A simple self-adjuvanting biomimetic nanovaccine self-assembled with the conjugate of phospholipids and nucleotides can induce a strong cancer immunotherapeutic effect†
Received
11th August 2020
, Accepted 10th September 2020
First published on 11th September 2020
Abstract
Biomimetic nanoparticles have potential applications in many fields due to their favorable properties. Here, we developed a self-adjuvanting biomimetic anti-tumor nanovaccine, which was self-assembled with an amphiphilic conjugate synthesized with the phospholipids of 1,2-dioleoyl-sn-glycero-3-phosphoethanolamine (DOPE) and hydrophilic Toll-like receptor (TLR9) agonist CpG ODN. The nanovaccine could not only provide effective initial antigen stimulation and sustained long-term antigen supply with a controlled release, but also induce antigen cross-presentation via the MHC-I pathway initiating CD8+ T-cell responses. Moreover, the dense nucleotide shell around the nanovaccine could promote antigen endocytosis via various receptor-mediated pathways into dendritic cells. CpG ODN interacted with TLR9 triggering the cytokine secretion of TNF-α and IL-10, which further boosted the anti-tumor humoral and cellular immune responses, which led to a significant tumor suppressive effect and remarkable survival prolongation. So, this nanovaccine self-assembled with phospholipid–nucleotide amphiphiles can serve as a safe, simple and efficient approach for anti-tumor immunotherapy.
1. Introduction
Nanovaccines have been investigated in recent years as an emerging field in cancer immunotherapy owing to their ability to cross biological barriers, prolong circulation times, and induce an enhanced long-lasting protective immune effect.1–3 Generally, nanovaccine carriers are required to deliver antigens and/or adjuvants to overcome intrinsic difficulties of functional cargos and trigger delightful immune responses.4–6 Many studies reported that the co-delivery of antigens and adjuvants of CpG ODN, whether encapsulated in or located outside the nanocarriers, could promote strong immune responses and enhance the anti-tumor effect.7,8 Recently, it has been reported that nucleic acids (CpG ODN) arranged in a dense spherical spatial form (SNAs) on a core made of gold or other polymers, can endow them with unique properties, such as rapid uptake through a receptor-mediated mechanism and efficient protection against nuclease degradation, and significantly enhance the immune response.9–12 However, the preparation of popular polymeric nanocarriers is complicated and traditional core polymers have potential physiological toxicity.6,13–16
Biomimetic NPs to mimic the chemical and structural features of biological systems possess distinct properties, including favorable biocompatibility, extended circulation and targeted drug delivery. Here, we report a simple self-adjuvanting biomimetic nanovaccine (NaV) self-assembled with the conjugate of phospholipids and nucleotides, which had a dense nucleotide shell similar to SNAs. In this study, hydrophobic biomimetic phospholipids of DOPE and hydrophilic adjuvanting Toll-like receptor (TLR9) agonist CpG ODN were exploited and covalently linked together. The resultant amphiphilic molecules similar to the phospholipid bilayer of the cell membrane self-assembled forming nanocapsules. So, the nanovaccine mimicked both the chemical and structural characteristics of cells displaying good biocompatibility. Furthermore, CpG ODN not only as adjuvants enhanced the immune responses, but also the dense nucleotide shell formed with CpG ODN promoted antigen endocytosis via various receptor-mediated pathways and subsequently boosted the anti-tumor humoral and cellular immune responses. Therefore, the biomimetic nanovaccine offered a simple, safe and effective strategy for cancer treatment.
2. Materials and methods
2.1 Preparation of self-adjuvanting biomimetic nanovaccines
The preparation of self-adjuvanting biomimetic nanovaccines involved three steps. Briefly, DOPE (Sigma-Aldrich Co., USA) was pyridyldithiol-activated by 3-(2-pyridyldithio) propionic acid N-hydroxysuccinimide ester (SPDP). Then, the activated DOPE was conjugated with thiol terminated CpG ODN (Sangon Biotech Ltd, China) via a disulfide bond in the presence of a phase transfer catalyst. Finally, the resulted amphiphiles of DOPE-S-S-CpG ODN and ovalbumin (OVA, a model antigen, Sigma-Aldrich Co., USA) solutions were mixed and stirred at 25 °C for the formation of self-adjuvanting biomimetic nanovaccines (named NaVs) with self-assembly. The NaVs were washed with distilled water and lyophilized.
2.2 Characterization of DOPE-S-S-CpG ODN amphiphiles and NaVs
The conjugate of DOPE-S-S-CpG ODN was confirmed by agarose gel electrophoresis. The particle sizes and zeta potential of the NaVs were analyzed using Zetasizer Nano ZP (Malvern Instruments Ltd, UK). The redox-responsive properties of the NaVs were studied through observing the changes in the size after reaction with excess DL-dithiothreitol (DTT). To investigate the stability of the NaVs, the size of the NaVs was measured every five days until the nanoparticles aggregated. The morphology of the NaVs was observed using transmission electron microscopy (TEM) (Tecnai-F20, FEI, the Netherlands).
