DOI:
10.1039/D0AN01447H
(Paper)
Analyst, 2021,
146, 207-212
Fe–N/C single-atom nanozyme-based colorimetric sensor array for discriminating multiple biological antioxidants†
Received
21st July 2020
, Accepted 26th September 2020
First published on 28th September 2020
Abstract
Identifying the species and concentrations of antioxidants is really important because antioxidants play important roles in various biological processes and numerous diseases. Compared with an individual sensor detecting a single antioxidant with limited specificity, a sensor array could simultaneously identify various antioxidants, in which 3–5 types of nanomaterials with peroxidase-like activity are absolutely necessary. Herein, as a single-atom nanozyme, Fe–N/C with oxidase-mimicking activity was applied to construct a triple-channel colorimetric sensor array: (1) Fe–N/C catalytically oxidized three substrates 3,3′,5,5′-tetramethylbenzidine (TMB), 2,2′-azino-bis(3-ethylbenzthiazoline-6-sulfonic acid) (ABTS) and o-phenylenediamine (OPD) to produce blue oxidized TMB (oxTMB), green oxidized ABTS (oxABTS) and yellow oxidized OPD (oxOPD), respectively; (2) with oxTMB, oxABTS and oxOPD as three sensing channels, a colorimetric sensor array was constructed for simultaneously discriminating glutathione (GSH), L-cysteine (L-Cys), ascorbic acid (AA), uric acid (UA), and melatonin (MT), even quantifying concentrations (with GSH as a model analyst). The performance of the sensor array was validated through accurately identifying 15 blind samples containing GSH, L-Cys, AA, UA and MT in buffer solution and human serum samples, and also in binary and ternary mixtures. This work proved that fabricating a single nanozyme-based sensor array was a simplified and reliable strategy for simultaneously probing multiple antioxidants.
Introduction
Antioxidants in living organisms are essential substances that are involved in keeping the balance of oxidant and antioxidant systems through scavenging overproduced reactive oxygen species, thus preventing complex diseases, such as diabetes, stroke, Parkinson's disease, cardiovascular disease, inflammatory diseases, and even cancer.1,2 For example, biothiols (such as L-cysteine (L-Cys) and glutathione (GSH)) are the most widely distributed antioxidants in cells, maintaining intracellular redox activity and signal transduction, and are usually applied as biomarkers of various diseases (liver damage, Alzheimer's disease and cancer).3–5 Uric acid (UA) is another kind of rich antioxidant in human blood, responsible for over half of the total antioxidant capacity. A higher level of UA can lead to gout and Lesch–Nyhan syndrome.6 Ascorbic acid (AA) as a neuro regulatory factor is also a vital antioxidant, and AA deficiency will cause scurvy.7 Also, melatonin (MT) can delay aging and improve sleep quality, and a low concentration of MT can cause various cancers.8 Hence, distinguishing these antioxidants will not only develop reliable approaches for satisfying the requirement of medical diagnosis but also provide deep insight into the biochemical processes.
To date, various sensing strategies (such as liquid chromatography,9 mass spectrometry,10 electrochemistry,11etc.12) have been developed to detect and discriminate antioxidants. Recently, nanozyme-based sensors (nanomaterials with enzymatic activity) have been explored and applied for antioxidant detection, providing facile and low-cost approaches for detecting antioxidants.13–15 However, most of the nanozyme-based sensors could only detect a single antioxidant, and also their specificity is greatly limited. For example, peroxidase-like nanozymes could oxidize colorless 3,3′,5,5′-tetramethylbenzidine (TMB) to blue oxidized TMB (oxTMB), and oxTMB acted as a colorimetric probe for the detection of L-Cys or GSH with a “turn-off” sensing mode.16–22 Due to the similar reductive reactivity, such a sensing array could not identify L-Cys and GSH,23,24 even AA and UA.25 Subsequently, inspired by the response of gustatory and olfactory systems to taste and smell, cross-reactive sensor arrays (so-called artificial “noses/tongues”) have emerged as promising alternatives for simultaneous discrimination of multiple antioxidants.26–34 By generating unique identification patterns, a series of antioxidants were differentiated.35–37 It was observed that various sensing channels, such as gold nanoparticles, semiconductor nanocrystals, quantum dots and metal–organic framework nanozymes, were selected to construct the sensor arrays, in which 3–5 species are absolutely necessary.38–40 In order to further simplify the sensing processes, could a single nanozyme be applied to construct multiple response channels for simultaneously discriminating biological antioxidants? This remains a challenge.
