayer (Fig. S9 and S10†).
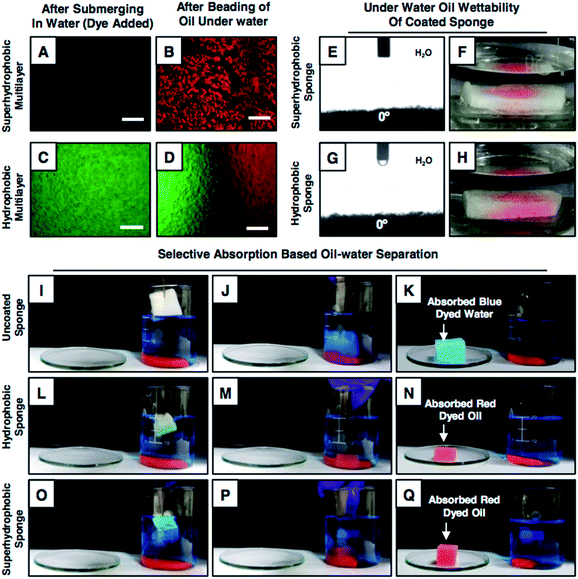 |
| Fig. 3 (A–D) Fluorescence microscopic images (scale bar 200 μm) of superhydrophobic (A and B) and hydrophobic (C and D) multilayers after submerging in fluorescein dye-added aqueous phase (A and C) followed by underwater exposure to Nile red-added silicone oil (B and D). (E–H) Contact angles (E and G) and digital (F and H) photographs depicting beading of oil (red colour aids visual inspection) underwater on both superhydrophobic (E and F) and hydrophobic (G and H) multilayer-coated melamine sponge. (I–Q) Digital images comparing the ability for collecting the sedimented bulk model-oil (dyed with Nile red) under water (dyed with methylene blue) by uncoated (I–K), hydrophobic (L–N) and superhydrophobic (O–Q) multilayer-coated spongy materials. | |
Investigation on selective underwater oil affinity for hydrophobic and superhydrophobic multilayers
Furthermore, experiments were designed to investigate the selective affinity of both the superhydrophobic and hydrophobic multilayer coatings towards both the oil/oily phase and aqueous phase underwater, where the as-synthesized superhydrophobic and hydrophobic multilayers were submerged in a dye (fluorescein dye with green fluorescence, water-soluble)-added aqueous phase. In the case of the hydrophobic multilayer, the aqueous phase immediately infiltrated through the porous multilayer coating, as evident from the appearance of the green fluorescence signal in Fig. 3C. However, no fluorescence signal was observed for the superhydrophobic coating as the continuous layer of trapped air in the superhydrophobic multilayer restricted the infiltration of the aqueous phase inside the coating (Fig. 3A). After this aqueous phase treatment, immediately, both the hydrophobic and superhydrophobic multilayer coatings were exposed to the dye (Nile red provides a red fluorescence signal)-contaminated oil phase underwater. The dye-added oil phase was immediately soaked by both the multilayers, as evident from the red fluorescence signal for the polymeric coatings in Fig. 3B, D and S11.† Interestingly, the infiltrated aqueous phase in the hydrophobic multilayer was completely replaced by the oil phase underwater, which was likely due to the existence of discontinuous trapped air. Inspired from this earlier result, a highly porous and commercially available substrate that is melamine sponge was decorated individually with moderately hydrophobic (20 bilayers in the absence of salt; ODA-treated) and superhydrophobic multilayers (9 bilayers in the presence of salt; ODA-treated) (Fig. S12†). At the end, both the hydrophobic and superhydrophobic melamine sponges displayed underwater superoleophilicity with an oil-contact angle of 0°, as shown in Fig. 3 E–H, and these sponges remained equally efficient (6500 wt%) for the selective absorption of the oil phase, as shown in Fig. 3I–Q, Movies 7 and 8.† Moreover, the hydrophobic sponge could be reversibly deformed with 60% compressive strain; however, the embedded underwater superoleophilicity remained unperturbed, as demonstrated in Fig. S13.† In addition to this, the embedded under oil superhydrophobicity (Fig. 1F) of the hydrophobic multilayer coating was extended for the selective filtration of bulk oils (both light and heavy) from oil/water mixtures following the gravity-driven filtration process, where the hydrophobic coating was pre-wetted with the oil phase before exposing to the practically relevant three-phase oil/water mixture that consisted of a light oil (kerosene, blue), aqueous phase (yellow) and heavy oil (dichloromethane, pink). The successful and selective passage of the oil phases was confirmed with the experimental demonstrations in Fig. S14.†
Ability for oil-in-water emulsion separation
