DOI:
10.1039/D0AN01656J
(Paper)
Analyst, 2021,
146, 146-155
A label-free photoelectrochemical immunosensor for carcinoembryonic antigen detection based on a g-C3N4/CdSe nanocomposite†
Received
17th August 2020
, Accepted 2nd October 2020
First published on 5th October 2020
Abstract
Herein, a label-free photoelectrochemical immunosensor based on a g-C3N4/CdSe nanocomposite was established and applied to detect carcinoembryonic antigen (CEA). The prepared nanocomposite materials were characterized by transmission electron microscopy (TEM), X-ray diffraction (XRD), ultraviolet-visible absorption spectroscopy (UV-vis), X-ray photoelectron spectroscopy (XPS), fourier transform infrared spectrometer (FT-IR) and photoluminescence spectroscopy (PL). The results indicate that g-C3N4/CdSe nanocomposite materials were successfully synthesized. In a typical assembly process, the immunosensor was constructed by modifying a fluorine-doped tin oxide (FTO) electrode with poly dimethyl diallyl ammonium chloride (PDDA), the g-C3N4/CdSe nanocomposite, the anti-carcinoembryonic antigen antibody (Ab) and the blocking agent bovine serum albumin (BSA) successively. In the presence of CEA, the photocurrent signal of the prepared immunosensor decreased significantly. Accordingly, under the optimal conditions, a label-free photoelectrochemical immunosensor was established, and it exhibited excellent selectivity and repeatability for CEA detection. The detection limit was 0.21 ng mL−1, and the range was 10 ng mL−1–100 μg mL−1. Simultaneously, the immunosensor also provides a likely sensing device for detecting other protein targets, which is of great significance for early clinical diagnosis.
1 Introduction
Malignant tumors have become one of the greatest threats to human health, and a mass of investigation results show that one-sixth of deaths around the world are caused by malignant tumors.1,2 Therefore, the efficient and early diagnosis of malignant tumors is crucial to improving the survival rate.2,3 Carcinoembryonic antigen (CEA), as one of the most common biomarkers in malignant tumors, is generally acknowledged as an effective indicator and widely used in clinical diagnosis.2–4 The normal concentration of serum CEA in healthy adults is less than 5 ng mL−1;1 therefore the CEA level in human serum is a significant index of the state of tumor progression,5,6 and diverse analytical methods have been used for the detection of CEA, such as fluorometry, electrochemistry, electrochemiluminescence, radioimmunoassay and photoelectrochemistry.2–4 Among them, photoelectrochemistry has attracted lots of attention because of its native properties such as low background, fast analysis, simple apparatus and low cost.3 Although photoelectrochemistry has been developed for detecting CEA, the photoelectrochemical sensors used therein still have some constraints, such as complex assembly and limited sensitivity.7–10 Therefore, developing an efficient and straightforward photoelectrochemical sensor for CEA detection in human blood sera with high sensitivity is of great significance for early clinical diagnosis.
Photoelectrochemical biosensing is a quite promising analytical approach, and has attracted lots of attention due to its superior analytical performance.11–13 A typical photoelectrochemical analysis procedure refers to the photon–electricity conversion process, and in order to obtain an ideal photoelectrochemical sensing system, the choice of photoactive materials is a critical factor.14,15 To date, various kinds of photoactive nanomaterials such as zero dimensional (0D), one dimensional (1D) and two dimensional (2D) nanomaterials have been studied in photoelectrochemical sensing fields.16 Among the above materials, 2D nanomaterials have attracted lots of attention due to their outstanding advantages such as good electron mobility, more surface active sites and large surface area.16 Graphite-like carbon nitride (g-C3N4), a 2D metalloid semiconductor photoactive material, has attracted widespread attention because of its unique optical and electrical properties, such as a relatively narrow band gap (2.7 eV), high visible optical absorption, excellent charge separation and transport capabilities, remarkable thermal and chemical stability and good biocompatibility.17–19 Nevertheless, the high recombination rate of photogenic electron–hole pairs of pure g-C3N4 restricts its application in the photoelectrochemical sensing field.20 To surmount this disadvantage and improve the photoelectric conversion efficiency, some alterations are necessary for g-C3N4. For example, 0D quantum dots (QDs) with a suitable band gap can effectively reduce the recombination rate of photogenic electron–hole pairs and extend their light-capturing ability.21 In the past few years, CdSe has attracted lots of attention and has been efficiently used as a sensitizer for wide band gap semiconductors due to its narrow band gap of 1.74 eV.22,23 Hence, in this work, CdSe was used as the sensitizer for g-C3N4 to construct a sensitive photoelectrochemical immunosensor.
In this work, a g-C3N4/CdSe nanocomposite was used as the photoactive material, and a convenient photoelectrochemical immunosensor for detecting CEA was developed. It can be seen from Scheme 1 that the g-C3N4/CdSe nanocomposite was dropped on the FTO electrode surface pretreated with poly dimethyl diallyl ammonium chloride (PDDA). After air-drying, a uniform functional nanofilm was formed and used as the matrix electrode for the proposed immunosensor. Subsequently, the antibody (Ab), which is specifically recognized by CEA, was incubated on the electrode surface, and the unbound sites were blocked with BSA. For target CEA detection, different concentrations of CEA were incubated on the electrode surface, and the photocurrent intensity changed with the change in the concentration of CEA. The developed photoelectrochemical immunosensor provides a specific, stable and convenient idea for the detection of other biomarkers.