In our previous study, Fe-TPP⊂rho-ZIF (Fe-TPP = tetraphenylporphyrin iron; rho-ZIF = zeolitic imidazolate skeleton with a rho topology) was synthesized, and further pyrolyzed to form atomically dispersed Fe–N4 immobilized on a carbon substrate (Fe–N/C).41 In this study, as a single-atom nanozyme, Fe–N/C with oxidase-mimicking activity was applied to construct a three-channel colorimetric sensor array for simultaneously discriminating five representative biological antioxidants: GSH, L-Cys, AA, UA, and MT. Fe–N/C could catalytically oxidize TMB, ABTS (2,2′-azino-bis(3-ethylbenzthiazoline-6-sulfonic acid)) and OPD (o-phenylenediamine) to produce blue oxTMB, green oxidized ABTS (oxABTS) and yellow oxidized OPD (oxOPD), respectively. With oxTMB, oxABTS and oxOPD as sensing probes, a three-channel colorimetric sensor array was constructed: the target antioxidants (GSH, L-Cys, AA, UA, and MT) could reduce oxTMB, oxABTS and oxOPD to TMB, ABTS and OPD to different extents, respectively, accompanied by various colorimetric responses. As fingerprints, such variations of colorimetric responses could be applied to discriminate GSH, L-Cys, AA, UA, and MT as low as 100 nM, even quantifying concentrations (with GSH as a model analyst). The performance of the sensor array was validated through accurately identifying 15 blind samples containing GSH, L-Cys, AA, UA and MT, even in human serum samples, and also in binary and ternary mixtures. This single nanozyme-based sensor array opens a new avenue for simplifying the architecture of sensor arrays.
Experimental section
Materials
Dimethyl sulfoxide (DMSO) and CH3CH2OH were purchased from Beijing Chemical Works. Dimethyl CH3COOH was purchased from Beijing Tongguang Fine Chemicals Company. CH3COONa, L-cysteine (L-Cys), ascorbic acid (AA), melatonin (MT), uric acid (UA), glutathione (GSH), 3,3′,5,5′-tetramethylbenzidine (TMB), 2,2′-azino-bis(3-ethylbenzthiazoline-6-sulfonic acid) (ABTS) and o-phenylenediamine (OPD) were purchased from Aladdin Industrial Corporation (Shanghai, China). Zirconia, 2-ethylimidazole, zinc oxide (ZnO), 5,5-dimethyl-1-pyridine-N-oxide (DMPO), (NH4)2SO4, tetraphenylporphyrin iron (Fe-TPP) and N,N-diethylformamide (DEF) were purchased from Beijing Chemical Reagent Factory (Beijing, China). Human serum was purchased from Aldrich. All chemicals were used as received without further purification. Deionized water (18.2 MΩ cm, Millipore) was used in all experiments.
Synthesis of Fe–N/C
Fe–N/C was prepared according to our previous procedures.41
Oxidase-like properties of Fe–N/C with TMB, ABTS and OPD as substrates
Typically, 100 μL of TMB (10 mM) in DMSO and 100 μL of the as-prepared Fe–N/C (1.0 mg mL−1) were successively injected into buffer solution (20 mM NaAc–HAc, pH = 4.0) at room temperature with the final volume of 1 mL. The oxidized product (oxTMB) was evaluated by UV-vis spectra with wavelength from 500 to 800 nm. Alternatively, N2 was used to partially remove dissolved O2 for 30 min, and further evaluated by UV-vis spectra. Similarly, ABTS and OPD were also used as substrates, and the oxidized products (oxABTS and oxOPD) were evaluated by UV-vis spectra with wavelength from 375 to 475 and 370 to 620 nm, respectively.
For steady-state kinetics, keeping CFe–N/C at 0.1 mg mL−1 and varying CTMB (from 0.02 mM to 1.0 mM) with a final volume of 1 mL, the initial oxidation reaction velocity of TMB was obtained by monitoring the absorbance at 652 nm for 1 min. Similarly, varying CABTS (from 0.02 mM to 1.0 mM) and COPD (from 0.1 mM to 5.0 mM) with a final volume of 1 mL, the initial oxidation reaction velocities of ABTS and OPD were obtained by monitoring the absorbance at 420 and 450 nm for 1 min. The kinetic parameters, Km and Vmax, were calculated using the Lineweaver–Burk plot (derived from the Michaelis–Menten equation), shown as 1/ν = Km/Vmax × 1/[S] + 1/Vmax, where ν is the initial reaction velocity, Km is the Michaelis constant, Vmax is the maximal initial reaction velocity, and [S] is the substrate concentration.