Next, the performances of the hydrophobic and superhydrophobic spongy substrates in separating oil-in-water emulsions were compared in detail. In general, the extrusion pressure is larger for superhydrophobic interfaces due to the presence of continuous meta-stable trapped air that extremely repels the aqueous phase. Eventually, the oil-in-water emulsion consisting of tiny oil droplets with the diameter of hundreds/thousands of nanometers, dispersed in the bulk water phase, was unable to pass through the superhydrophobic coating under the gravitational force, as shown in Fig. 4A and B. Eventually, no oil/water separation was observed (Fig. 4A and B). However, the hydrophobic interface having a lower extrusion pressure readily allowed the passage of the aqueous oil-in-water emulsion and the embedded underwater superoleophilicity in the hydrophobic multilayer coating readily and selectively absorbed the oil droplets (Fig. 3C and D). Eventually, this low extrusion pressure in the hydrophobic coating helped in separating the oil-in-water emulsions under the gravitational force. The hydrophobic spongy substrate (having pore size of 145.0 ± 12.8 μm and porosity of 98.5 ± 0.5%), which displayed underwater superoleophilicity, was observed to be capable of separating oil-in-water milky emulsions (5 (v/v)% 1,2-dichloroethane (DCE)) with water collection/separation efficiency above 95%; this was estimated following the eqn (S1) provided in the ESI.† Under the gravitational force, the milky oil-in-water emulsion phase passed through the selected porous material, and the micro oil droplets that were dispersed in the bulk aqueous phase of the oil-in-water emulsion were immediately and selectively separated by the hydrophobic surface; this performance was continued till the material reach its maximum oil absorption capacity (6500 wt%). Eventually, a clear aqueous phase was selectively filtrated (Fig. 4C–E and Movie 9†) and collected in a separate conical flask/beaker. The successful separation of oil contamination was further characterized by optical microscopy images and DLS studies. Moreover, no peak for carbon was noticed in the 13C nuclear magnetic resonance (NMR) spectra of the filtrate after performing the oil-in-water emulsion (5 (v/v)% DCE) separation using the hydrophobic multilayer-coated sponge, as shown in Fig. S15.† The synthesized hydrophobic surface with high selective oil absorption capacity (6500 wt%) was appropriate for the continuous and long-term separation of oil-in-water emulsions (where the content of the oil was below 10 v%).
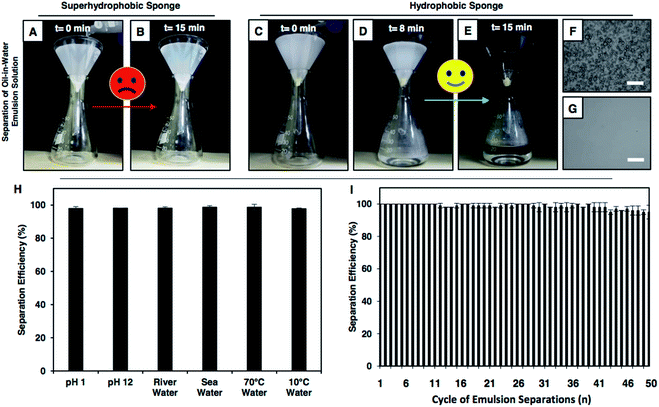 |
| Fig. 4 (A–E) Comparing the performances of oil-in-water emulsion (5% (v/v) DCE in water) separation with superhydrophobic (A and B) and hydrophobic (C–E) melamine sponges. (F and G) Bright field microscopic images of emulsion solution before (F) and after (G) filtration through hydrophobic sponge; scale bar: 100 μm. (H) Separation efficiency of 5% of oil-in-water emulsions under diverse chemically complex scenarios like pH 1, pH 12, river water, artificial sea water, 70 °C water and 10 °C water. (I) Separation efficiency of oil-in-water emulsion for consecutive 50 times. | |
This oil-in-water emulsion separation was also successfully performed using various other oils and water-immiscible organic solvents including diesel, petrol, chloroform, and toluene, and the separation efficiency remained around 95%, as shown in Table S1.† In contrast, the intrusion of the oil-in-water emulsion through the superhydrophobic sponge was fundamentally and practically challenging to achieve due to the extreme repulsion of the bulk aqueous phase and as expected, the passage of the oil-in-water emulsion was highly restricted, as shown in Fig. 4A, B and Movie 10.