The OVA loading capacity of the NaVs was determined using an Enhanced-BCA Protein Assay Kit. To evaluate the release behavior of antigens from the NaVs in vitro, 5 mg of lyophilized NaV powder was dissolved with 1 mL of phosphate buffered saline (PBS, pH7.4) and placed in a dialysis bag (molecular weight: 50
000 Da) which was immersed in 10 ml of PBS at 37 °C, and the OVA concentration in dialysate was measured with BCA kits at regular intervals.
2.3 Immunization studies in vitro
2.3.1 Cytotoxicity assessment.
Bone marrow-derived dendritic cells (BMDCs) were derived from C57BL/6 mouse femur and cultured with IL-4 and GM-CSF (Peprotech, USA) for 6 days before further experiments. BMDCs with a density of 1 × 105 cells per mL were co-incubated with the NaVs at a series of concentrations for 24 h, and the cytotoxicity of NaVs was evaluated using CCK-8 kits (Dojindo Molecular Technologies Inc., Japan).
2.3.2 Antigen uptake in BMDCs.
To observe whether the NaVs could facilitate the antigen uptake by BMDCs, after co-incubation several times with free OVA and NaVs (each concentration of FITC-OVA: 20 μg mL−1) for 8 h at 37 °C, BMDCs were washed using immunol staining wash buffer to remove the extracellular FITC-OVA or NaVs. Afterwards, the BMDCs were labeled with 4,6-diamidino-2-phenylindole (DAPI) and imaged with a laser scanning confocal microscope (Zeiss LSM 800, Germany).
2.3.3 Endocytic mechanism of NaVs in BMDCs.
For further exploring the endocytic pathways of DCs to NaVs, we used several uptake inhibitors to pretreat cells separately to block the corresponding endocytic pathway. Briefly, BMDCs were co-cultured with fucoidan (FCD, 10 μg mL−1), chlorpromazine (CPM, 10 μg mL−1) or methyl-β-cyclodextrin (MBCD, 10 μg mL−1) for 1 hour, respectively. Afterwards, the pretreated cells were co-incubated with free FITC-OVA or NaVs (containing FITC-OVA) for hours while untreated cells were used as a negative control. Then the quantity of FITC-OVA in BMDCs was detected using a flow cytometer (BD Biosciences, CA, USA) and the reduction of the antigen uptake of the NaVs compared to that of the negative control group was calculated.
2.3.4 Effect of NaVs on BMDCs in vitro.
In order to confirm whether the NaVs could promote BMDC activation and antigen cross-presentation, immature BMDCs were co-cultured with PBS, free OVA, OVA + CpG and NaVs at 37 °C in a 5% CO2 cell incubator for 6 h, respectively. Then the BMDCs were subsequently stained with fluorescence-labeled antibodies against CD11c, CD86, CD40 and OVA257–264 peptide SIINFEKL (Thermo Fisher Scientific Inc., USA). Data were collected and plotted using a flow cytometer. Meanwhile, the cytokines of tumor necrosis factor-α (TNF-α) and interleukin-6 (IL-6) released in culture supernatants were quantified with ELISA kits (Thermo Fisher Scientific Inc., USA).
2.4 Immunization studies in vivo
2.4.1 Antigen depot effect.
Six-week-old female C57BL/6 mice (n = 5) were used to study the release behavior of the NaVs in vivo. 100 μL suspensions of the NaVs and free OVA (each containing 20 μg Cy7-labeled OVA) were intramuscularly injected into the mice, respectively. Then Cy7 fluorescence signals of the immunization site were detected at predetermined intervals with a Maestro imaging system (CRI, USA) and the mean fluorescence intensity (MFI) was calculated at each checkpoint.
2.4.2 Tumor challenge and therapeutic effect.
All animal procedures were performed in accordance with the Guidelines for Care and Use of Laboratory Animals of the Chinese Academy of Medical Science and Peking Union Medical College and were approved by the Animal Ethics Committee of the Chinese Academy of Medical Science and Peking Union Medical College. After getting acclimated to the SPF barrier environment, healthy female C57BL/6 mice were used to establish xenograft tumor models. E·G7-OVA (5 × 105) cells in the logarithmic growth phase were injected subcutaneously into the right back of the mice. Until the tumor reached 5 mm in diameter, immunotherapy was performed on the mice. Tumor-bearing mice were divided into 4 groups, a 50 μL suspension of free OVA, OVA + CpG, and NaVs (each formulation containing 20 μg OVA) was respectively injected, using PBS as a negative control. The immunization was performed three times at intervals of every other week.
To further evaluate the anti-tumor effect of the NaVs, the length and width of mouse tumors were recorded with digital vernier calipers every two days, while observing the mental state of the mice. Tumor volume (mm3) was calculated according to the following formula: (length × width2)/2. Two days after the last immunization, the mice were sacrificed and their peripheral blood, tumors and spleens were collected immediately for the following immunological experiments. The solid tumor tissues collected from tumor-bearing mice were stained with hematoxylin and eosin (H&E) and TUNEL apoptosis kits (Beyotime Biotechnology, China) to observe the influence of the NaVs on the necrosis and apoptosis of tumor cells.