Construction of a three-channel sensor array for discriminating antioxidants
Three sensing probes (oxTMB, oxOPD and oxABTS) were applied to construct the sensor array for discriminating five antioxidants (L-Cys, AA, MT, UA and GSH). The procedures are as follows: 100 μL Fe–N/C suspension (1.0 mg mL−1) and 100 μL TMB (10 mM) in DMSO solution, 100 μL OPD (50 mM) in DMSO solution or 100 μL ABTS (10 mM) in buffer solution were successively injected into buffer solution (20 mM NaAc–HAc, pH = 4.0) with the final volume of 1 mL. After incubation for 10 min at room temperature, the supernatant was obtained by centrifugation, respectively. Subsequently, 100 μL of 0.5–10 μM antioxidants was added to the supernatant for 1 h. The UV–vis spectra were recorded, and the absorbance values at 652, 450, and 420 nm were employed for discriminating the five antioxidants. This process was repeated for five antioxidant analytes to obtain six replicates of each. Therefore, five kinds of antioxidants were tested against the three sensing elements. Each test produced a training-data matrix of 5 antioxidants × 3 arrays × 6 replicates. The data were subjected to Origin and linear discriminant analysis (LDA) in SYSTAT V13.0.
Results and discussion
Oxidase-like activity of Fe–N/C
According to the reported procedures,41 Fe-TPP⊂rho-ZIF (Fe-TPP = tetraphenylporphyrin iron; rho-ZIF = zeolitic imidazolate skeleton with a rho topology) was synthesized, and then pyrolyzed to form atomically dispersed Fe–N4 immobilized on a carbon substrate (Fe–N/C) (Fig. S1, ESI†): (1) encapsulating tetraphenylporphyrin iron (Fe-TPP) molecules into the large interior cages of the rho-ZIF (zeolitic imidazolate framework with the rho topology) forming a host–guest Fe-TPP⊂rho-ZIF precursor, (2) pyrolyzing the precursor to obtain an atomically dispersed Fe–N4 with single-atom Fe loading as high as 3.8 wt%. Herein, as a single-atom nanozyme, Fe–N/C was applied to catalytically oxidize TMB (3,3′,5,5′-tetramethylbenzidine), OPD (o-phenylenediamine) as well as ABTS (2,2′-azino-bis(3-ethylbenzthiazoline-6-sulfonic acid)), respectively (Fig. 1). With TMB as a substrate, 0.1 mg mL−1 Fe–N/C and 1 mM TMB did not exhibit absorption peaks in the range of 500–800 nm (Fig. 1A). In the presence of 0.1 mg mL−1 Fe–N/C, 1 mM colourless TMB turned deep blue at 10 min, and a characteristic absorption peak at 652 nm was clearly observed, corresponding to the oxidized TMB (oxTMB).42 On further increasing the reaction time, the absorption peak remained stable (Fig. S2A, ESI†). Alternatively, if the reaction solution was bubbled with N2 for 30 min to partially remove the dissolved O2, the absorption peak decreased significantly. The inset of Fig. 1A shows the corresponding solution photographs. The results demonstrated that Fe–N/C acted as an oxidase-mimicking nanozyme. Subsequently, OPD was also investigated, as shown in Fig. 1B. 5 mM colourless OPD did not exhibit absorption peaks in the range of 370–620 nm. In the presence of 0.1 mg mL−1 Fe–N/C, colourless OPD turned bright yellow, and a characteristic absorption peak at 450 nm reached maximum at 15 min and remained stable (Fig. S2B, ESI†), corresponding to the oxidized OPD (oxOPD).34 Similarly, the absorption peak at 450 nm obviously decreases upon dissolved O2 being partially removed by bubbled N2. The inset of Fig. 1B shows the corresponding solution photographs. Meanwhile, as shown in Fig. 1C, 0.1 mg mL−1 Fe–N/C could also catalytically oxidize ABTS and ABTS switched to green oxidized ABTS (oxABTS),43 showing an absorption peak at 420 nm maximized at 5 min (Fig. S2C, ESI†). The corresponding solution photographs are shown in the inset of Fig. 1C.