† Thus, the demonstration of the gravity-driven selective filtration of the oil-in-water emulsion through superhydrophobic interfaces was not reported in the literature, as shown in Table 1. Moreover, the performance of oil/water separation by the biomimicked superhydrophobic interfaces at an elevated temperature was not known, as documented in Table 1. In comparison, only the hydrophobic multilayer-coated spongy substrate was superior over the superhydrophobic material as the hydrophobic multilayers that were embedded with super oil affinity underwater were unprecedentedly efficient for separating both oil-in-water emulsions and bulk oil/water mixtures following both the selective filtration and absorption processes, as noted in Table 1. Furthermore, this oil/water emulsion separation process was expedited by the application of vacuum, and the separation was successfully carried out with a high flux rate of 62
608.69 m h−1 MPa−1. Also, this oil/water separation was performed under various practically relevant challenging settings including extreme pH, seawater, river water, and extreme temperatures (Fig. S16–S18†). The hydrophobic multilayer coating could survive continuous exposure to elevated temperatures, extreme pH and artificial seawater and river water for minimum of 7 days without compromising the underwater superoleophilicity. Unlike the superhydrophobic interface, the as-synthesized hydrophobic multilayer coating remained very efficient to separate an oil-in-water emulsion repetitively at challenging settings, as shown in Fig. 4H and I. Thus, the hydrophobic multilayers were capable of cleaning up different forms of oil spillages including oil-in-water emulsions and bulk oil/water mixtures following both the selective absorption and filtration processes; a report of this demonstration is unprecedented in the literature.
Table 1 A comparative oil/water separation performance by biomimicked superhydrophobic interfaces37–54 and current hydrophobic multilayer coating. Current hydrophobic multilayers were highly efficient in separating both oil-in-water emulsion and bulk oil/water mixtures following both energy-efficient selective absorption and filtration processes at elevated temperatures (above 70 °C), whereas superhydrophobic interfaces were inherently inefficient for filtration-based oil-in-water separation; moreover, such interfaces were incapable of performing at elevated temperatures
Coatings/materials |
Description of the coatings/materials |
Absorption-based bulk oil/water separation |
Filtration-based bulk oil/water separation |
Filtration-based oil-in-water emulsion separation |
Oil-water separation at elevated temperature (70 °C) |
References |
Superhydrophobic |
Coating of branched polydimethylsiloxane (PDMS) brush |
Yes |
No |
No |
No |
37
|
Superhydrophobic |
Sol–gel coating of PDMS-based copolymers |
Yes |
No |
No |
No |
38
|
Superhydrophobic |
Perfluorodecyltriethoxysilane-modified coating of hydroxyapatite nanowires with zinc oxide |
Yes |
No |
No |
No |
39
|
Superhydrophobic |
Crystallized coating of ultrahigh molecular weight polyethylene |
Yes |
No |
No |
No |
40
|
Superhydrophobic |
Electrospinning coating of polysulfone and poly(glycerol sebacate) |
Yes |
No |
No |
No |
41
|
Superhydrophobic |
Composite coating of fluorinated graphene oxide and zeolite imidazole framework |
Yes |
No |
No |
No |
42
|
Superhydrophobic |
Coating based on graphene monoliths |
Yes |
No |
No |
No |
43
|
Superhydrophobic |
Coating based on surface-modified nanoclay |
No |
Yes |
No |
No |
44
|
Superhydrophobic |
Coating with multiscale hierarchical dendritic structure on steel mesh |
No |
Yes |
No |
No |
45
|
Superhydrophobic |
Coating of hydrophobic nanosilica-filled PDMS |
No |
Yes |
No |
No |
46
|
Superhydrophobic |
Electrochemical polymerization followed by acrylation |