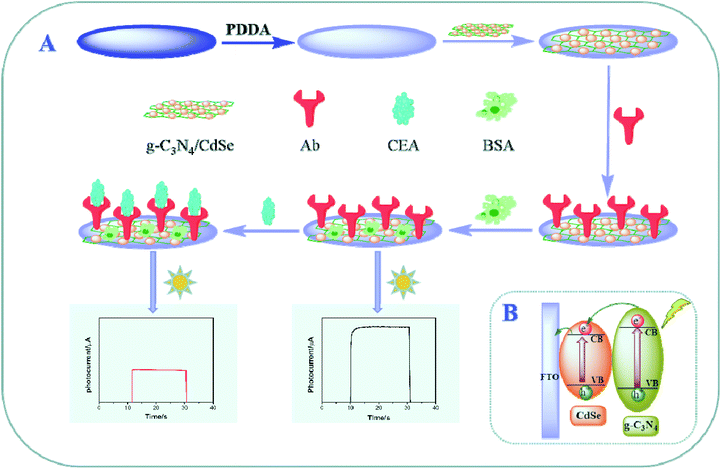 |
| Scheme 1 (A) Schematic showing the steps involved in the fabrication of the photoelectrochemical immunosensor. (B) Possible photo-generation electron-transfer mechanism of the immunosensor electrode. | |
2 Experiment
2.1 Synthesis of g-C3N4, CdSe and the g-C3N4/CdSe nanocomposite
The synthetic method of g-C3N4 was based on the literature methods.20 Briefly, melatine (10 g) was calcined at 550 °C under an air atmosphere for 4 h. After cooling to room temperature, the yellow product was milled into a powder. Then, the prepared powder was sintered at 500 °C under atmospheric conditions for another 2 h. After natural cooling, the obtained powder was dispersed with ultrapure water by ultrasonicating for 12 h. Thereafter, the suspension was purified by centrifuging at 6000 rpm for 15 min and the residual bulk g-C3N4 was removed. Finally, the milky suspension was stored for further use.
The L-cysteine-modified CdSe QDs were prepared according to the literature method.24 Briefly, NaSeSO3 aqueous solution was synthesized by dissolving 0.3950 g of Se powder and 1.890 g of Na2SO3 in 50 mL of deionized water under a nitrogen atmosphere at 75 °C for 8 h. The fresh NaSeSO3 solution was obtained. Then, 2.16 mL of 1 M L-cysteine and 0.2510 g of CdCl2 were added to 200 mL of ultrapure water and stirred to form a homogeneous solution. Then, 1 M NaOH was added gradually to adjust the pH of the solution to 11, and then deaerated with high-purity N2 for 30 min. Thereafter, 2.25 mL of NaSeSO3 was injected into this mixture and refluxed continuously for 2 h at 75 °C under highly pure N2 protection. Finally, a bright yellow solution was obtained. After cooling, the CdSe QDs were washed thoroughly with anhydrous ethanol and centrifuged at 8000 rpm for 15 min, and the sediment was stored in a refrigerator at 4 °C for further use.
The preparation method of the g-C3N4/CdSe nanomaterial was as follows: the CdSe sediment was added into the g-C3N4 suspension and sonicated for 1 h. After being stored at 4 °C overnight, the mixture was purified by centrifuging at 9000 rpm for 15 min. In the end, the wet solid was dried overnight at 35 °C and stored in a refrigerator for further use.
2.2 Preparation of the FTO/g-C3N4/CdSe electrode
First, FTO electrodes were cleaned by ultrasonic treatment with acetone, ethyl alcohol, and water respectively and dried at 60 °C overnight; the effective working area of the electrode was 0.45 cm2. Next, 30 μL of PDDA (2%) was dropped on the electrode surface and kept for 1 h. After rinsing with 10 mM PBS (pH = 7.4) and drying under nitrogen, 30 μL of g-C3N4/CdSe suspension solution (0.2 mg mL−1) was deposited on the electrode surface and dried under atmospheric conditions. Afterward, the prepared electrode was flushed with ultrapure water. After drying under nitrogen, the sensor electrode was prepared successfully.
2.3 Fabrication of the immunosensor and CEA analysis
The anti-CEA antibody was a macromolecular protein, which contained –NH2 and –COOH groups, and the prepared CdSe was modified with L-cysteine. After the electrode was modified with g-C3N4/CdSe, the immunosensor electrode was incubated with 30 μL of EDC-NHS to activate the –NH2 and –COOH groups and rinsed with 10 mM PBS (pH = 7.4). Under atmospheric conditions, 30 μL of 1 mg mL−1 anti-CEA antibody was dropped on the electrode surface and incubated overnight at 4 °C, and the anti-CEA antibody was successfully bonded to the electrode surface by the amide bond. Subsequently, the electrode was rinsed with PBS, and the nonspecified binding sites were blocked with BSA (30 μL, 1%, w/v) at 37 °C for 1 h. After being rinsed with 10 mM PBS, the obtained electrode served as the photoelectrochemical immunosensor.
For CEA analysis, the resulting immunosensor electrode was incubated with 30 μL of CEA at different concentrations for 70 min. After rinsing with PBS and drying under nitrogen, the resultant immunosensor electrode was applied in photoelectrochemical measurement. Scheme 1 shows the whole fabricating steps of the prepared photoelectrochemical immunosensor.
2.4 Photoelectrochemical detection
The photoelectrochemical test was performed in 0.1 M PBS including 0.1 M AA, and AA served as the sacrificial electron donor in photoelectrochemical measurement.25 A 250 W Xe lamp was used in the whole work, and the wavelength range was 280–1000 nm. The impressed voltage was 0.0 V. The photoelectrochemical signal was recorded while the excitation light source was irradiated on the electrode surface.