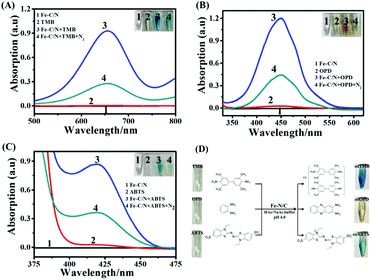 |
| Fig. 1 UV-vis absorbance spectra of (A) the TMB-Fe–N/C system, (B) the OPD-Fe–N/C system and (C) the ABTS-Fe–N/C system in buffer solution (20 mM NaAc–HAc, pH = 4.0); (D) Fe–N/C catalysed the oxidation of TMB, OPD and ABTS to produce oxTMB, oxOPD and oxABTS, respectively. | |
To further identify the reactive oxygen species (ROS) involved, electron spin resonance (ESR) spectroscopy was employed using 5,5-dimethyl-1-pyridine-N-oxide (DMPO) as a trapping agent (Fig. S3, ESI†). In the absence of Fe–N/C, no ESR signal was observed, while in the presence of Fe–C/N, remarkable characteristic peaks were detected, corresponding to the typical DMPO-O2˙− with an intensity ratio of 1
:
1
:
1
:
1, suggesting the generation of O2˙−.44 These results demonstrated that Fe–N/C could catalyze O2 to generate O2˙− in the Fe–C/N and TMB/OPD/ABTS system. Fig. 1D shows Fe–N/C catalytically oxidizing TMB, OPD and ABTS to blue oxTMB, yellow oxOPD and green oxABTS, respectively.
With TMB as a substrate, the concentration-, pH- and temperature-dependent catalytic activities were explored (Fig. S4, ESI†). At 25 °C, upon increasing Fe–N/C concentration (20 mM NaAc–HAc, pH = 4.0), the catalytic activity gradually increases. By keeping Fe–N/C at 0.1 mg mL−1 and varying CTMB from 0.02 mM to 1.0 mM, a typical Michaelis–Menten curve was obtained (Fig. S5A, ESI†). Based on these data, Michaelis constant (Km) and the maximum initial reaction rate (Vmax) were extracted: Km = 0.205 mM and Vmax = 541 nmol L−1 s−1 (Fig. S5B, ESI†). Compared with the reported oxidase mimics (Table S1, ESI†), Fe–N/C exhibited a smaller Km, indicating a relatively strong affinity between Fe–N/C and TMB. Similarly, for OPD (Fig. S6A and S6B, ESI†), the obtained Km was 0.676 mM and Vmax was 1.06 μmol L−1 s−1; for ABTS (Fig. S7A and S7B, ESI†), the obtained Km was 0.118 mM and Vmax was 344 nmol L−1 s−1 (Table S2, ESI†). These results showed that Fe–N/C exhibited different catalytic activities towards TMB, OPD and ABTS.
Construction of Fe–C/N-based sensor arrays for antioxidant detection
After Fe–N/C catalytically oxidized colourless TMB to blue oxTMB, Fe–N/C was further centrifuged. With oxTMB as a probe, a colorimetric sensing strategy could be designed to detect antioxidants, such as L-cysteine (L-Cys), glutathione (GSH), uric acid (UA), ascorbic acid (AA) and melatonin (MT), because these antioxidants could reduce blue oxTMB to colourless TMB, accompanied by the decreased absorption peak at 652 nm (Fig. S8A, ESI†). The parameter A/A0 (A and A0 were corresponding to the absorption of oxTMB in the presence and absence of the target antioxidant) could be applied as a readout signal for sensing the target antioxidant. Similarly, with oxOPD or oxABTS as a colorimetric probe, the five target antioxidants could also be detected (Fig. S8B and S8C, ESI†). However, as shown in Fig. 2, due to the lack of specificity, such a single probe could not simultaneously identify the five antioxidants.