2.4.3 Secretion of OVA-specific antibodies.
Before the mice were dislocated, the blood was drawn from the postorbital venous plexuses and blood serum was isolated through centrifugation twice at 3000 rpm for 10 min. Then, the level of OVA-specific antibodies including IgG, IgG1, and IgG2a secreted in blood was determined using ELISA kits.
2.4.4 T Cell immune responses and immune memory.
The spleens from the immunized mice were made into a single cell suspension and the lymphocytes were extracted. Some of the splenocytes were co-incubated with anti-mouse antibodies against CD4, CD8 and CD3, and detected using a flow cytometer to analyze the proliferation of T cells. The leftover cells were re-stimulated with OVA for 3 days and stained with CD8, CD4, CD62L and CD44 antibodies labeled with different fluorescence to analyze the generation of memory T cells. Meanwhile, the cell supernatant was harvested and the secretion of TNF-α and interleukin-10 (IL-10) was evaluated using ELISA kits to further confirm the type of immune response.
2.5 Statistical analysis
Data were expressed as mean ± standard deviation (SD). The differences were assessed using ANOVA or the Student's t-test and the Tukey's post-test. P value not more than 0.05 was considered significant.
3. Results and discussion
3.1 NaV preparation and characterization studies
DOPE and CpG ODN were covalently linked using SPDP as the crosslinking agent. The successful synthesis of DOPE-S-S-CpG ODN was confirmed by agarose gel electrophoresis (Fig. S1†). The resulting DOPE-S-S-CpG ODN and OVA (as model antigen) self-assembled forming NaVs. The morphology of the NaVs was characterized by TEM and the image showed that the formulations were uniform nanocapsules (Fig. 1A). The diameter of the NaVs determined with dynamic light scattering (DLS) was 192.4 ± 0.66 nm, while that of the blank nanocapsule without OVA (named SNCs) was 138.2 ± 0.90 nm (Fig. 1B and Table S1†). The size of the NaVs remained stable in PBS solution for 45 days approximately (Fig. 1C). The loading capacity of OVA in NaVs determined by an Enhanced-BCA Protein Assay Kit was (26.4 ± 0.77)%. OVA had a burst release of 30% from the NaVs during the first two days under conditions that mimic the intracellular reducing environment (4 mM DTT in 7.4 pH buffer) followed by a controlled release reaching about 65% in 25 days, which was faster than the release behavior of OVA (44.99 ± 1.69)% under conditions without DTT (Fig. 1D), indicating that the NaVs did possess redox-responsive properties allowing antigens’ quick release after uptake into cells, and the nanovaccines could provide strong initial antigen stimuli and long-term sustained antigen exposure to immune cells, which was important for eliciting powerful immune responses.
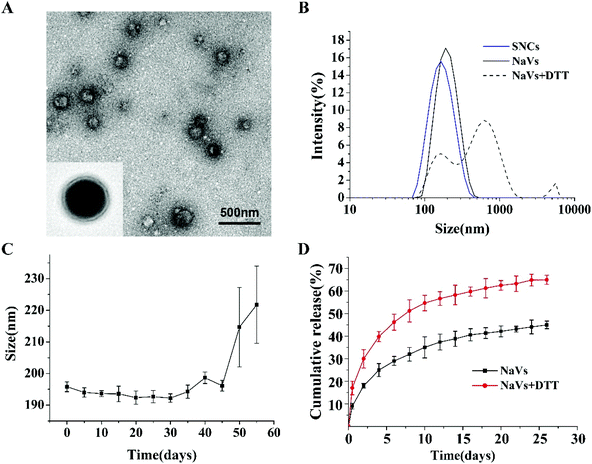 |
| Fig. 1 (A) Typical TEM images of NaVs. (B) The particle distribution of different formations, and (C) stability study of NaVs in vitro, and (D) in vitro OVA release profile from NPs with/without the DTT reaction in 7.4 pH buffer. | |
3.2 Immunization studies in vitro
3.2.1 Cytotoxicity assessment.
The cell viability of the BMDCs treated with the NaVs at various concentrations was assessed by the CCK-8 assay. According to the experimental result, even when the concentration of OVA was up to 60 μg ml−1, the cell viability remained high (102.1 ± 5.77)%, which demonstrated that these nanovaccines showed excellent safety to BMDCs (Fig. 2A).
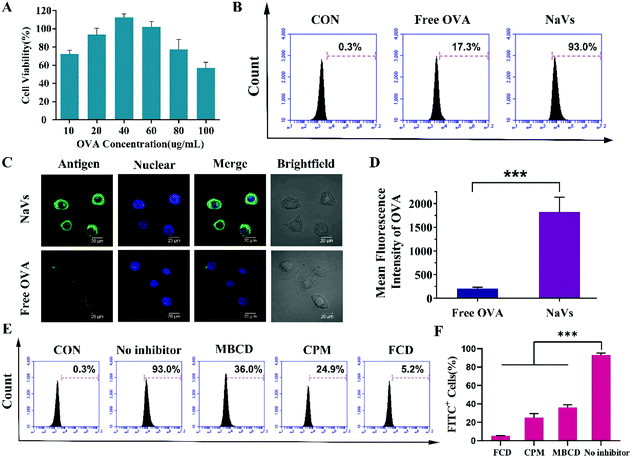 |
| Fig. 2 Studies on cytotoxicity and cellular uptake pathways of nanoparticles. (A) Cell viability at different concentrations of OVA. (B) Representative flow cytometry analysis of the cellular uptake of NaVs and free OVA. (C) CLSM images of BMDCs after incubation with free FITC-OVA and NaVs and (D) representative image of the mean fluorescence intensity analysis of OVA ingested by cells, analyzed using ImageJ software. (E) A representative flow cytometry profile of the nanoparticle uptake by DCs with different inhibitors, and (F) representative fluorescence intensity histogram. *p < 0.05, **p < 0.01, ***p < 0.001. | |
