DOI:
10.1039/D0BM01519A
(Paper)
Biomater. Sci., 2021,
9, 221-237
An ROS-sensitive tegafur-PpIX-heterodimer-loaded in situ injectable thermosensitive hydrogel for photodynamic therapy combined with chemotherapy to enhance the tegafur-based treatment of breast cancer †
Received
8th September 2020
, Accepted 29th October 2020
First published on 31st October 2020
Abstract
Chemotherapy and surgery are commonly used clinical treatments for breast cancer. However, unsatisfactory therapeutic effects, unavoidable side effects, and the removal of breast tissue during surgery are still major obstacles to be overcome during breast-cancer treatment. To overcome the aforementioned issues, a biomimetic and thermosensitive hydrogel encapsulating reactive oxygen species (ROS)-sensitive tegafur (TF)-protoporphyrin IX (PpIX) heterodimers (TTP) was prepared aimed at the strategy of synergizing chemotherapy and photodynamic therapy. TF was grafted onto the photosensitizer PpIX through ROS-sensitive thioether bonds. Under 630 nm laser irradiation, the ROS concentration in tumors was increased to help drug release. The “on-demand” drug release maximized the therapeutic effects of TF and effectively reduced its toxicity. Particularly, the ROS concentration was increased, and it was expected that the tumor-cell-killing abilities of ROS could be exploited. A hydrogel formed from temperature-sensitive chitosan and silk sericin was selected as a drug carrier, which formed a drug reservoir intratumorally after intratumoral injection. Intratumoral injection gave the drug precise and long-term release behavior, which allowed for the clever avoidance of various physical and biological barriers in vivo. In vivo and in vitro studies prove that the use of this TTP hydrogel provides a new attempt to overcome the many difficulties related to breast-cancer treatment simultaneously, and this study opens up new paths for breast-cancer treatment.
1. Introduction
Tumors are a medical problem in the field of public health worldwide. According to statistics from 2018, China was ranked first in the world in terms of tumor morbidity and mortality among the global cancer-affected population.1 In spite of the considerable effort devoted to the treatment of tumors, surgery is still the most direct way to treat various malignant tumors. Unfortunately, not all tumors are suitable for surgical treatment, such as brain tumors and advanced breast cancer. A survey showed that more than half of the cancer patients in China are over 60 years old.2 Organ weakness, age-related diseases, and slow recovery after surgery can cause elderly cancer patients to face greater surgical risks. Breast-cancer patients, in order to maintain breast integrity, are more inclined to choose chemotherapy and radiotherapy to treat breast cancer.3–5
Chemotherapy and radiotherapy offer new treatment paths for patients who are not suitable for surgery.6–9 However, the more widely accepted solution is chemotherapy; this is mainly attributed to its broader tumor treatment scope.10 Commonly used chemotherapy drugs include alkylating agents, such as cyclophosphamide, antimetabolite drugs, such as fluorouracil and its derivatives, antitumor antibiotics, such as doxorubicin, camptothecisn, and paclitaxel, etc.11,12 Among these chemotherapeutic drugs, fluorouracil and its derivatives (TF) offer great advantages due to their low cardiotoxicity, relatively weak renal toxicity, and low levels of myelosuppression. In spite of these numerous merits, the clinical application of fluorouracil and its derivatives is restrained due to the high dosage required during tumor treatment, which can easily lead to drug resistance. Therefore, they are commonly used in co-administration or adjuvant administration regimes.13 The design and use of prodrugs is one of the predominant strategies for prolonging drug action time and reducing drug physiological toxicity. TF is the prodrug of 5-fluorouracil (5-FU), which can be metabolized into 5-FU to interfere in the processes of DNA, RNA, and protein synthesis to exert anti-tumor effects.
Photodynamic therapy (PDT) is an emerging anti-tumor therapy modality.14,15 PDT has attracted tremendous attention due to its minimal invasiveness and non-toxicity.16,17 An increasing number of researchers has combined photosensitizers with therapeutic substances in single drug-delivery systems to obtain enhanced anti-tumor efficiency, such as through the conjugation of protoporphyrin IX (PpIX) and peptide,18 pyropheophorbide a and paclitaxel,19 and PpIX and polyethylene glycol.20 Studies have shown that the combination of chemotherapeutic agents and photosensitizers can synergistically facilitate anti-tumor effects, enhance intracellular reactive oxygen species (ROS) generation, and reduce side effects.21–23 Based on these properties of PDT, we look forward to utilizing PDT to improve the efficacy and reduce the side effects of TF.