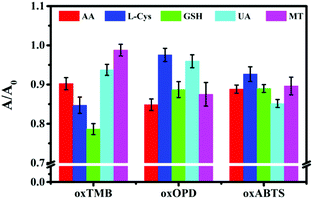 |
| Fig. 2 Colorimetric sensor assays with oxTMB, oxOPD or oxABTS as sensing probes for detecting 100 nM AA, L-Cys, GSH, UA and MT. Error bars represent the standard deviations from three replicates. | |
In order to clearly discriminate L-Cys, GSH, UA, AA and MT, a three-channel colorimetric sensor array was constructed through simultaneously using oxTMB, oxOPD and oxABTS as probes (Scheme 1). For each channel, the parameter A/A0 worked as the colorimetric response pattern. For each antioxidant (L-Cys, GSH, UA, AA and MT), three types of A/A0 (oxTMB, oxOPD and oxABTS) were tested six times, generating a matrix of 3 channels × 6 replicates × 5 analysts. Linear discrimination analysis (LDA) transformed the matrix into two canonical factors for generating a two-dimensional (2D) diagram, as shown in Fig. 3.32 First, for 1 μM L-Cys, GSH, UA, AA and MT, a unique 2D plot was obtained, as shown in Fig. 3A: (1) each point indicated a response pattern for an individual antioxidant against the designed sensor array, (2) these points correctly located in their respective regions without being crossly overlapped, and (3) five distinct regions were observed corresponding to L-Cys, GSH, UA, AA and MT, respectively. These results demonstrated that 1 μM L-Cys, GSH, UA, AA and MT could be completely identified (Fig. S9A and Table S3 in the ESI†). Furthermore, by decreasing the concentrations to 500 nM (Fig. S9B and Table S4 in the ESI†), the same result was obtained, as shown in Fig. 3B. Moreover, as shown in Fig. 3C, if these concentrations were further decreased to 100 nM, the same discriminating ability was observed (Fig. S9C and Table S5 in the ESI†). At 50 nM (Fig. S9D, ESI†), L-Cys, GSH and UA could be clearly identified, and AA and MT overlapped, as shown in Fig. 3D. Based on these results, it was believed that the sensor array could efficiently identify the five antioxidants as low as 100 nM. The jackknifed classification matrix showed that the classification accuracy of antioxidants was enhanced from 40% for the response signal from oxABTS to 100% for that from oxOPD, oxABTS and oxTMB (Fig. S9E, ESI†). In order to testify the discrimination ability, 15 unknown samples (containing 100 nM L-Cys, GSH, UA, AA and MT, respectively) were clearly identified with the accuracy of 100% (Table S6, ESI†).
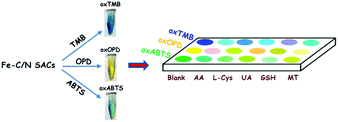 |
| Scheme 1 Schematic illustration of the three-channel colorimetric sensor array based on Fe–C/N for discriminating AA, L-Cys, UA, GSH and MT. | |
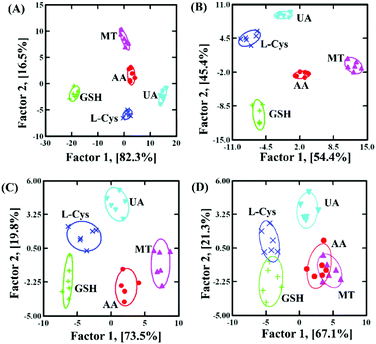 |
| Fig. 3 The canonical score plot of three receptors for discriminating five antioxidants at different concentrations: (A) 1000 nM, (B) 500 nM, (C) 100 nM and (D) 50 nM. | |
Based on the discrimination results discussed above, the sensor array was applied to quantify the concentrations. With GSH as a model analyst, 100 nM–5 μM GSH clearly located in different regions, as shown in Fig. 4A (Fig. S9F and Table S7 in the ESI†). Due to the fact that factor 2 (1.3%) was much smaller than factor 1 (98.5%), it was feasible to apply factor 1 to correlate with GSH concentration. As shown in Fig. 4B, the dose–response linear relationship between GSH and factor 1 suggested that the sensor array could quantify GSH as low as 100 nM. To further evaluate the applicability of the sensor array to identify the mixture, GSH and MT with different molar ratios (GSH
:
MT = 3
:
7, 5
:
5, 7
:
3 at a total concentration of 500 nM) were investigated. As shown in Fig. 5A (Table S8, ESI†), with the help of LDA patterns, the mixture of GSH and MT, as well as 500 nM GSH or MT, was distinctly discriminated from each other. Moreover, the mixture of L-Cys and MT with different molar ratios (L-Cys /MT = 3
:
7, 5
:
5, 7
:
3 at a total concentration of 500 nM), as well as 500 nM L-Cys or MT, was also identified, as shown in Fig. 5B (Table S9, ESI†). Interestingly, even the mixture of GSH, MT and L-Cys with different molar ratios (GSH
:
MT
:
L-Cys = 6
:
2
:
2, 2
:
6
:
2, 2
:
2
:
6 at a total concentration of 500 nM), as well as 500 nM GSH, MT or L-Cys, was also identified, as shown in Fig. 5C (Table S10, ESI†). In order to testify the discrimination ability, 18 unknown samples (containing GSH
:
MT
:
L-Cys = 6
:
2
:
2, 2
:
6
:
2, 2
:
2
:
6 at a total concentration of 500 nM, as well as 500 nM GSH, MT or L-Cys, respectively) were clearly identified with the accuracy of 100% (Table S11, ESI†). These results showed that the discrimination ability of the sensor array was well maintained in binary or ternary mixtures.