No |
Yes |
No |
No |
47
|
Superhydrophobic |
Hybrid coating of candle soot and silica nanoparticles |
No |
Yes |
No |
No |
48
|
Superhydrophobic |
Scratched and pricked polyethylene (PE) film |
Yes |
Yes |
No |
No |
49
|
Superhydrophobic |
Layer-by-layer coating of poly(2-vinyl-4,4-dimethylazlactone) and poly(ethylene imine) |
Yes |
Yes |
No |
No |
50
|
Superhydrophobic |
Condensation polymerization of cctadecylamine, melamine and formaldehyde |
Yes |
Yes |
No |
No |
51
|
Superhydrophobic |
Hybrid aerogel coating of silk fibroin proteins and organically substituted alkoxysilanes |
Yes |
Yes |
No |
No |
52
|
Superhydrophobic |
Porous composite coating of poly(vinylidene fluoride) (PVDF) and multiwalled carbon nanotube (MWCNT) |
Yes |
Yes |
No |
No |
53
|
Superhydrophobic |
Reduced graphene oxide (rGO)-based coating |
Yes |
Yes |
No |
No |
54
|
Hydrophobic
|
Alkylamine-modified chemically reactive multilayers coating
|
Yes
|
Yes
|
Yes
|
Yes
|
Current work
|
Conclusions
In summary, the synthesized moderately hydrophobic multilayers, comprising discontinuous trapped air, possessed superior stability of superoleophilicity properties underwater over the superhydrophobic interfaces irrespective of whether under a prolonged and continuous exposure to the aqueous phase or elevated temperatures. A simple chemical approach was unprecedentedly explored in stabilizing underwater superoleophobicity by the appropriate tailoring of topography as well as chemistry. Furthermore, the hydrophobic multilayer coatings, optimized with appropriate alkylamine, remained highly capable of separating both bulk and emulsified oil/oily phases from the aqueous phase following the selective absorption process and the energy-efficient filtration process, respectively. In comparison, the superhydrophobic coating was fundamentally inappropriate for separating an oil-in-water emulsion following the filtration process. In addition to this, covalently cross-linked, hydrophobic multilayer coatings were able to performed oil-in-water emulsion separation at diverse and practically relevant severe settings. Thus, such an unprecedented principle for optimizing durable underwater superoleophilicity would be of potential interest for various fundamental and application-oriented studies.
Conflicts of interest
There are no conflicts to declare.
Acknowledgements
Science and Engineering Research Board (YSS/2015/000818), and Board of Research in Nuclear Sciences (BRNS) (34/20/31/2016-BRNS, DAE-YSRA), the Ministry of Electronics and Information Technology (grant no. 5(9)/2012-NANO) and Ministry of Human Resource Development (F. No. 22-03/2016-TS.II/TC) are acknowledged for their financial support. We are thankful to CIF and the Department of Chemistry, Center for Nanotechnology, Indian Institute of Technology-Guwahati. Ms Dibyangana Parbat and Mr Kousik Maji are grateful to IIT-Guwahati for their Ph.D. fellowships from the Institute and Mr Avijit Das thanks CSIR for his SRF fellowship support.
References
- X. J. Feng and L. Jiang, Adv. Mater., 2006, 18, 3063 CrossRef CAS.
- X. M. Li, D. Reinhoudt and M. Crego-Calama, Chem. Soc. Rev., 2007, 36, 1350 RSC.
- Y. Y. Yan, N. Gao and W. Barthlott, Adv. Colloid Interface Sci., 2011, 169, 80 CrossRef CAS PubMed.
- S. Wang, K. Liu, X. Yao and L. Jiang, Chem. Rev., 2015, 115, 8230 CrossRef CAS PubMed.
- B. Su, Y. Tian and L. Jiang, J. Am. Chem. Soc., 2016, 138, 1727 CrossRef CAS PubMed.
- Y. Li, X. J. Huang, S. H. Heo, C. C. Li, Y. K. Choi, W. P. Cai and S. O Cho, Langmuir, 2007, 23, 2169 CrossRef CAS PubMed.
- Y. Li, T. Sasaki, Y. Shimizu and N. Koshizaki, J. Am. Chem. Soc., 2008, 130, 14755 CrossRef CAS.
- C. Peng, Z. Chen and M. K. Tiwari, Nat. Mater., 2018, 17, 355 CrossRef CAS.
- A. B. D. Cassie and S. Baxter, Trans. Faraday Soc., 1944, 40, 546 RSC.