In this study, we are committed to developing an optimized treatment method for breast cancer patients that can replace surgical treatment; this method is expected to play an important role in improving patient compliance and anti-tumor effects, and in reducing side effects. Thus, a thermosensitive hydrogel encapsulating ROS-responsive TF-PpIX dimers (TTP) was rationally designed and developed. TF and the photosensitizer PpIX were linked via ROS-sensitive thioether bonds. Meanwhile, a TF and PpIX (TSP) dimer compound without ROS sensitive thioether bonds was synthesized as a control to evaluate the release behavior and antitumor efficiency of the ROS-induced prodrug. Excitingly, using a thermosensitive hydrogel as the drug carrier has been proven to be efficient in effectively avoiding various physical and biological barriers in the body, thereby achieving precise and long-term drug release. As we know, low toxicity is a significant criterion for materials that are used in pharmaceutical and biomedical fields. Silk sericin (SS) has been used as a medical material due to its non-toxicity, low immunogenicity, and good biocompatibility.24–26 Chitosan (CS), which has been approved by the FDA, possesses many attractive properties, such as blood compatibility, safety, and microbial degradation.27–29 Both CS and SS are biomimetic materials that have previously been applied in biomaterial fields.30 Also, hydrogels prepared using CS often demonstrate thermosensitive properties. Hence, SS and CS were selected as the materials for the thermosensitive hydrogel, which can be intratumorally injected and transformed into an immovable drug reservoir in the body. Under 630 nm laser irradiation, the thioether bonds can be destroyed by high concentrations of ROS, gradually triggering the release of drugs. Additionally, high concentrations of ROS have shown great success in killing tumor cells. In this treatment system, chemotherapy worked in tandem with photodynamic therapy to improve the anti-tumor effects. The advantages of “on-demand” drug release, low systematic toxicity, and synergistic therapy endow the TTP-loaded thermosensitive hydrogel with great application potential in the field of breast cancer treatment.
2. Materials and methods
2.1. Materials
2.1.1. Reagents.
TF, PpIX, N-(3-dimethylaminopropyl)-N′-ethylcarbodiimide hydrochloride (EDC·HCl, 99.0%), 4-dimethylaminepyridine (DMAP, 99.0%), β-glycerol phosphate disodium salt (GP, 95.0%), and succinic anhydride (SA, 99.0%) were bought from Aladdin Reagent Co., Ltd. Thiodiglycolic anhydride (TA) was purchased from Beijing Hwrkchemical Co., Ltd. Silk sericin (SS) was purchased from Fuxiang Pharmaceutical Technology (Shanghai) Co., Ltd. Chitosan (CS, degree of deacetylation >95.0%, viscosity = 100–200 mPa s) was obtained from Shanghai Macklin Biochemical Co., Ltd. All other chemicals were supplied by Materials Company of Shenyang Pharmaceutical University and were used as received.
2.1.2. Cells and animals.
Mouse breast-cancer 4T1 cells and human breast-cancer MCF-7 cells were obtained from Wuya College of Innovation, Shenyang Pharmaceutical University, Shenyang, China, and incubated in RPMI 1640 medium with 10% FBS and 1% penicillin–streptomycin in a CO2 incubator at 37 °C.
BALB/c mice and Sprague-Dawley (SD) rats were bought from the Laboratory Animal Center of Shenyang Pharmaceutical University, Shenyang, China. All animal procedures were performed in accordance with the Guidelines for the Care and Use of Laboratory Animals of “Shenyang Pharmaceutical University” and approved by the Animal Ethics Committee of “Shenyang Pharmaceutical University”, Shenyang, China.
2.2. Synthesis of TTP/TSP
The reaction between TF and formaldehyde was carried out based on a previously reported protocol.31,32 Typically, TF (20.0 g, 0.1 mol) was mixed with 10 mL of 40% formaldehyde aqueous solution. The mixture was placed in an oil bath (50 °C) and stirred for 6 h. The water and formaldehyde were removed under reduced pressure to obtain TF-OH. TF-OH was then used to carry out the conjugation of TF and TA or SA (TF-TA/TF-SA). The synthesis process of TF-TA/TF-SA was as follows. Briefly, TF-OH (2.16 g, 0.01 mol), TA (1.32 g, 0.01 mol)/SA (1.0 g, 0.01 mol), and triethylamine (TEA, 1.39 ml, 0.01 mol) were dissolved in 10 mL of dichloromethane (DCM). The solution was kept under darkness and the reaction proceeded under the protection of nitrogen at room temperature for 4 h. The reaction was monitored via thin-layer chromatography (TLC). The product, TF-TA/TF-SA, was obtained after purifying using silica gel.