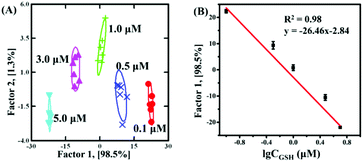 |
| Fig. 4 Identification of GSH at various concentrations using the colorimetric sensor array: (A) the canonical score plot as obtained from LDA for GSH at different concentrations; (B) the plot of the discriminant factor 1 versus the logarithm of GSH concentrations. | |
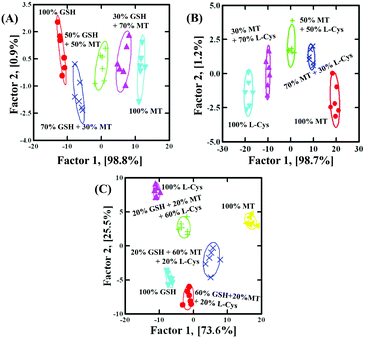 |
| Fig. 5 (A) The canonical score plot for the discrimination of binary mixtures containing GSH and MT at the total concentration of 500 nM; (B) the canonical score plot for the discrimination of binary mixtures containing MT and L-Cys at the total concentration of 500 nM; and (C) the canonical score plot for the discrimination of ternary mixtures containing GSH, MT and L-Cys at the total concentration of 500 nM. | |
Potential applications in human serum
Finally, the feasibility of discriminating antioxidants in human serum samples was further validated. In 100-fold diluted human serum samples, 500 nM L-Cys, GSH, UA, AA and MT were spiked. As shown in Fig. 6A, the serum itself produced a background response, and such a response did not affect the response of L-Cys, GSH, UA, AA and MT, exhibiting an anti-interfered capacity from the compositions in human serum. Serum, 500 nM L-Cys, GSH, UA, AA and MT were located in six different matrices and distinguished from each other (Fig. 6B and Table S12 in the ESI†). Moreover, 15 unknown samples (containing 500 nM L-Cys, GSH, UA, AA and MT, respectively) in 100-fold diluted human serum could be correctly distinguished (Table S13, ESI†). In addition, the quantification of GSH in 100-fold diluted human was further analyzed: in spiked human serum with GSH at 100 nM, 500 nM and 1000 nM, the detected GSH was 93.3 nM, 510.8 nM and 1035.1 nM, respectively (Table S14, ESI†). The recovery was 93.3–103.5%. These results revealed that the three-channel sensor array could be applied to discriminate and quantify biological antioxidants in human serum.
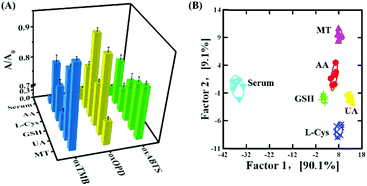 |
| Fig. 6 (A) Colorimetric sensor array with oxTMB, oxOPD or oxABTS as receptors for 500 nM AA, L-Cys, GSH, UA and MT spiked in the human serum. Error bars represent the standard deviations from three replicates; (B) the canonical score plot for discriminating AA, L-Cys, GSH, UA and MT at 500 nM spiked in the human serum. | |
Conclusions
In summary, as a single-atom nanozyme, Fe–N/C with oxidase-like activity catalysed the oxidation of TMB, OPD and ABTS to blue oxTMB, yellow oxOPD and green oxABTS. With oxTMB, oxOPD and oxABTS as three colorimetric channels, a colorimetric sensor array was constructed for discriminating L-Cys, GSH, UA, AA and MT as low as 100 nM. Moreover, the established sensor array could not only quantify individual antioxidant and discriminate the binary and ternary mixtures, but also correctly identify blind samples containing L-Cys, GSH, UA, AA or MT in buffer solutions and spiked human serum. Finally, the performance of the sensor array was successfully testified in human serum, showing potential applications in biomedical diagnosis. Compared with the reported sensor arrays based on 3–5 species, the developed sensor array based on a Fe–C/N single-atom nanozyme with oxidase-like activity provided a simplified strategy for constructing reliable sensor arrays.