- A. B. D. Cassie and S. Baxter, Nature, 1945, 155, 21 CrossRef CAS.
- W. Barthlott and C. Neinhuis, Planta, 1997, 202, 1 CrossRef CAS.
- L. Feng, Z. Zhang, Z. Mai, Y. Ma, B. Liu, L. Jiang and D. Zhu, Angew. Chem., Int. Ed., 2004, 43, 2012 CrossRef CAS PubMed.
- M. Jin, S. Li, J. Wang, Z. Xue, M. Liao and S. Wang, Chem. Commun., 2012, 48, 11745 RSC.
- M. Hirtz, A. Oikonomou, T. Georgiou, H. Fuchs and A. Vijayaraghavan, Nat. Commun., 2013, 4, 2591 CrossRef.
- J. Yong, J. Huo, F. Chen, Q. Yang and X. Houa, Phys. Chem. Chem. Phys., 2018, 20, 25140 RSC.
- J. Yuan, X. Liu, O. Akbulut, J. Hu, S. L. Suib, J. Kong and F. Stelacci, Nat. Nanotechnol., 2008, 3, 332 CrossRef CAS PubMed.
- J. Zhang and S. Seeger, Adv. Funct. Mater., 2011, 21, 4699 CrossRef CAS.
- C. R. Crick, J. A. Gibbinsa and I. P. Parkin, J. Mater. Chem. A, 2013, 1, 5943 RSC.
- Z. Chu, Y. Feng and S. Seeger, Angew. Chem., Int. Ed., 2015, 54, 2328 CrossRef CAS.
- J. Ge, H. Y. Zhao, H. W. Zhu, J. Huang, L. A. Shi and S. H. Yu, Adv. Mater., 2016, 28, 10459 CrossRef CAS.
- J. Gu, H. Fan, C. Li, J. Caro and H. Meng, Angew. Chem., Int. Ed., 2019, 58, 5297 CrossRef CAS PubMed.
- J. Jin, X. Zhao, Y.-H. Du, M. Ding, C. Xiang, N. Yan, C. Jia, Z. Han and L. Sun, iScience, 2018, 6, 289 CrossRef CAS PubMed.
- C. H. Peterson, S. D. Rice, J. W. Short, D. Esler, J. L. Bodkin, B. E. Ballachey and D. B. Irons, Science, 2003, 302, 2082 CrossRef CAS PubMed.
- C. P. D. Brussaard, L. Peperzak, S. Beggah, L. Y. Wick, B. Wuerz, J. Weber, J. S. Arey, B. Burg, A. Jonas, J. Huisman and J. R. Meer, Nat. Commun., 2016, 7, 11206 CrossRef CAS PubMed.
- G. Ding, W. Jiao, R. Wang, M. Yan, Z. Chu and X. He, J. Mater. Chem. A, 2019, 7, 17766 RSC.
- Y. Liu, X. Chen and J. H. Xin, J. Mater. Chem., 2009, 19, 5602 RSC.
- P. Papadopoulos, L. Mammen, X. Deng, D. Vollmer and H.-J. Butt, Proc. Natl. Acad. Sci. U. S. A., 2013, 110, 3254 CrossRef CAS PubMed.
- C. Xiang and L. Sun, J. Phys. Chem. C, 2018, 122, 29210 CrossRef CAS.
- C. Xiang, L. Sun, Y. Wang, G. Wang, X. Zhao and S. Zhang, J. Phys. Chem. C, 2017, 121, 15448 CrossRef CAS.
- D. M. Lynn and R. Langer, J. Am. Chem. Soc., 2000, 122, 10761 CrossRef CAS.
- M. R. Weatherspoon, M. B. Dickerson, G. Wang, Y. Cai, S. Shian, S. C. Jones, S. R. Marder and K. H. Sandhage, Angew. Chem., Int. Ed., 2007, 46, 5724 CrossRef CAS PubMed.
- J. Ford, S. R. Marder and S. Yang, Chem. Mater., 2009, 21, 476 CrossRef CAS.
- S. L. Bechler and D. M. Lynn, Biomacromolecules, 2012, 13, 1523 CrossRef CAS.
- D. Parbat, S. Gaffar, A. M. Rather, A. Gupta and U. Manna, Chem. Sci., 2017, 8, 6542 RSC.