3.2.2 Endocytosis mechanism of NaVs.
We next investigated the antigen uptake capacity of the NaVs. After co-incubation with free FITC-OVA or NaVs for 8 h respectively, images taken with a confocal fluorescence microscope showed that a much more significant OVA signal appeared in BMDCs treated with the NaVs than with the free OVA group. Fluorescence analysis of the representative image using the ImageJ software indicated that the mean fluorescence intensity (MFI) of OVA in the NaV group far exceeded that in the free OVA group, which was enhanced about 6-fold. The results of the flow cytometry analysis also demonstrated that the NaVs facilitated the uptake of antigens in BMDCs (93.0% vs. 17.3%) (Fig. 2B–D).
Although Chad A. Mirkin et al. reported that high-density oligonucleotides on the surface of nanoparticles could bind scavenger receptors on the surface of macrophages and promote nanoparticle uptake into macrophages,12 in an attempt to further explore the endocytic pathways and investigate the mechanism of nanoparticle uptake in DCs that is important for initiating immune responses for vaccines, we pretreated BMDCs with methyl-β-cyclodextrin (MBCD), chlorpromazine (CPM) and fucoidan (FCD), respectively. MBCD can remove cholesterol from the cell membrane effectively inhibiting caveolin-dependent endocytosis, CPM can block the formation of clathrin-coated pits, while FCD is a pharmacological inhibitor of class A scavenger receptor (SR-A).17–20 As shown in Fig. 2E and F, compared to untreated cells, FCD led to a drastic reduction of the antigen uptake of the NaVs (dropped up to 5.2%), MBCD reduced the uptake of cells to 36.0%, while CPM caused an uptake decrease to 24.9%. All the data suggested that the NaVs internalized into BMDCs through multiple receptor-mediated endocytosis pathways, but mainly mediated by SR-A. Altogether, the NaVs constructed with the conjugate of adjuvant CpG ODN and biomimetic material DOPE having a “3D” structure could load antigens in the cavity of nanocapsules as vaccine delivery nanocarriers, and the high-density nucleotides on the surface of nanocapsules could bind the various receptors on the surface of BMDCs and promote antigen endocytosis circumventing the drawbacks of free antigens, which in turn facilitated the CpG ODN interaction with the TLR9 receptor in endosomes and activated antigen presenting cells.21–27
3.2.3 BMDC activation and antigen cross-presentation.
In order to further confirm whether the NaVs could promote BMDC maturation and activation, which was essential for the initiation of antigen-specific immune responses, we co-incubated immature BMDCs with PBS, free OVA, free OVA + CpG and NaVs for 6 h, respectively. The expression level of co-stimulatory molecules CD86 and CD40 on BMDCs treated with the NaVs was nearly doubled compared to other groups (Fig. 3A and B). Moreover, we demonstrated that the SIINFEKL OVA-CD8+ epitope presented by MHC class I H-2Kb molecules on the surface of BMDCs treated with the NaVs increased nearly 4 times compared with PBS groups (Fig. 3C), indicating that the NaVs could promote antigen cross-presentation via the MHC-I pathway which would subsequently initiate CD8+ T cell responses which are critical for cancer treatment, while exogenous antigens are mainly presented via the MHC-II peptide complex.28,29 Furthermore, the results of the enzyme-linked immunosorbent assay (ELISA) of cytokines showed that the BMDCs co-incubated with the NaVs had a remarkably enhanced level of TNF-α compared with other groups (Fig. 3D) and IL-6 (Fig. 3E); in particular, IL-6 was enhanced up to ten-fold compared to the PBS group. IL-6 can stimulate the production of chemokines and promote the activation and proliferation of B cells,30,31 while TNF-α plays important roles in stimulating the T cell expansion and inducing potent tumor-specific CTLs.32,33 Therefore, these in vitro results showed that the NaVs could promote DC maturation, activation and antigen cross-presentation favoring the induction of antigen-specific CTL-polarizing immune responses.