Conflicts of interest
There are no conflicts to declare.
Acknowledgements
This work was supported by the National Natural Science Foundation of China (21673022) and the Fundamental Research Funds for the Central Universities.
Notes and references
- X. Zhang, Z. Chen and X. Zuo, ACS Sustainable Chem. Eng., 2020, 8, 3922–3928 CrossRef CAS.
- L. Gülin, Arch. Toxicol., 2012, 86, 345–391 CrossRef.
- J. Liu, Y. Q. Sun, Y. Huo, H. Zhang, L. Wang, P. Zhang, D. Song, Y. Shi and W. Guo, J. Am. Chem. Soc., 2014, 136, 574–577 CrossRef CAS.
- X. Chen, Y. Zhou, X. Peng and J. Yoon, ChemInform, 2010, 39, 2120–2135 CAS.
- H. Jung, X. Chen, J. Kim and J. Yoon, ChemInform, 2013, 42, 6019–6031 CAS.
- Y. Y. Sautin and R. J. Johnson, Nucleosides, Nucleotides Nucleic Acids, 2008, 27, 608–619 CrossRef CAS.
- X. Zhu, T. Zhao, Z. Nie, Y. Liu and S. Yao, Anal. Chem., 2015, 87, 8524–8530 CrossRef CAS.
- M. H. Lee, J. L. Thomas, Y. C. Chang, Y. S. Tsai, B. Liu and H. Lin, Biosens. Bioelectron., 2016, 79, 789–795 CrossRef CAS.
- A. R. Ivanov, I. V. Nazimov, L. Baratova, A. P. Lobazov and G. B. Popkovich, J. Chromatogr. A, 2001, 913, 315–318 CrossRef CAS.
- P. Liu, Y. Q. Huang, W. J. Cai, B. F. Yuan and Y. Q. Feng, Anal. Chem., 2014, 86, 9765–9773 CrossRef CAS.
- F. Matemadombo, C. Apetrei, T. Nyokong, M. L. Rodríguez-Méndez and J. A. D. Saja, Sens. Actuators, B, 2012, 166–167 Search PubMed.
- G. G. Huang, X. X. Han, M. K. Hossain and Y. Ozaki, Anal. Chem., 2009, 81, 5881–5888 CrossRef CAS.
- Z. P. Lou, S. Zhao, Q. Wang and H. Wei, Anal. Chem., 2019, 91, 15267–15274 CrossRef CAS.
- Y. Ding, G. Wang, F. Sun and Y. Lin, ACS Appl. Mater. Interfaces, 2018, 10, 32567–32578 CrossRef CAS.
- M. Huang, H. Wang, D. He, P. Jiang and Y. Zhang, Chem. Commun., 2019, 55, 3634–3637 RSC.
- X. Li, X. Y. Yang, J. Q. Sha, T. Han, C. J. Du, Y. J. Sun and Y. Q. Lan, ACS Appl. Mater. Interfaces, 2019, 11, 16896–16904 CrossRef CAS.
- M. Gao, X. Lu, S. Chen, D. Tian, Y. Zhu and C. Wang, ACS Appl. Nano Mater., 2018, 1, 4703–4715 CrossRef CAS.
- X. Li, C. Kong and Z. Chen, ACS Appl. Mater. Interfaces, 2019, 11, 9504–9509 CrossRef CAS.
- J. Du, J. Wang, W. Huang, Y. Deng and Y. He, Anal. Chem., 2018, 90, 9959–9965 CrossRef CAS.
- M. Gao, X. Lu, M. Chi, S. Chen and C. Wang, Inorg. Chem. Front., 2017, 4, 1862–1869 RSC.
- L. Song, Y. Zhu, Z. Yang, C. Wang and X. Lu, J. Mater. Chem. B, 2018, 6, 5931–5939 RSC.
- N. Song, F. Ma, Y. Zhu, S. Chen, C. Wang and X. Lu, ACS Sustainable Chem. Eng., 2018, 6, 16766–16776 CrossRef CAS.