3 Results and discussion
3.1 Characterization of g-C3N4, CdSe and the g-C3N4/CdSe nanocomposite
The surface elemental composition and chemical states of g-C3N4/CdSe studied by XPS measurements are shown in Fig. 1A. XPS can accurately measure an atom's inner electron binding energy and its chemical shift, providing many types of information for chemical research. In g-C3N4, C can be divided into three minor peaks. At 285.10, 288.06 and 288.8 eV, they are considered to be the C–OH group, C–C group, and N–C
N group, respectively. N 1s has three peaks at 398.78, 399.62 and 400.97 eV, which are C–N
C, N–H and N–(C)3 in g-C3N4.26–28 In CdSe, Cd produces two absorption peaks: Cd 3d5/2 (405.61 eV) and Cd 3d3/2 (412.58 eV), and two Se peaks: Se 3d5/2 (54.08 eV) and Se 3d3/2 (55.4 eV).29
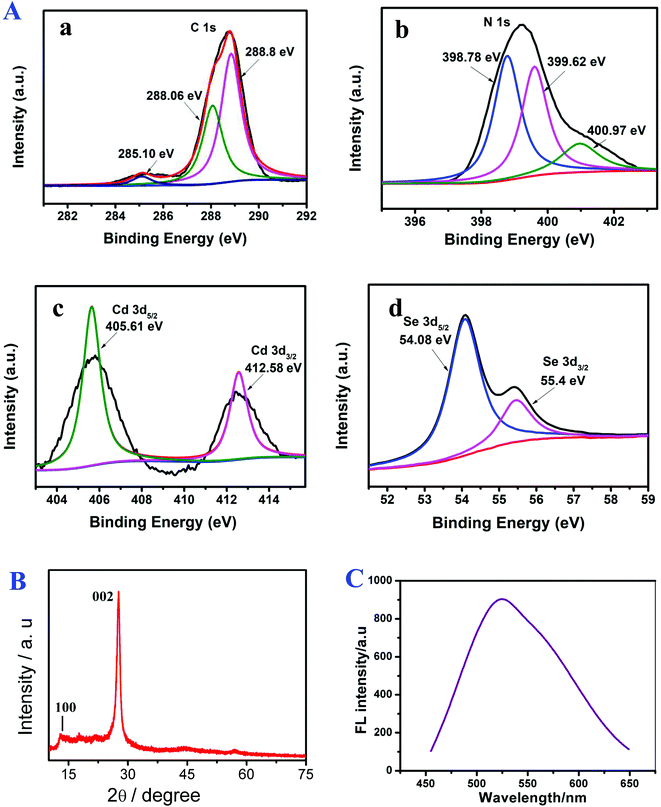 |
| Fig. 1 (A) XPS spectra of (a) C, (b) N, (c) Cd and (d) Se, (B) XRD pattern of g-C3N4 and (C) PL spectra of CdSe. | |
Fig. 1B shows the XRD pattern of g-C3N4. In the pattern, the diffraction peaks at 2θ = 12.6° (100) and 28.2° (002) (Card No. 019-0191) were assigned to pure g-C3N4.30,31 The optical property of CdSe QDs was investigated by PL.32 The surface morphology of the g-C3N4/CdSe nanocomposite was characterized by TEM and HRTEM. Fig. 2A and B show the TEM and HRTEM images of the g-C3N4/CdSe nanocomposite, respectively. It can be seen from Fig. 2A that there are both thin layer nanometer sheets and granular structures in the nanocomposite, and the thin layer nanometer sheet structure belonged to g-C3N4 and the granular structure can be ascribed to CdSe QDs. Fig. 2B shows the HRTEM images of the g-C3N4/CdSe nanocomposite. The lattice fringes of CdSe can clearly be seen in the image.
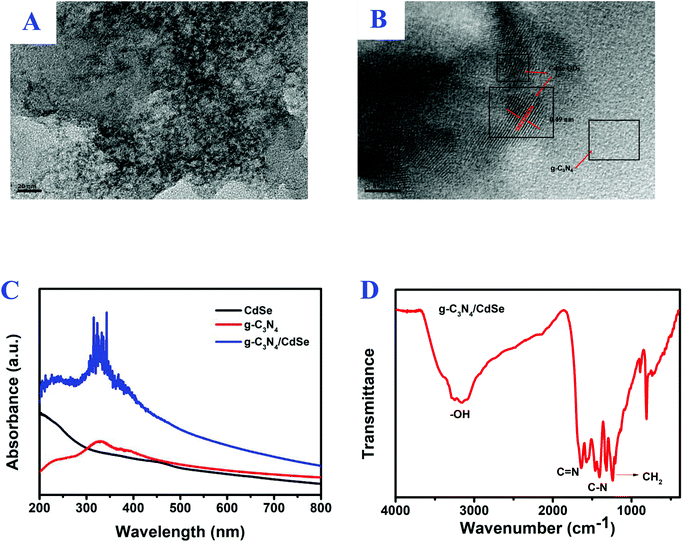 |
| Fig. 2 (A) TEM and (B) HRTEM images of the g-C3N4/CdSe nanocomposite, (C) UV-vis absorption spectrum of CdSe, g-C3N4 and g-C3N4/CdSe, and (D) FT-IR spectrum for g-C3N4/CdSe. | |
In order to study the light absorption of nanomaterials, the UV-vis absorption spectra of g-C3N4/CdSe, g-C3N4 and CdSe QOs were measured.33,34 After the nanomaterials absorb ultraviolet and visible light, a charge transition occurs, and then a band-shaped absorption spectrum is generated. The position of the spectral peak is used to determine the light utilization efficiency of the material. According to the spectrum of Fig. 2C, the pure CdSe QDs displayed an obvious absorption peak at 445 nm with an absorption edge at 500 nm, and in the PL emission spectra of CdSe QDs, the peak position of pure CdSe QDs was observed at 522 nm, which is consistent with the literature report.25,32 Furthermore, g-C3N4 has obvious absorption in the 200–440 nm wavelength range. The absorption intensity of the g-C3N4/CdSe composite material in the range of ultraviolet and visible light is greatly enhanced. It can be seen that, compared with a single material, composite nanomaterials have better light absorption capacity and higher light utilization efficiency.