For the conjugation of TF and PpIX, ethylene glycol (EG) was introduced to TF-TA/TF-SA as a crosslinker. TF-TA (0.724 g, 2 mmol)/TF-SA (0.66 g, 2 mmol), EDC·HCl (0.382 g, 2 mmol), and a catalytic amount of DMAP were dissolved in 10 mL of DCM. The solution was stirred in an ice bath for 30 min to activate the carboxyl groups. EG (0.248 g, 4 mmol) was added to the solution and the mixture was stirred under nitrogen protection at room temperature for 12 h. TLC was used to monitor the progress of the reaction. The product, TF-TA-EG/TF-SA-EG, was achieved following silica gel purification.
Then the final TF and PpIX conjugate products with and without thioether bonds (TTP/TSP) were obtained via an esterification reaction. In detail, PpIX (0.563 g, 1 mmol), EDC·HCl (0.192 g, 1 mmol), and a catalytic amount of DMAP were dissolved in 20 mL of THF. The reaction system was placed in an ice bath and stirred under darkness for 30 min to activate the carboxyl groups of PpIX. Then, TF-TA-EG (0.812 g, 2 mmol)/TF-SA-EG (0.748 g, 2 mmol) was added to the above solution, and the reaction was allowed to proceed under nitrogen protection under darkness for another 12 h. TLC was applied to monitor the reaction. The final products were purified via preparative liquid chromatography, with a mobile phase of acetonitrile
:
water = 95
:
5 (v/v) for TTP and acetonitrile
:
water = 93
:
7 (v/v) for TSP. For all reactions, mass spectrometry (MS, Water ACQUITY QDA)/Fourier-transform ion cyclotron resonance mass spectrometry (FT-ICR MS, BRUKER Soarix7.0T), Fourier-transform infrared (FT-IR, BRUKER TENSOR 27) spectroscopy, and nuclear magnetic resonance (1H NMR, BRUKER AVANCE III 600 MHz) spectroscopy analyses were utilized to confirm the structures of the intermediate and final products.
2.3. Characterization of the in vitro properties of TTP/TSP
TTP, TSP, and their intermediate products were dissolved in methanol to form solutions with concentrations of about 0.02 mg mL−1. The ultraviolet (UV) spectra were obtained using UV-1800 apparatus (Shimadzu, Japan). PpIX, TTP and TSP were dissolved in DMSO at a certain PpIX equivalent concentration, and fluorescence spectra were detected using a Varioskan Flash molecular device (Thermo Scientific ). Differential scanning calorimetry (DSC) data were recorded using a METTLER TOLEDO DSC1 instrument (METTLER TOLEDO).
For ROS generation assays, dichlorofluorescin diacetate (DCFH-DA) was used as the fluorescence probe, and it hydrolyzed into dichlorofluorescin (DCFH), as previously reported.33 PpIX, TTP, and TSP were dissolved in 0.1 mL of DMSO and diluted to 0.5 μM (PpIX equivalent concentration) with deionized water. 200 μL samples were added into a 96-well plate and 10 μL of DCFH was added to evaluate the ROS generation by each sample. The fluorescence intensity of DCF converted by ROS from DCFH was detected using a molecular device at 488 nm excitation and 530 nm emission wavelengths after 630 nm laser irradiation (50 mW cm−2) for a predetermined time interval.
2.4. The preparation and characterization of TTP/TSP thermo-sensitive hydrogels
CS (1.75 g) was added into 0.1 M acetic acid aqueous solution (50 mL) slowly under continuous stirring, and stirring was continued for another 15 min until the chitosan dissolved entirely. SS (1.75 g) was dissolved in 50 mL of deionized water carefully. A certain ratio (v/v) of CS solution and SS solution was mixed, followed by the addition of GP aqueous solution (50%, w/v) at a chitosan solution to GP solution volume ratio of 1
:
1 under continuous stirring. For the preparation of the prodrug-loaded hydrogels, TTP/TSP was mixed with SS to form a homogeneous powder mixture and dissolved in water with 0.5 wt% poloxamer F68. All procedures were performed at room temperature.