- M. Gao, X. Lu, S. Chen, D. Tian, Y. Zhu and C. Wang, ACS Appl. Nano Mater., 2018, 9, 4703–4715 CrossRef.
- T. Jin, Y. Li, W. Jing, Y. Li, L. Fan and X. Li, Chem. Commun., 2020, 56, 659–662 RSC.
- H. Li, Y. Wen, X. Zhu, J. Wang, L. Zhang and B. Sun, ACS Sustainable Chem. Eng., 2020, 8, 520–526 CrossRef CAS.
- J. R. Askim, M. Mahmoudi and K. S. Suslick, Chem. Soc. Rev., 2013, 42, 8649 RSC.
- K. Diehl and E. Anslyn, ChemInform, 2013, 42, 8596–8611 CAS.
- C. C. You, O. R. Miranda, B. Gider, P. S. Ghosh, I. B. Kim, B. Erdogan, S. A. Krovi, U. H. F. Bunz and V. M. Rotello, Nat. Nanotechnol., 2007, 2, 318–323 CrossRef CAS.
- M. De, S. Rana, H. Akpinar, O. R. Miranda, R. R. Arvizo, U. H. F. Bunz and V. M. Rotello, Nat. Chem., 2009, 1, 461–465 CrossRef CAS.
- J. Mao, Y. Lu, N. Chang, J. Yang, S. Zhang and Y. Liu, Analyst, 2016, 141, 4014–4017 RSC.
- N. Chang, Y. Lu, J. Mao, M. Li, S. Zhang and Y. Liu, Analyst, 2016, 141, 2046–2052 RSC.
- N. D. B. Le, G. Y. Tonga, R. Mout, S. T. Kim, M. E. Wille, S. Rana, K. A. Dunphy, D. J. Jerry, M. Yazdani and R. Ramanathan, J. Am. Chem. Soc., 2017, 139, 8008–8012 CrossRef CAS.
- Z. Pode, R. Peri-Naor, J. M. Georgeson, T. Ilani, V. Kiss, T. Unger, B. Markus, H. M. Barr, L. Motiei and D. Margulies, Nat. Nanotechnol., 2017, 12, 1161–1168 CrossRef CAS.
- X. Wang, L. Qin, M. Zhou, Z. Lou and H. Wei, Anal. Chem., 2018, 90, 11696–11702 CrossRef CAS.
- S. Li, K. Li, X. Li and Z. Chen, ACS Appl. Mater. Interfaces, 2019, 11, 37371–37378 CrossRef CAS.
- W. Huang, Y. Q. Deng and Y. He, Biosens. Bioelectron., 2017, 89–94 CrossRef.
- D. Yuan, H. Yan, J. Liu, J. Liu and J. Wang, Chin. Chem. Lett., 2019, 31, 455–458 CrossRef.
- X. Li, C. Kong and Z. Chen, ACS Appl. Mater. Interfaces, 2019, 11, 9504–9509 CrossRef CAS.
- X. Zhang, Q. Liu, Z. Chen and X. Zuo, Talanta, 2020, 215, 120935 CrossRef CAS.
- D. Yuan, J. J. Liu, H. H. Yan, C. M. Li, C. Z. Huang and J. Wang, Talanta, 2019, 203, 220–226 CrossRef CAS.
- W. Wei, X. Shi, P. Gao, S. Wang, W. Hu, X. Zhao, Y. Ni, X. Xu, Y. Xu and W. Yan, Nano Energy, 2018, 52, 29–37 CrossRef CAS.
- X. Liu, L. Huang, Y. Wang, J. Sun, T. Yue, W. Zhang and J. Wang, Sens. Actuators, B, 2020, 306, 127565 CrossRef CAS.
- Y. Peng, X. Chen, G. Yi and Z. Gao, Chem. Commun., 2011, 47, 2916–2918 RSC.
- L. Wang, Y. Zeng, A. Shen, X. Zhou and J. Hu, Chem. Commun., 2015, 51, 2052–2055 RSC.
Footnote |
† Electronic supplementary information (ESI) available: Characterization of Fe–N/C, UV-vis spectra of TMB-, OPD-, and ABTS-Fe–N/C systems, steady-state dynamic curves with TMB, OPD and ABTS as substrates, source data about blind samples. See DOI: 10.1039/d0an01447h |
|
This journal is © The Royal Society of Chemistry 2021 |
Click here to see how this site uses Cookies. View our privacy policy here.