FT-IR was used to verify the surface functionalities of g-C3N4/CdSe in Fig. 2D. The absorption bands that appeared in the range of 1235 cm−1 to 1645 cm−1 lead to the stretching vibrations in the heterocycles of C–N and C
N.33 The peak observed at 1462 cm−1 corresponds to CH2 bending and that at 1630 cm−1 corresponds to –NH bending in the spectra of CdSe-QDs. The intense peak in the spectra at 3271 cm−1 corresponds to the –OH group.34,35 It can also be seen that the characteristic peak position indicates that the structure of g-C3N4 did not change during the process of compounding g-C3N4 with CdSe. The XPS, XRD, PL, TEM, HRTEM, UV-vis, and FT-IR results proved that the photoactive nanocomposite was successfully prepared.
3.2 Optimized conditions
To effectively apply the constructed photoelectrochemical immunosensor to detect CEA, the incubation time of CEA with Ab, the concentrations of the g-C3N4/CdSe nanocomposite and anti-CEA antibody, and the ratio of g-C3N4 to CdSe were optimized. As shown in Fig. 3A, the photoelectrochemical signals increased with the increase in the amount of CdSe (the final applied amount of CdSe was set to 1). When the amount of CdSe was 1, the photoelectrochemical signal tended to exhibit the highest intensity. Then the photoelectrochemical signals decreased as the amount of CdSe increased from 1 to 2, which can be ascribed to the blockage of the transfer of electrons by the superfluous CdSe. Therefore, the amount of CdSe QDs used in this immunosensor was 1.
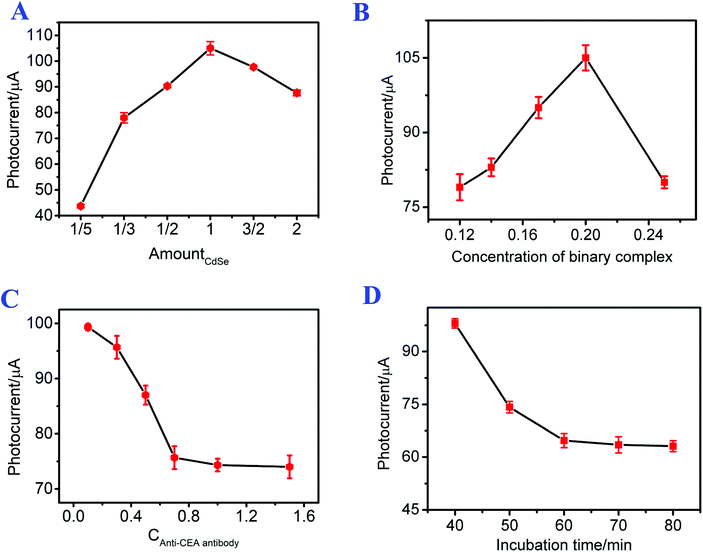 |
| Fig. 3 Effects of (A) the ratio of g-C3N4 to CdSe, the concentration of (B) the g-C3N4/CdSe nanocomposite and (C) the antibody, and (D) the incubation time of CEA with Ab. | |
It can be seen from Fig. 3B that the photocurrent intensity increased with the increasing concentration of g-C3N4/CdSe from 0.12 mg mL−1 to 0.2 mg mL−1, which is probably because the total quantity of optically active substances increased with the increasing concentration of g-C3N4/CdSe, leading to an increase of the photocurrent intensity. Thereafter, the photocurrent intensity decreased rapidly as the concentration of g-C3N4/CdSe increased from 0.2 mg mL−1 to 0.25 mg mL−1, which may be due to the saturation of the optically active substance, and the superfluous nanocomposites impeded the transfer of electrons. Thus, 0.2 mg mL−1 of g-C3N4/CdSe nanocomposite was used throughout the whole work.
The incubation concentration of the antibody has an evident impact on the linear detection range of the immunosensor; thus the concentration of the antibody was optimized. As shown in Fig. 3C, the photocurrent signals of the photoelectrochemical immunosensor decreased with the increasing concentration of the antibody, and the changes of the photoelectrochemical intensity tended to be gentle when the concentration of the antibody was up to 1 mg mL−1, meaning that the immunosensor electrode cannot combine with the excess amount of antibody. Therefore, 1 mg mL−1 of antibody was used in this work.
To investigate the relationship between the incubation time of CEA with Ab and the photocurrent, the photocurrent response signals of the photoelectrochemical immunosensor at different incubation times of CEA (30 μL, 1 ng mL−1) with Ab were studied. As shown in Fig. 3D, the photocurrent intensity of the photoelectrochemical immunosensor decreased with increasing incubation time of CEA with Ab from 40 min to 70 min. As the incubation time increased continually, the photocurrent intensity remained constant. The result means that the combination of CEA and Ab was complete at 70 min. Accordingly, 70 min was chosen as the incubation time of CEA with Ab throughout the whole work.