The pH values of solutions were determined using a Mettler S220-K-CN pH meter before gelation. The gelation time was measured using the test-tube inversion method. In short, the solution was incubated at 37 °C, and the gelation time refers to the time required for the solution to no longer flow after the test tube was inverted. The drug loading capacity (DLC) was determined via an acid-damage method. Briefly, 50 mg of TTP hydrogel or TSP hydrogel was added to 10 mL of HCl (1 M) under magnetic stirring. NaOH solution (10 mL, 1 M) was added to adjust the pH to neutral after the acidic hydrogel solution was stirred under darkness for 8 h. 0.5 mL of the mixed solution was removed and 20 μL of the supernatant was analyzed using a HPLC instrument equipped with a UV-vis detector (HITACHI Chromaster 5410) at 271 nm after the solution had been centrifuged for 10 min (10
000 rpm). The concentration of TF-OH was determined and the DLC of TF was calculated according to the following formula:
where
WTF-OH is the content of TF-OH in the measured hydrogel;
WTF-OH/230.07 × 200.17 is the amount of TF calculated based on TF-OH; and
Whydrogel is the amount of drug-loaded hydrogel.
The ROS generation abilities of PpIX hydrogel, TTP hydrogel, and TSP hydrogel were measured using the DCFH method, as described before. The hydrogels were lyophilized, and the morphologies of the lyophilized hydrogel powder were characterized using a Hitachi S-3400 N scanning electron microscope (SEM). FT-IR spectra were recorded using a BRUKER TENSOR 27 FT-IR spectrometer.
Lysozyme and elastase K were used to study the in vitro degradation of the hydrogels. In short, 1.5 mL of blank sol, TTP sol, and TSP sol were incubated respectively at 37 °C until gelation was complete. 2 mL of normal saline solution containing lysozyme (0.4 mg mL−1) and elastase K (0.2 mg mL−1) was added to the top of the hydrogels. The culture medium was changed and the remaining hydrogel was accurately weighed once a day.
2.5. Rheological measurements
The drug-loaded hydrogels and blank hydrogels were characterized via the temperature mode of a rotational rheometer using AR 2000EX apparatus (TA Instruments) equipped with a parallel-plate sample holder (40 mm in diameter). The elastic modulus (G′) and viscous modulus (G′′) data were recorded as the temperature ranged from 20 to 50 °C; a frequency of 1 Hz, strain of 1%, and heating rate of 2 °C min−1 were selected.
2.6. Photodynamic triggered TF-OH release in vitro
According to previous studies, TF-OH can be transformed into TF and formaldehyde via enzymolysis, which generates a synergistic anti-tumor effect in vivo.31,32 Therefore, this article investigated the in vitro release behavior of TF-OH from the prodrug-loaded hydrogel using a dialysis method. In order to determine the effects of ROS on the release behaviors of the TTP hydrogel and TSP hydrogel, normal saline solution with 10 mM H2O2 was used as the release medium. The prodrug-loaded hydrogel was added into dialysis tubes containing 5 mL of release medium, followed by immersion in 30 mL of release medium. A VCL-630nmLNE1 (Beijing Honglan Light Technology Co., Ltd) 630 nm laser device was used to irradiate the hydrogel (630 nm, 150 mW cm−2) for 5 min at predetermined time intervals. The continuous mode of the laser device was used, and laser irradiation was performed once a day until the end of the test period. The released TF-OH in the release medium was determined using a HPLC instrument (HITACHI Chromaster 5110) equipped with a UV-vis detector (HITACHI Chromaster 5410) at 271 nm, with a mobile phase of acetonitrile
:
water (30
:
70, v
:
v). In order to study drug release triggered by ROS and synergetic triggered drug release via a combination of ROS and laser irradiation, 10 mM H2O2 alone and 10 mM H2O2 with laser irradiation were used.
2.7.
In vitro cytotoxicity
4T1 and MCF-7 cells were seeded in 96-well plates at densities of 5 × 103 cells per well and incubated overnight. Different concentrations of blank hydrogel, the market preparation of the tegafur injection Fangke®, TTP hydrogel, and TSP hydrogel diluted with the aforementioned culture medium were added and co-incubated with the cells for 24, 48, and 72 h, respectively. Then, 10 μL of CCK-8 reagent was added to each well, and incubation proceeded for a further 4 h. A Varioskan Flash microplate reader (Thermo Scientific) was used to detect the absorbance of each well at 450 nm. The cell viabilities were calculated according to the following formula:
where Ae is the absorbance of the experimental group, Ab is the absorbance of the blank group, and Ac is the absorbance of the control group.