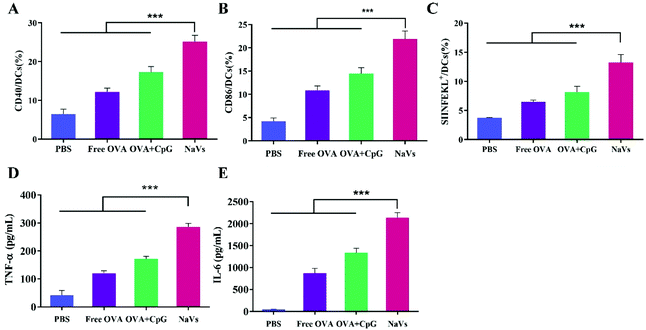 |
| Fig. 3 Effects of different components on the antigen cross-presentation and activation of BMDCs. Representative flow cytometry images of (A) CD40+, (B) CD86+ and (C) SIINFEKL+ cells. Expression levels of (D) TNF-α and (E) IL-6 in supernatants were determined with ELISA assays, after BMDCs were treated with PBS, free OVA, OVA + CpG and NaVs. *p < 0.05, **p < 0.01, ***p < 0.001. | |
3.3 Immunization studies in vivo
3.3.1 Antigen release behavior of NaVs in vivo.
Encouraged by the results in vitro, we next sought to examine the antigen depot effect and release studies of NaVs in vivo using the Maestro imaging system. As shown in Fig. 4A, the fluorescence Cy7 intensity signals of free OVA reached the zenith about 6 hours after injection and then decreased gradually until they were not detected at 120 h. In contrast, the initial fluorescence intensity of the NaVs increased relatively slowly and reached a peak value at 12 h and still 25% remained at the sites after 7 days (Fig. 4B). The above results indicated that the NaVs could provide strong initial immune stimulation and sustained long-term antigen supply in vivo.
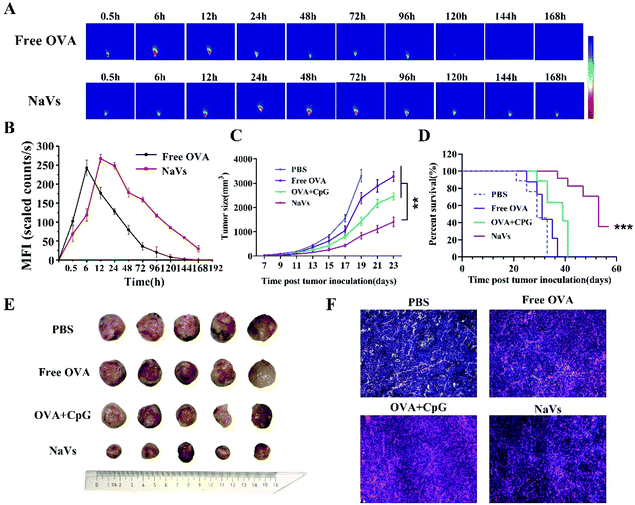 |
| Fig. 4 (A) Antigen release behavior of NaVs at the injection site. Representative fluorescence images of different formulations at different points in time after injection. (B) Average fluorescence intensity at the injected site within a predetermined time period. (C) Average tumor growth curves were recorded and plotted. (D) Survival of mice treated with PBS, free OVA, OVA + CpG or NaVs. (E) Tumors harvested from tumor-bearing mice after different component treatments, and (F) H&E staining of tumor tissues obtained from mice immunized with different formulations. *p < 0.05, **p < 0.01, ***p < 0.001. | |
3.3.2 Therapeutic effect of NaVs.
We then established E·G7-OVA tumor models and further examined the in vivo therapeutic effect of the NaVs. As shown in Fig. 4C and E, compared with the PBS group, NaV vaccination could significantly suppress tumor growth, OVA + CpG moderately inhibited tumor growth, while free OVA only offered a slight tumor growth inhibition. In terms of survival prolongation, most of the animals immunized with the NaVs survived over 40 days, while the mice treated with other groups almost died within 41 days (Fig. 4D). So, NaV vaccination could offer a significant protection and remarkably prolong the life span of immunized mice. Moreover, the results of H&E staining (Fig. 4F) and TUNEL apoptosis of tumor tissues (Fig. S2†) showed that, compared with other groups, NaV vaccination could effectively promote the necrosis and apoptosis of tumor cells.
3.3.3 OVA-specific antibody production.
In order to further confirm the type of immune response triggered by the NaVs and confirm the direction of lymphocyte differentiation, we first analyzed the type and amount of IgG in the blood of tumor-bearing mice which reflect the subtype of Th cells. It is generally acknowledged that the level of IgG1 antibodies is associated with Th2 immune response, whereas the production of IgG2a antibodies is characterized for Th1 responses.34,35 As shown in Fig. S3,† the mice immunized with the NaVs exhibited the highest OVA-specific IgG levels among all the groups, especially characterized with a high IgG2a/IgG1 ratio as well, which meant NaV polarized immune response towards Th1. In addition, the Th1-dominated responses have potential for the induction of CTL responses to eliminate antigen-specific tumor cells.36 Therefore, the NaVs not only have the property of significantly inducing the production of IgG antibodies, but also have the trend of eliciting Th1-type cell immune responses.
3.3.4 T Immune cell activation.
For gaining a more in-depth understanding of the immune responses and relative mechanism of the NaVs in vivo, the spleen lymphocytes of the immunized mice were stained with anti-mouse APC-CD3, FITC-CD4 and Cy5.5-CD8 antibodies. As shown in Fig. 5A, compared to other groups, the percentage of CD4+ T cells from the mice treated with the NaVs greatly enhanced from 8.9% to 26.5% (Fig. 5B), while the percentage of CD8+ T cells increased from 5.4% to 13.6% (Fig. 5C and D). Altogether, the NaVs could significantly promote the expansion of CD4+ T cells and CD8+ cells in the immune system of tumor-bearing mice.