3.3 Photoelectrochemical and EIS behavior
To investigate the modification process of the photoelectrochemical immunosensor, the photocurrent signals of the modified electrodes are shown in Fig. 4A. The FTO electrode exhibited a small photocurrent signal response (curve a, ∼0 μA). After the g-C3N4/CdSe nanocomposite was coated on the electrode surface, the photoelectrochemical immunosensor showed a relatively high photocurrent intensity (curve b, I = 105 μA) because the direct band gap of g-C3N4 matched the energy levels of CdSe QDs, which could effectively suppress the recombination of the photo-generated electron–hole pairs and extend their light-capturing ability. As Ab and BSA were incubated on the electrode surface step by step, the photocurrent response decreased to 75 μA (curve c). The reduction of the photocurrent can be attributed to the assembled materials sectionally inhibiting the electron transfer between AA and photogenic electron–hole pairs.36,37 After the target CEA was incubated on the electrode surface, the photocurrent response decreased continually (curve d, ∼60 μA), which can be attributed to the protein insulation layer, and implied that the target CEA had combined with anti-CEA successfully. The photocurrent response of the proposed immunosensor at different fabrication steps confirmed the successful assembly of the immunosensor.
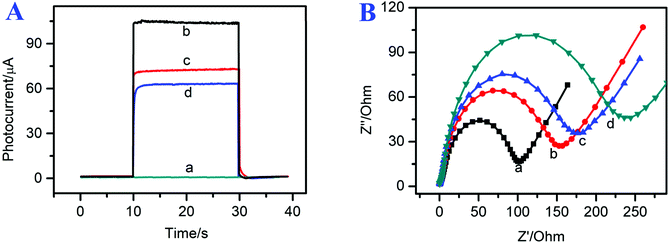 |
| Fig. 4 (A) Photocurrent responses for the stepwise immunosensor fabrication and (B) the corresponding EIS spectra: (a) FTO, (b) FTO/g-C3N4/CdSe, (c) FTO/g-C3N4/CdSe/Ab/BSA and (d) FTO/g-C3N4/CdSe/Ab/BSA/CEA. | |
To further demonstrate the successful preparation of the photoelectrochemical immunosensor, electrochemical impedance spectroscopy (EIS) was used to characterize the assembly steps of the immunosensor interface. The EIS spectrum is composed of a semicircular part, from the electron transfer-limited process, and a linear part from the diffusion-limited process. The diameter of the semicircular area is the electron-transfer resistance, which represents the electron-transfer status of the assembly process. As shown in curve a of Fig. 4B, the bare FTO electrode shows a small electron transfer resistance (Ret) of approximately 100 Ω. After the g-C3N4/CdSe nanocomposite was coated on the FTO electrode, the resistance increased to 155 Ω, which can be attributed to the low conductivity and reduced charge transfer of CdSe QDs and non-metallic semiconductors.25 Next, Ab and BSA were coated on the electrode surface; the resistance increased to 180 Ω, and the increase of the resistance can be assigned to the low conductivity and evident steric hindrance of the protein molecules.38 Finally, the electrode was immobilized with 1 μg mL−1 of target CEA (curve d, 235 Ω), and the resistance increased continually, indicating that CEA was incubated successfully. The EIS results were in accordance with the photocurrent response of the proposed immunosensor at different fabrication steps, indicating that the photoelectrochemical immunosensor was assembled successfully.
3.4 Photoelectrochemical detection of CEA
Under the optimized experimental conditions, the prepared photoelectrochemical immunosensor was applied to CEA detection, and the photocurrent signal of the assembled immunosensor was directly related to the CEA concentration. Fig. 5A shows the photocurrent response of the immunosensor at different CEA concentrations. After CEA incubation, the signal intensity of the immunosensor decreased with increasing CEA concentration from 10 ng mL−1 to 100 μg mL−1, and the detection limit of the proposed immunosensor was 0.21 ng mL−1. Fig. 5B shows the linear relation between the photocurrent signal and logarithm of the CEA concentration, and the linear regression equation was I (μA) = 61.1045–7.0407
log
CCEA/μg mL−1 (n = 3), with a linearly dependent coefficient of 0.996. The detection results indicate that this method can efficaciously detect CEA, and the linear regression equation can facilitate the evaluation of the quantity of CEA. For the purpose of demonstrating the performance of the established immunosensor, the immunosensor was compared with other existing sensors and protocols. As shown in Table S1,† the proposed photoelectrochemical immunosensor showed a wider range in CEA detection.