The cytotoxicity under the synergistic effect of PDT and chemotherapy was also studied. After cells were treated with the TTP hydrogel or TSP hydrogel for 4 h, cells were irradiated with a laser at 630 nm (60 mW cm−2) for 100 s. Cells were incubated for a further 44 h, and 10 μL of CCK-8 reagent was added to each well, followed by another 4 h of incubation. The absorbance was detected using a microplate reader, and the cell viabilities were calculated according to the above formula. The combination index (CI) of chemotherapy and photodynamic therapy was calculated, according to a formula previously reported, as follows:19
where IC
50,TTP+L is the IC
50 value of the TTP hydrogel group under laser irradiation, IC
50,TTP is the IC
50 value of the TTP hydrogel group, and IC
50,TSP is the IC
50 value of the TSP hydrogel group.
2.8. Intracellular TF release and cellular uptake assays
4T1 cells were seeded in a 24-well plate at a density of 2 × 105 cells per well and incubated overnight. The medium was replaced with Fangke® and TTP/TSP hydrogel solution (50, 100, 150 μg mL−1, based on TF equivalent), diluted with RPMI 1640 medium, and incubated at 37 °C. After 1, 2, and 4 h of incubation, the media containing Fangke® and TTP/TSP hydrogel were removed, and cells were washed with PBS 3 times to remove residual drug traces. 0.3 mL of Triton X-100 (1%) was added to each well, and culturing was continued for another 30 min under shaking to lyse the cells. 200 μL of cell lysis buffer was taken, and proteins were precipitated via mixing the cell lysis buffer with 200 μL of methanol using a Vortex Genie 2T vortex mixer (Scientific Industries). After centrifugation for 10 min at 12
000 rpm, 20 μL of supernatant was taken for quantitative analysis via HPLC, and another 100 μL of supernatant was examined using a microplate reader. The protein content was corrected using a bicinchoninic acid (BCA) protein quantitation kit assay. For the ROS-mediated TF release assay, cells were irradiated using a laser at 630 nm for 100 s at 60 mW cm−2 after 2 h of co-incubation with the hydrogels.
The observation of cellular uptake was conducted via confocal laser scanning microscopy (CLSM, Nikon C2 plus). After 1, 2, and 4 h of co-incubation with PpIX, TTP, and TSP hydrogels, 4T1 cells in a 6-well plate at a density of 1 × 105 cells per well were fixed with 4% paraformaldehyde. The cell nuclei were stained with DAPI, and cells were observed and photographed via CLSM.
2.9. Cellular uptake mechanism of the hydrogels
The mechanism of the cellular uptake of the hydrogels was studied via incubating 4T1 cells in a 24-well plate at a density of 1 × 105 cells per well with cellular uptake inhibitors (chlorpromazine, sodium azide, 5-(N-ethyl-N-isopropyl)amiloride (EIPA), nocodazole, indomethacin, quercetin, and monensin sodium) for 2 h. Then, the medium containing the inhibitors was replaced with medium containing TTP hydrogel, TSP hydrogel, and PpIX hydrogel, respectively, and incubation was continued for another 2 h. After the cells were lysed as described above, TF in the cell lysis buffer was detected via the HPLC method and the TTP and TSP content levels were measured using a microplate reader.
2.10. Intracellular ROS detection
A DCFH-DA kit was used to detect the ROS generation of different preparations in 4T1 cells observed via CLSM. 4T1 cells were seeded in a 6-well plate at a density of 1 × 105 cells per well and incubated overnight. After 12 h, the medium was discarded. PpIX hydrogel, TTP hydrogel, and TSP hydrogel at a TF equivalent concentration of 20 μg mL−1 (diluted in culture medium) were added, respectively, and culturing was continued for another 4 h. Cells were washed twice with PBS buffer after the hydrogel-containing medium was removed. 1 mL of DCFH-DA solution (10 μmol L−1) was added to each well, and the cells were cultured in a CO2 incubator at 37 °C under darkness for a further 30 min. Then, residual DCFH-DA was clearly removed. 1 mL of medium was added to each well, and for the laser irradiation groups, the cells were irradiated with a 630 nm laser (60 mW cm−2) for 5 min. The quantitative detection of ROS was conducted via a microplate reader at fluorescence wavelengths of λEx = 488 nm and λEm = 525 nm. The qualitative analysis of ROS generation was undertaken via CLSM after the cells were fixed with 4% formaldehyde.