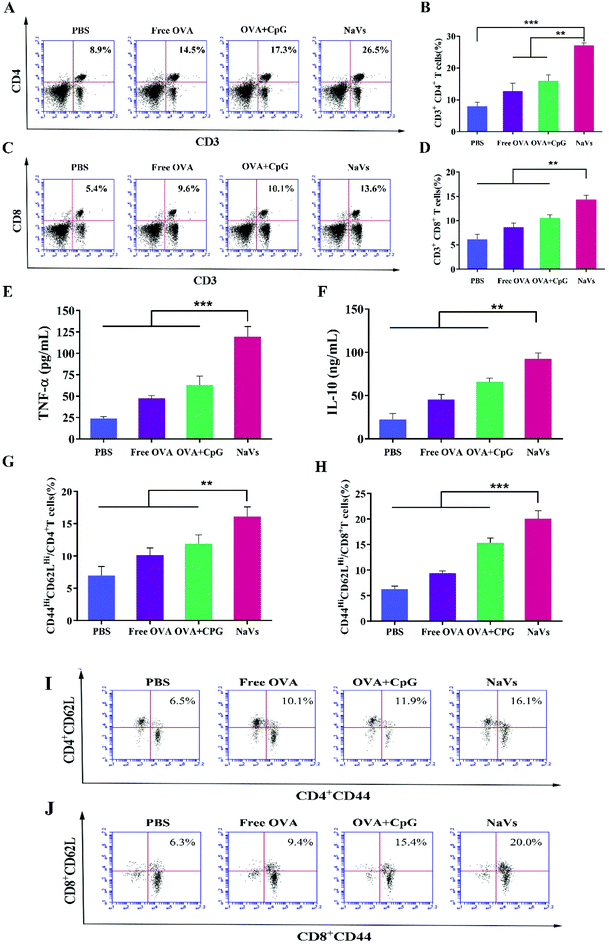 |
| Fig. 5 T immune cell activation and representative FACS plots. (A) Representative FACS plots and (B) percentage of CD3+CD4+ T cells and (C and D) CD3+CD8+ T cells were plotted for mice of different groups, followed by the histograms of (E) TNF-α and (F) IL-10. The type and proportion of memory T cells in splenocytes after stimulation were detected and analyzed, and the proportion of central memory T cells in (G) CD4+ T cell population or (H) CD8+ T cell population and their representative FACS plots are shown. *p < 0.05, **p < 0.01, ***p < 0.001. | |
Furthermore, the cytokine levels of the supernatant of splenocytes after antigen re-stimulation in vitro were detected. As shown in Fig. 5E, the NaVs increased the cytokine level of TNF-α approximately 4 times compared with the PBS group, which is consistent with the in vitro results. The NaVs also significantly elevated the level of cytokine IL-10 (Fig. 5F). IL-10 as an anti-inflammatory factor can specifically limit the Th17 inflammation to eliminate the protumor environment and promote Th1-type anti-tumor immunity to recognize and eliminate tumors cells, and hence inhibit the tumor development and metastasis.37,38 It can also activate NK cells and CD8+T cells directly or indirectly to enhance anti-tumor effects and contribute to the formation of immune memory.39 As stated in the previous study, here the cytokines of TNF-α and IL-10 were upregulated, mainly mediated by NF-κB and AP-1 activation through mitogen-activated protein kinase (MAPK) and nuclear factor kappa-B inducing kinase-IkB kinase-IkB (NIK-IKK-IkB) pathways which resulted from TLR-9 receptor binding with dense CpG ODNs after the NaVs were internalized into endosomes.5,40 Altogether, we conclude that the NaVs could enhance both humoral and cellular immune responses.
3.3.5 Memory T cell immune response.
An important principle of tumor nanovaccine is to stimulate the immune system producing long-lasting antigen-specific immune memory, which prevents tumor formation and recurrence. So, we examined the memory T cell immune responses. CD44 is the most reliable marker of mouse memory cells and generally is used to define the primary and memory T cells, while CD62L, an important adhesion molecule on central memory T cells, is usually used to distinguish between central memory T cells (CD44HiCD62LHi) and effector memory T cells (CD44HiCD62LLo).41–43 After the spleen lymphocytes from mice treated with the NaVs were re-stimulated by the antigens, TCM proliferated in a large amount in both CD4+T (PBS 6.5% vs. NaVs 15.9%) cells and CD8+T cells (PBS 8.9% vs. NaVs 18.2%) (Fig. 5G and H). These data suggested that the NaVs could effectively induce the production and proliferation of TCM, so that the body's immune system can maintain the memory of tumor antigens and provide long-term immune surveillance.