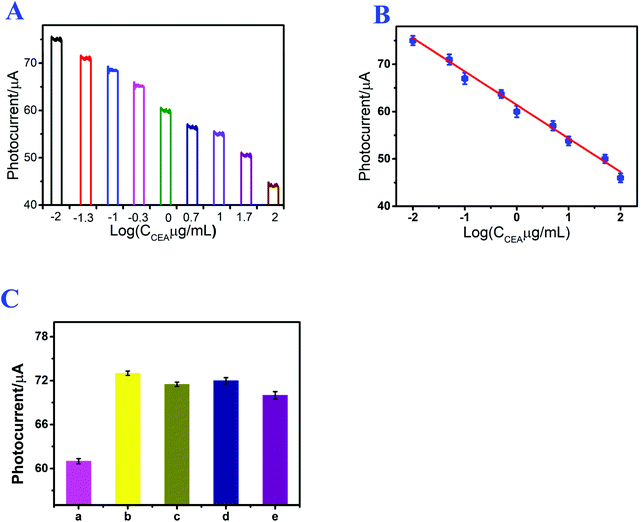 |
| Fig. 5 (A) Photocurrent response and (B) the corresponding calibration curve of the immunosensor for the detection of different concentrations of CEA from 10 ng mL−1 to 100 μg mL−1. (C) Selectivity of the proposed photoelectrochemical immunosensor with different interferences: (a) 1 μg mL−1 CEA, (b) 10 μg mL−1 Cyt C, (c) 10 μg mL−1 IL-6, (d) 10 μg mL−1 AA and (e) blank. | |
3.5 Repeatability, specificity and stability
Repeatability is a significant index for photoelectrochemical immunosensors and also an important part of analytical methods. The repeatability of the established photoelectrochemical immunosensor was studied by analyzing the photocurrent responses of three immunosensor electrodes that were modified with 1 μg mL−1 of CEA. The relative standard deviation was 2.73%, and the result demonstrated the acceptable repeatability of the established photoelectrochemical immunosensor for CEA detection.
Specificity is also a crucial index for immunosensors and affects the sensitivity of photoelectrochemical immunosensors. Thus, to explore the specificity of the established photoelectrochemical immunosensor, the resultant immunosensor electrodes were used for detecting other different interferences, such as AA, IL-6 and Cyt C. As shown in Fig. 5C, in the presence of CEA, the immunosensor exhibited a relatively low photocurrent. After replacing CEA with AA, IL-6 and Cyt C, the photocurrent responses of the immunosensor electrode were much closer to the result of the blank test. The results indicated that the assembled photoelectrochemical immunosensor possessed excellent specificity for CEA detection.
The storage stability of the proposed immunosensor for CEA detection was also investigated. After the immunosensor electrode was stored for 2 weeks at 4 °C, the photocurrent response of the immunosensor was 98.2% of the original value. The results demonstrated that the established photoelectrochemical immunosensor possessed excellent storage stability.
4 Conclusions
In conclusion, a simple and convenient photoelectrochemical immunosensor based on FTO/g-C3N4/CdSe electrodes for detecting CEA was assembled successfully. Under the optimized experimental conditions, the proposed photoelectrochemical immunosensor displayed an outstanding detection range and detection limit. Owing to its good specificity and reproducibility, the established photoelectrochemical immunosensor has promise for target CEA detection, and has further application potential in the detection and biological analysis of other antigens.
Conflicts of interest
The authors declare that they have no conflicts of interest.
Acknowledgements
This work was supported by the National Natural Science Foundation of China (Grant No. 21976001), Natural Science Foundation of Anhui Province (1808085QB53), and Open Fund for Discipline Construction of Institute of Physical Science and Information Technology.
References
- Q. Wu, N. B. Li, Y. Wang, Y. Liu, Y. C. Xu, S. T. Wei, J. D. Wu, G. R. Jia, X. D. Fang, F. F. Chen and X. Q. Cui, A 2D transition metal carbide MXene-based SPR biosensor for ultrasensitive carcinoembryonic antigen detection, Biosens. Bioelectron., 2019, 144, 111697 CrossRef CAS.
- J. L. Sun, K. Cui, L. Li, L. N. Zhang and J. H. Yu, Visible-light-driven renewable photoelectrochemical/synchronous visualized sensing platform based on Ni:FeOOH/BiVO4 photoanode and enzymatic cascade amplification for carcinoembryonic antigen detection, Sens. Actuators, B, 2020, 304, 127301 CrossRef CAS.
- C. Y. Wang, Y. Y. Wang, H. J. Zhang, H. P. Deng, X. X. Xiong, C. Y. Li and W. W. Li, Molecularly imprinted photoelectrochemical sensor for carcinoembryonic antigen based on polymerized ionic liquid hydrogel and hollow gold nanoballs/MoSe2 nanosheets, Anal. Chim. Acta, 2019, 1090, 64–71 CrossRef CAS.
- X. T. Li, H. Y. Lv, X. X. Sun and C. F. Ding, Green-emitting carbon dot loaded silica nanoparticles coated with DNA-cross-linked hydrogels for sensitive carcinoembryonic antigen detection and effective targeted cancer therapy, Chem. Commun., 2019, 55, 15101–15104 RSC.
- J. Feng, X. Li, H. Cheng, W. Y. Huang, H. X. Kong, Y. Q. Li and L. J. Li, A boronate-modified molecularly imprinted polymer labeled with a SERS-tag for use in an antibody-free immunoassay for the carcinoembryonic antigen, Microchim. Acta, 2019, 186, 774 CrossRef CAS.
- S. X. Wu, H. L. Tan, C. H. Wang, J. H. Wang and S. R. Sheng, A colorimetric immunoassay based on coordination polymer composite for the detection of carcinoembryonic antigen, ACS Appl. Mater. Interfaces, 2019, 11, 43031–43038 CrossRef CAS.
- X. S. Gao, S. Y. Niu, J. J. Ge, Q. Y. Luan and G. F. Jie, 3D DNA nanosphere-based photoelectrochemical biosensor combined with multiple enzyme-free amplification for ultrasensitive detection of cancer biomarkers, Biosens. Bioelectron., 2020, 147, 111778 CrossRef CAS.
- D. Huang, L. Wang, Y. Zhan, L. N. Zou and B. X. Ye, Photoelectrochemical biosensor for CEA detection based on SnS2-GR with multiple quenching effects of Au@CuS-GR, Biosens. Bioelectron., 2019, 140, 111358 CrossRef CAS.