2.11. Plasma pharmacokinetics studies
In order to study the pharmacokinetics of TTP/TSP prodrugs in vivo, a known proportion of poloxamer F68 was used as a solubilizer to dissolve TTP/TSP in normal saline at a TF equivalent concentration of 5 mg mL−1. SD rats were selected to investigate the pharmacokinetics properties of TTP and TSP. The market preparation Fangke® was used as a control. SD rats were intravenously treated with the preparations (50 mg kg−1 TF equivalent). 0.5 mL of blood was collected from orbital veins and centrifugated (12
000 rpm, 10 min ). The supernatant was collected, and protein was precipitated using methanol. After centrifugation, 20 μL of sample was analyzed via the HPLC method using a HITACHI Chromaster 5110 instrument equipped with a HITACHI Chromaster 5410 UV-vis detector. The amounts of TF, TTP, and TSP in plasma were examined.
2.12. Biocompatibility, biodegradability, and in vivo biodistribution
A tumor-bearing mouse model was established to investigate the biocompatibility, biodegradability, and biodistribution in vivo of the different hydrogels. 4T1 cells were injected subcutaneously into the left axillae of BALB/c mice at 2 × 106 cells per mouse. For biocompatibility assays, normal saline and blank hydrogel were injected intratumorally when the tumors grew to about 200 mm3. Tumors were collected and stained with hematoxylin–eosin (H&E). An inverted microscope was utilized to investigate the biocompatibility of the hydrogel. For biodegradability, tumors with sizes of 200 mm3 were treated with blank hydrogel, TTP hydrogel, and TSP hydrogel. Mice were sacrificed at a predetermined time, and the hydrogel in the tumor was carefully collected and weighed. As for biodistribution sections, when the tumors reached around 400 mm3, DiR-loaded PpIX hydrogel, TTP hydrogel, and TSP hydrogel were injected intratumorally at a PpIX equivalent concentration of 10 mg kg−1. An IVIS Lumina III (PerkinElmer) small-animal living imaging system was used to monitor the distribution of hydrogels in mice after 0, 1, 3, and 5 days. Mice were sacrificed on the fifth day after administration, and tumors, hearts, livers, spleens, lungs, and kidneys were taken for in vivo imaging. In order to quantitatively detect TF and its prodrugs in the plasma, major organs, and tumors of tumor-bearing mice, mice were intratumorally injected with TTP hydrogel and TSP hydrogel. After 3 days of injection, mice were sacrificed, and plasma, major organs, and tumors were collected. The TF, TTP, and TSP content levels were determined using a HPLC instrument.
2.13. Anti-tumor efficiency in vivo
BALB/c mice were treated with 2 × 106 4T1 cells subcutaneously to establish a tumor-bearing mouse model, as described above. When the tumors reached about 100 mm3, the tumor-bearing mice were divided into 6 groups randomly (6 mice per group): normal saline group, Fangke® group, TTP hydrogel group, TTP hydrogel with laser group, TSP hydrogel group, and TSP hydrogel with laser group. For the Fangke® group, the market preparation tegafur injection Fangke® was injected venously at a dosage of 20 mg kg−1, once every 2 days. For the TTP hydrogel and TSP hydrogel without laser groups, TTP hydrogel and TSP hydrogel were injected intratumorally at a TF equivalent concentration of 60 mg kg−1, once every 7 days. For the TTP hydrogel and TSP hydrogel with laser groups, the dosage and interval of administration were the same as those for the TTP hydrogel and TSP hydrogel without laser groups, except that 630 nm laser irradiation was performed at 250 mW cm−2 after 1 day of intratumoral administration, thrice a week, for 10 min each time. The tumor volumes were measured every 2 days, and they were calculated with the following formula: V = (tumor length) × (tumor width)2/2, and the mice were weighed once a day. The tumor inhibition rate (TIR) was calculated according to the formula: TIR (%) = (1-tumor weight of treatment group/tumor weight of normal saline group) × 100%. At the end of treatment, mice were sacrificed, and tumors and major organs were collected for histological analysis. Formaldehyde fixation and H&E staining were used for analysis.
2.14. Statistical analysis
All data are presented as mean ± standard deviation (n = 3 for in vitro experiments and n = 6 for in vivo experiments). Differences between groups were evaluated based on p values, which were calculated from Student's t-test, and p < 0.5 was considered a significant difference; p < 0.01 was considered an extremely significant difference.