4. Conclusions
In summary, as shown in Fig. 6, we developed a self-adjuvanting biomimetic nanovaccine self-assembled with the amphiphilic conjugate synthesized with hydrophobic phospholipids and hydrophilic TLR9 agonist CpG ODN. The nanovaccine with a 3D structure could load antigens in the cavity, provide effective initial immune stimulation and sustained long-term antigen supply, and promote antigen cross-presentation via the MHC-I pathway initiating CD8+ T-cell responses. Moreover, the dense nucleotide shell around the nanovaccine could promote antigen endocytosis via various receptor-mediated pathways into dendritic cells. The CpG ODN interaction with TLR9 triggering the cytokine secretion of TNF-α and IL-10 further boosted the anti-tumor humoral and cellular immune responses as well as generated effective immune memory, which led to a significant tumor suppressive effect and remarkable survival prolongation. So, the self-adjuvanting biomimetic nanovaccine can serve as a great promising immunotherapeutic approach for tumor treatment. Moreover, the method reported here could be applied to produce various types of nanovaccines for the treatment of infectious diseases though loading different antigens in the cavity of the capsule, or to prepare siRNA nanocarriers for gene therapy through replacing the CpG ODN shell around the nanovaccine with siRNA.
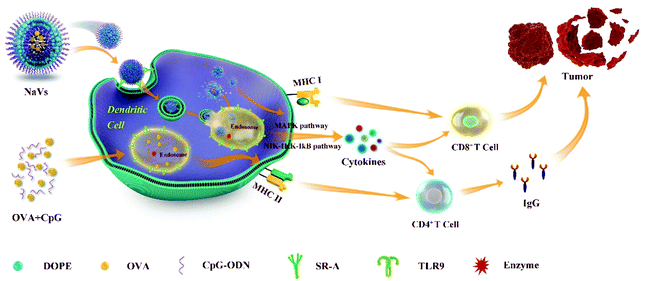 |
| Fig. 6 Illustration of the NaVs anti-tumor immune effect mechanism. | |
Conflicts of interest
The authors declare no conflict of interest.
Acknowledgements
This work was supported by the Natural Science Foundation of China (No. 31870920), CAMS Innovation Fund for Health and Longevity Pilot Project (Youth Award Program, 2019-RC-HL-015) and CAMS Innovation Fund for Medical Sciences (CAMS-I2M-3-004).
References
- S. Acharya and S. K. Sahoo, Adv. Drug Delivery Rev., 2011, 63, 170–183 CrossRef CAS.
- K. Shao, S. Singha, X. Clemente-Casares, S. Tsai, Y. Yang and P. Santamaria, ACS Nano, 2015, 9, 16–30 CrossRef CAS.
- J. Zhou, A. V. Kroll, M. Holay, R. H. Fang and L. Zhang, Adv. Mater., 2019, e1901255, DOI:10.1002/adma.201901255.
- G. Zhu, F. Zhang, Q. Ni, G. Niu and X. Chen, ACS Nano, 2017, 11, 2387–2392 CrossRef CAS.
- M. Luo, H. Wang, Z. Wang, H. Cai, Z. Lu, Y. Li, M. Du, G. Huang, C. Wang, X. Chen, M. R. Porembka, J. Lea, A. E. Frankel, Y.-X. Fu, Z. J. Chen and J. Gao, Nat. Nanotechnol., 2017, 12, 648–654 CrossRef CAS.
- S. Singha, K. Shao, K. K. Ellestad, Y. Yang and P. Santamaria, ACS Nano, 2018, 12, 10621–10635 CrossRef CAS.
- T. Kang, Y. Huang, Q. Zhu, H. Cheng, Y. Pei, J. Feng, M. Xu, G. Jiang, Q. Song, T. Jiang, H. Chen, X. Gao and J. Chen, Biomaterials, 2018, 164, 80–97 CrossRef CAS.
- Y. Hu, L. Lin, J. Chen, A. Maruyama, H. Tian and X. Chen, Biomaterials, 2020, 252, 120114 CrossRef CAS.
- C. A. Mirkin, R. L. Letsinger, R. C. Mucic and J. J. Storhoff, Nature, 1996, 382, 607–609 CrossRef CAS.
- C. A. Mirkin and S. H. Petrosko, Clin. Chem., 2018, 64, 971–972 CrossRef CAS.
- J. R. Ferrer, A. J. Sinegra, D. Ivancic, X. Y. Yeap, L. Qiu, J.-J. Wang, Z. J. Zhang, J. A. Wertheim and C. A. Mirkin, ACS Nano, 2020, 14, 1682–1693 CrossRef CAS.
- C. H. J. Choi, L. Hao, S. P. Narayan, E. Auyeung and C. A. Mirkin, Proc. Natl. Acad. Sci. U. S. A., 2013, 110, 7625–7630 CrossRef CAS.
- Y. Wang, L. Miao, A. Satterlee and L. Huang, Adv. Drug Delivery Rev., 2015, 87, 68–80 CrossRef CAS.
- L. Giodini, F. L. Re, D. Campagnol, E. Marangon, B. Posocco, E. Dreussi and G. Toffoli, Nanomedicine, 2017, 13, 583–599 CrossRef CAS.
- Y. Xin, M. Huang, W. W. Guo, Q. Huang, L. Z. Zhang and G. Jiang, Mol. Cancer, 2017, 16, 134 CrossRef.
- D. Shi, N. M. Bedford and H.-S. Cho, Small, 2011, 7, 2549–2567 CrossRef CAS.