- S. Yang, S. Chen, Y. Tian, C. Feng and L. Chen, Chem. Mater., 2008, 20, 1233 CrossRef CAS.
- Z. -J. Yu, J. Yang, F. Wan, Q. Ge, L. -L. Yang, Z. -L. Ding, D. -Q. Yang, E. Sacherc and T. T. Isimjand, J. Mater. Chem. A, 2014, 2, 10639 RSC.
- Y. Liu, X. Wang and S. Feng, Adv. Funct. Mater., 2019, 29, 1902488 CrossRef.
- H. Guo, J. Yang, T. Xu, W. Zhao, J. Zhang, Y. Zhu, C. Wen, Q. Li, X. Sui and L. Zhang, ACS Appl. Mater. Interfaces, 2019, 11, 13704 CrossRef CAS PubMed.
- G. Wen and Z. Guo, J. Mater. Chem. A, 2018, 6, 7042 RSC.
- S. Sun, L. Zhu, X. Liu, L. Wu, K. Dai, C. Liu, C. Shen, X. Guo, G. Zheng and Z. Guo, ACS Sustainable Chem. Eng., 2018, 6, 9866–9875 CrossRef CAS.
- S. Lee, B. Kim, S.-H. Kim, E. Kim and J.-H. Jang, Adv. Funct. Mater., 2017, 27, 1702310 CrossRef.
- K. Jayaramulu, K. K. R. Datta, C. Rçsler, M. Petr, M. Otyepka, R. Zboril and R. A. Fischer, Angew. Chem., Int. Ed., 2016, 55, 1178 CrossRef CAS.
- L.-B. Lv, T.-L. Cui, B. Zhang, H.-H. Wang, X. H. Li and J.-S. Chen, Angew. Chem., Int. Ed., 2015, 54, 15165 CrossRef CAS.
- P. M. Gore and B. Kandasubramanian, J. Mater. Chem. A, 2018, 6, 7457 RSC.
- Z. Han, B. Li, Z. Mu, S. Niu, J. Zhang and L. Ren, Small, 2017, 13, 1701121 CrossRef PubMed.
- J. Lv, Z. Gong, Z. He, J. Yang, Y. Chen, C. Tang, Y. Liu, M. Fan and W.-M. Lau, J. Mater. Chem. A, 2017, 5, 12435 RSC.
- C. Xiao, L. Si, Y. Liu, G. Guan, D. Wu, Z. Wang and X. Hao, J. Mater. Chem. A, 2016, 4, 8080 RSC.
- J. Li, R. Kang, X. Tang, H. She, Y. Yang and F. Zha, Nanoscale, 2016, 8, 7638 RSC.
- T. Zhao, D. Zhang, C. Yu and L. Jiang, ACS Appl. Mater. Interfaces, 2016, 36, 24186–24191 CrossRef PubMed.
- M. J. Kratochvil, U. Manna and D. M. Lynn, Polym. Chem., 2017, 55, 3127–3136 CrossRef CAS.
- L. Kang, J. Li, J. Zeng, W. Gao, J. Xu, Z. Cheng, K. Chen and B. Wang, J. Mater. Chem. A, 2019, 7, 16447 RSC.
- H. Maleki, L. Whitmore and N. Hüsing, J. Mater. Chem. A, 2018, 6, 12598 RSC.
- F. Chen, Y. Lu, X. Liu, J. Song, G. He, M. K. Tiwari, C. J. Carmalt and I. P. Parkin, Adv. Funct. Mater., 2017, 27, 1702926 CrossRef.
- H. Zhu, D. Chen, W. An, N. Li, Q. Xu, H. Li, J. He and J. Lu, Small, 2015, 11, 5222 CrossRef CAS.
Footnote |
† Electronic supplementary information (ESI) available: Experimental procedures and Fig. S1–S18 illustrating characterizations of hydrophobic and superhydrophobic multilayers, comparing oil-wettability under water, morphology of hydrophobic/superhydrophobic multilayers, stability of underwater superoleophilicity, AFM characterizations, accounting selective affinity for oil phase under water, performance for selective removal of bulk and emulsified oil droplets by hydrophobic multilayers coating. See DOI: 10.1039/c9ta09877a |
|
This journal is © The Royal Society of Chemistry 2020 |
Click here to see how this site uses Cookies. View our privacy policy here.