3. Results and discussion
3.1. The synthesis and structure confirmation of TTP/TSP
The processes for the synthesis of TTP and TSP are detailed in Fig. S1.† Firstly, TF and formaldehyde were reacted via an Eschweiler–Clarke reaction to generate a TF derivative containing a hydroxyl group. Then, the hydroxyl group was conjugated with TA/SA via esterification. The free carboxyl group of TF-TA/TF-SA reacted with the EG linkage through further esterification to form TF-TA-EG/TF-SA-EG. Finally, PpIX was coupled with the remaining hydroxyl groups of the EG linkage to form the final product: TTP/TSP. Fig. 1A shows the 1H NMR spectra of TTP and TSP. The 1H NMR spectra confirmed the chemical structures of the intermediates and final products (Fig. S2 and S3†). The 1H NMR assignments of the synthesized compounds are shown in the supplementary material. The MS and FT-ICR MS data in Fig. S4 and S5† further support the structures of these products. The FT-IR spectra in Fig. 1B and S6† show signals from ester bonds in TTP, TF-TA, TF-TA-EG, TSP, TF-SA, and TF-SA-EG at 1734, 1730, 1732, 1735, 1732, and 1734 cm−1, respectively; this is consistent with their chemical structures shown in Fig. S1.†
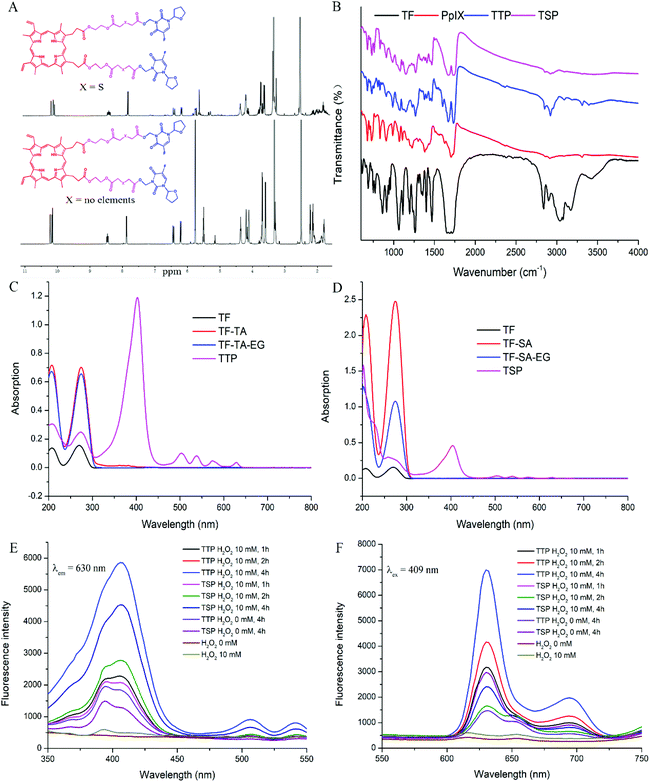 |
| Fig. 1 The structure confirmation and in vitro properties of TTP and TSP. (A) 1H NMR spectra of TTP and TSP. (B) FT-IR spectra of TF, PpIX, TTP, and TSP. (C) UV-vis spectra of TF, TF-TA, TF-TA-EG, and the TTP monomer. (D) UV-vis spectra of TF, TF-SA, TF-SA-EG, and the TSP monomer. (E) Fluorescence excitation spectra of TTP and TSP at a fixed emission wavelength of 630 nm following incubation in H2O2 at 0 or 10 mM. (F) Fluorescence emission spectra of TTP and TSP at a fixed excitation wavelength of 409 nm following incubation in H2O2 at 0 or 10 mM. | |
3.2. The in vitro properties of TTP/TSP
The UV-vis spectra of TTP, TSP, and derivatives of TF are shown in Fig. 1C and D. TF and its derivatives showed pyrimidine absorption at 207 nm and 274 nm, which illustrated that the simple modification of TF has no significant effects on the UV absorption of the pyrimidine ring. After conjugation with PpIX, the peaks of the pyrimidine portion of TTP shifted to 208 nm and 270 nm, the peaks of the pyrimidine portion in TSP shifted to 202 nm and 259 nm, and sharp Soret bands at 402 nm (TTP) and 404 nm (TSP) from PpIX appeared. The shifted absorption indicated the existence of p–π stacking between the pyrimidine ring and porphyrin ring.