- S. De Koker, B. G. De Geest, S. K. Singh, R. De Rycke, T. Naessens, Y. Van Kooyk, J. Demeester, S. C. De Smedt and J. Grooten, Angew. Chem., Int. Ed., 2009, 48, 8485–8489 CrossRef CAS.
- P. C. Patel, D. A. Giljohann, W. L. Daniel, D. Zheng, A. E. Prigodich and C. A. Mirkin, Bioconjugate Chem., 2010, 21, 2250–2256 CrossRef CAS.
- J. Rejman, A. Bragonzi and M. Conese, Mol. Ther., 2005, 12, 468–474 CrossRef CAS.
- D. A. Kuhn, D. Vanhecke, B. Michen, F. Blank, P. Gehr, A. Petri-Fink and B. Rothen-Rutishauser, Beilstein J. Nanotechnol., 2014, 5, 1625–1636 CrossRef.
- N. Hanagata, Int. J. Nanomed., 2017, 12, 515–531 CrossRef CAS.
- Y. M. Murad and T. M. Clay, Biodrugs, 2009, 23, 361–375 CrossRef CAS.
- H. Hemmi, O. Takeuchi, T. Kawai, T. Kaisho, S. Sato, H. Sanjo, M. Matsumoto, K. Hoshino, H. Wagner, K. Takeda and S. Akira, Nature, 2000, 408, 740–745 CrossRef CAS.
- R. Kuai, X. Sun, W. Yuan, L. J. Ochyl, Y. Xu, A. Hassani Najafabadi, L. Scheetz, M.-Z. Yu, I. Balwani, A. Schwendeman and J. J. Moon, J. Controlled Release, 2018, 282, 131–139 CrossRef CAS.
- G. N. Barber, Immunol. Rev., 2011, 243, 99–108 CrossRef CAS.
- F. Takeshita, I. Gursel, K. J. Ishii, K. Suzuki, M. Gursel and D. M. Klinman, Semin. Immunol., 2004, 16, 17–22 CrossRef CAS.
- J. Yue, R. M. Pallares, L. E. Cole, E. E. Coughlin, C. A. Mirkin, A. Lee and T. W. Odom, ACS Appl. Mater. Interfaces, 2018, 10, 21920–21926 CrossRef CAS.
- O. P. Joffre, E. Segura, A. Savina and S. Amigorena, Nat. Rev. Immunol., 2012, 12, 557–569 CrossRef CAS.
- A. Gardner and B. Ruffell, Trends Immunol., 2016, 37, 855–865 CrossRef CAS.
- S. I. Grivennikov and M. Karin, Ann. Rheum. Dis., 2011, 70(Suppl 1), i104–i108 CrossRef CAS.
- S. A. Jones and B. J. Jenkins, Nat. Rev. Immunol., 2018, 18, 773–789 CrossRef CAS.
- F. Balkwill, Cancer Metastasis Rev., 2006, 25, 409–416 CrossRef CAS.
- F. Balkwill, Nat. Rev. Cancer, 2009, 9, 361–371 CrossRef CAS.
- J. Liu, X. Liu, Y. Han, J. Zhang, D. Liu, G. Ma, C. Li, L. Liu and D. Kong, ACS Appl. Mater. Interfaces, 2018, 10, 30983–30993 CrossRef CAS.
- K. Heeg and S. Zimmermann, Int. Arch. Allergy Immunol., 2000, 121, 87–97 CrossRef CAS.
- S. Romagnani, Ann. Allergy, Asthma, Immunol., 2000, 85, 9–18 CrossRef CAS.
- M. Saraiva and A. O'Garra, Nat. Rev. Immunol., 2010, 10, 170–181 CrossRef CAS.
- M. Oft, Cancer Immunol. Res., 2014, 2, 194–199 CrossRef CAS.
- A. Naing, J. R. Infante, K. P. Papadopoulos, I. H. Chan, C. Shen, N. P. Ratti, B. Rojo, K. A. Autio, D. J. Wong, M. R. Patel, P. A. Ott, G. S. Falchook, S. Pant, A. Hung, K. L. Pekarek, V. Wu, M. Adamow, S. McCauley, J. B. Mumm, P. Wong, P. Van Vlasselaer, J. Leveque, N. M. Tannir and M. Oft, Cancer Cell, 2018, 34, 775–791 CrossRef CAS.
- H.-M. Wu, J. Wang, B. Zhang, L. Fang, K. Xu and R.-Y. Liu, Life Sci., 2016, 161, 51–59 CrossRef CAS.
- S. M. Kaech and W. Cui, Nat. Rev. Immunol., 2012, 12, 749–761 CrossRef CAS.
- F. Sallusto, J. Geginat and A. Lanzavecchia, Annu. Rev. Immunol., 2004, 22, 745–763 CrossRef CAS.
- L. Liu, P. Ma, H. Wang, C. Zhang, H. Sun, C. Wang, C. Song, X. Leng, D. Kong and G. Ma, J. Controlled Release, 2016, 225, 230–239 CrossRef CAS.
Footnote |
† Electronic supplementary information (ESI) available. See DOI: 10.1039/d0bm01333a |
|
This journal is © The Royal Society of Chemistry 2021 |
Click here to see how this site uses Cookies. View our privacy policy here.