- Y. H. Zhang, M. J. Li, H. J. Wang, R. Yuan and S. P. Wei, Supersensitive photoelectrochemical aptasensor based on Br, N-codoped TiO2 sensitized by quantum dots, Anal. Chem., 2019, 91, 10864–10869 CrossRef CAS.
- L. Ge, W. X. Wang, T. Hou and F. Li, A versatile immobilization-free photoelectrochemical biosensor for ultrasensitive detection of cancer biomarker based on enzyme-free cascaded quadratic amplification strategy, Biosens. Bioelectron., 2016, 77, 220–226 CrossRef CAS.
- Z. P. Yang, T. T. Qin, Y. T. Niu, Y. Y. Zhang, C. J. Zhang, P. Li, M. Zhu, Y. H. Jia and Q. W. Li, Flexible visible-light-driven photoelectrochemical biosensor based on molecularly imprinted nanoparticle intercalation-modulated graphene fiber for ultrasensitive urea detection, Carbon, 2020, 157, 457–465 CrossRef CAS.
- J. Luo, L. C. Fang, H. M. Liu, Q. J. Zhu, H. Huang, J. Deng, F. X. Liu, Y. Li and J. S. Zheng, Dual-signal amplified photoelectrochemical assay for DNA methyltransferase activity based on RGO-CdS:Mn nanoparticles and a CdTe@DNA network, Sens. Actuators, B, 2020, 304, 127266 CrossRef CAS.
- H. Wang, K. Q. Deng, J. Xiao, C. X. Li, S. W. Zhang and X. F. Li, A sandwich-type photoelectrochemical sensor based on tremella-like graphdiyne as photoelectrochemical platform and graphdiyne oxide nanosheets as signal inhibitor, Sens. Actuators, B, 2020, 304, 127363 CrossRef CAS.
- X. Y. Yang, L. Chen, X. X. Xiong, Y. Shu, D. Q. Jin, Y. Zang, W. Wang, Q. Xu and X. Y. Hu, Molecularly imprinted polymers and PEG double engineered perovskite: an efficient platform for constructing aqueous solution feasible photoelectrochemical sensor, Sens. Actuators, B, 2020, 304, 127321 CrossRef CAS.
- P. C. Yan, J. T. Dong, Z. Mo, L. Xu, J. C. Qian, J. X. Xia, J. M. Zhang and H. N. Li, Enhanced photoelectrochemical sensing performance of graphitic carbon nitride by nitrogen vacancies engineering, Biosens. Bioelectron., 2020, 148, 111802 CrossRef CAS.
- Y. Ma, Y. X. Dong, B. Wang, S. W. Ren, J. T. Cao and Y. M. Liu, CdS:Mn-sensitized 2D/2D heterostructured g-C3N4-MoS2 with excellent photoelectrochemical performance for ultrasensitive immunosensing platform, Talanta, 2020, 207, 120288 CrossRef CAS.
- Y. H. Xu, Z. R. Wen, T. S. Wang, M. Zhang, G. F. Ding, Y. S. Guo, D. Jiang and K. Wang, Ternary Z-scheme heterojunction of Bi SPR-promoted BiVO4/g-C3N4 with effectively boosted photoelectrochemical activity for constructing oxytetracycline aptasensor, Biosens. Bioelectron., 2020, 166, 112453 CrossRef CAS.
- L. Ge, Y. H. Xu, L. J. Ding, F. H. You, Q. Liu and K. Wang, Perovskite-type BiFeO3/ultrathin graphite-like carbon nitride nanosheets p-n heterojunction: Boosted visible-light-driven photoelectrochemical activity for fabricating ampicillin aptasensor, Biosens. Bioelectron., 2019, 124–125, 33–39 CrossRef CAS.
- T. Y. Shi, H. N. Li, L. J. Ding, F. H. You, L. Ge, Q. Liu and K. Wang, Facile preparation of unsubstituted iron(II) phthalocyanine/carbon nitride nanocomposites: A multipurpose catalyst with reciprocally enhanced photo/electrocatalytic activity, ACS Sustainable Chem. Eng., 2019, 7, 3319–3328 CrossRef CAS.
- X. P. Liu, J. S. Chen, C. J. Mao, H. L. Niu, J. M. Song and B. K. Jin, A label-free photoelectrochemical biosensor for urokinase-type plasminogen activator detection based on a g-C3N4/CdS nanocomposite, Anal. Chim. Acta, 2018, 1025, 99–107 CrossRef CAS.
- Z. W. Wang, T. D. Nguyen, L. P. Yeo, C. K. Tan, L. Gan and A. L. Y. Tok, Periodic FTO IOs/CdS NRs/CdSe clusters with superior light scattering ability for improved photoelectrochemical performance, Small, 2020, 16, 1905826 CrossRef CAS.
- M. A. Mahadik, H. S. Chung, H. I. Ryu, W. S. Chae, M. Cho and J. S. Jang, Efficient way to assemble CdS nanorose-decorated CdSe-tetrakaidecahedron heterojunction photoanodes for high-photoelectrochemical performance, ACS Sustainable Chem. Eng., 2019, 7, 19708–19719 CrossRef CAS.
- H. S. Hao, S. C. Hao, H. M. Hou, G. L. Zhang, Y. X. Hou, Z. W. Zhang, J. R. Bi and S. Yan, A novel label-free photoelectrochemical immunosensor based on CdSe quantum dots sensitized Ho3+/Yb3+-TiO2 for the detection of Vibrio parahaemolyticus, Methods, 2019, 168, 94–101 CrossRef CAS.
- X. R. Zhang, S. G. Li, X. Jin and X. M. Li, Aptamer based photoelectrochemical cytosensor with layer-by-layer assembly of CdSe semiconductor nanoparticles as photoelectrochemically active species, Biosens. Bioelectron., 2011, 26, 3674–3678 CrossRef CAS.
- X. P. Liu, J. S. Chen, C. J. Mao, H. L. Niu, J. M. Song and B. K. Jin, Enhanced photoelectrochemical DNA sensor based on TiO2/Au hybrid structure, Biosens. Bioelectron., 2018, 116, 23–29 CrossRef CAS.
- M. Faisal, M. Jalalah, F. A. Harraz, A. M. El-Toni, A. Khan and M. S. Al-Assiri, Au nanoparticles-doped g-C3N4 nanocomposites for enhanced photocatalytic performance under visible light illumination, Ceram. Int., 2020, 46, 22090–22101 CrossRef CAS.
- B. Zhang, X. He, X. H. Ma, Q. H. Chen, G. C. Liu, Y. M. Zhou, D. Ma, C. Y. Cui, J. Ma and Y. J. Xin, In situ synthesis of ultrafine TiO2 nanoparticles modified g-C3N4 heterojunction
photocatalyst with enhanced photocatalytic activity, Sep. Purif. Technol., 2020, 247, 116932–116940 CrossRef CAS.
- P. F. Zhu, M. Hu, M. Duan, L. S. Xie and M. Y. Zhao, High visible light response Z-scheme Ag3PO4/g-C3N4/ZnO composite photocatalyst for efficient degradation of tetracycline hydrochloride: Preparation, properties and mechanism, J. Alloys Compd., 2020, 840, 155714–155725 CrossRef CAS.
- E. M. Hashem, M. A. Ahmed and M. F. A. Messih, Facile one-pot aqueous synthesis of highly soluble and luminescent CdSe quantum dots without nitrogen bubbling, CrystEngComm, 2020, 22, 4816–4822 RSC.
- S. Y. Li, M. Zhang, Z. H. Qu, X. Cui, Z. Y. Liu, C. C. Piao, S. G. Li, J. Wang and Y. T. Song, Fabrication of highly active Z-scheme Ag/g-C3N4-Ag-Ag3PO4 (110) photocatalyst for visible light photocatalytic degradation of levofloxacin with simultaneous hydrogen production, Chem. Eng. J., 2020, 382, 122394 CrossRef CAS.
- S. Patnail, D. P. Sahoo and K. M. Parida, Bimetallic co-effect of Au-Pd alloyed nanoparticles on mesoporous silica modified g-C3N4 for single and simultaneous photocatalytic oxidation of phenol and reduction of hexavalent chromium, J. Colloid Interface Sci., 2020, 560, 519–535 CrossRef.
- Q. Liu, Y. Yang, X. P. Liu, Y. P. Wei, C. J. Mao, J. S. Chen, H. L. Niu, J. M. Song, S. Y. Zhang, B. K. Jin and M. Jiang, A facile in situ synthesis of MIL-101-CdSe nanocomposites for ultrasensitive electrochemiluminescence detection of carcinoembryonic antigen, Sens. Actuators, B, 2017, 242, 1073–1078 CrossRef CAS.
- S. R. Ar, H. M. Wilson, B. M. Momin, U. S. Annapure and N. Jha, CdSe quantum dots modified thiol functionalized g-C3N4: Intimate interfacial charge transfer between 0D/2D nanostructure for visible light H2 evolution, Renewable Energy, 2020, 158, 431–443 CrossRef.
- N. Sharma, S. Sharma and R. Sharma, Dielectric and optical studies of CdSe nanoparticles: green synthesis, J. Mater. Sci.: Mater. Electron., 2020 DOI:10.1007/s10854-020-04214-9.
- A. Kumari, N. Thakur, J. Vashishtt and R. R. Singh, Structural, luminescent and antimicrobial properties of ZnS and CdSe/ZnS quantum dot structures originated by precursors, Spectrochim. Acta, Part A, 2020, 229, 117962–117972 CrossRef CAS.
- H. Y. Wang, Q. H. Zhang, H. S. Yin, M. H. Wang, W. J. Jiang and S. Y. Ai, Photoelectrochemical immunosensor for methylated RNA detection based on g-C3N4/CdS quantum dots heterojunction and Phos-tag-biotin, Biosens. Bioelectron., 2017, 95, 124–130 CrossRef CAS.
- K. Y. Zhang, S. Z. Lv, Z. Z. Lin and D. P. Tang, CdS: Mn quantum dot-functionalized g-C3N4 nanohybrids as signal generation tags for photoelectrochemical immunoassay of prostate specific antigen coupling DNAzyme concatamer with enzymatic biocatalytic precipitation, Biosens. Bioelectron., 2017, 95, 34–40 CrossRef CAS.
- C. Wang, T. Wu, X. Wang, Q. X. Wei, Y. Y. Wang, C. Y. Li and D. Sun, Ultrathin-layered carbon intercalated MoS2 hollow nanospheres integrated with gold nanoparticles for photoelectrochemical immunosensing of squamous cell carcinoma antigen, Sens. Actuators, B, 2019, 297, 126716 CrossRef CAS.
Footnote |
† Electronic supplementary information (ESI) available. See DOI: 10.1039/d0an01656j |
|
This journal is © The Royal Society of Chemistry 2021 |
Click here to see how this site uses Cookies. View our privacy policy here.