The fluorescence spectra of TTP, TSP, and PpIX showed strong fluorescence signals at 630 nm (Fig. S2A†), which demonstrated that the conjugation of TF derivatives and PpIX had no effect on the fluorescence wavelength of PpIX. In consideration of the increasing importance of fluorescence in the diagnosis and treatment of tumors, the ability of TTP and TSP to maintain their fluorescence properties is essential for tumor therapy. The ROS generated by H2O2 can promote the dissociation of the thioether bonds of TTP and, as a result, the fluorescence intensity of TTP was much higher than that of TSP when they were incubated with H2O2 (Fig. 1E and F). As the concentration of H2O2 increased, the fluorescence intensity of TTP increased (Fig. S7C and D†), indicating that the cleavage of thioether bonds was ROS-concentration-dependent. According to DSC measurements (Fig. 2A), the melting point of PpIX was higher than 230 °C and the melting point of TF was 175 °C. A physical mixture of the two had an endothermic peak at 167 °C. In the range of 25–230 °C, TTP and TSP had endothermic peaks at 131 and 112 °C, respectively, as shown in Fig. 2A.
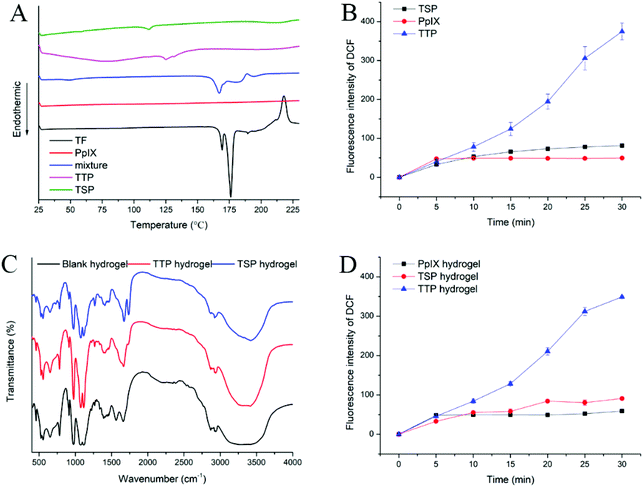 |
| Fig. 2 (A) The DSC curves of TF, PpIX, a mixture of TF and PpIX at a weight ratio of 0.42 : 0.58, TTP prodrug, and TSP prodrug. (B) ROS generation from PpIX, TTP, and TSP under 630 nm laser irradiation with time (n = 3). (C) FT-IR spectra of blank hydrogel, TTP-loaded hydrogel, and TSP-loaded hydrogel. (D) ROS generation from PpIX hydrogel, TTP hydrogel and TSP hydrogel under 630 nm laser irradiation with time (n = 3). | |
DCFH-DA can be converted to DCFH via hydrolysis, and DCFH can be oxidized to DCF with fluorescent properties by ROS. In this study, NaOH was used to hydrolyze DCFH-DA, and then DCFH was used to study the generation of ROS. After laser irradiation at 630 nm, the fluorescence intensity of DCF in the TTP group was significantly higher than those in the TSP and PpIX groups (Fig. 2B). This may be due to a reduction in the ability of PpIX to generate ROS, caused by aggregation. PpIX was released from TTP under laser irradiation, which reduced the aggregation of PpIX, thereby generating more ROS. However, TSP cannot release PpIX through ROS-mediated cleavage, which affected the generation of ROS.
3.3. Thermosensitive hydrogel formulation and its characterization
Firstly, the blank thermosensitive hydrogel was prepared, and the formulation was optimized based on the gelation time. As depicted in Table 1 and Fig. S8A–C,† the gelation time decreased as the amount of GP was increased. When CS
:
GP reached 1
:
1 (v/v), the gelation time did not change significantly when the amount of GP was continually increased. The amount of SS had little effect on the gelation time, but when CS
:
SS exceeded 1
:
1 (v/v), the hydrogel solution was too viscous to be injected. Thus, the CS/GP/SS ratio was fixed at 1
:
1
:
1 (v/v/v).
Table 1 The preparation and optimization of the CS/GP/SS thermosensitive hydrogel
CS (3.5%, w/v)/GP (50%, w/v)/SS (3.5%, w/v) (v/v/v) |
pH |
Gelation time at 37 °C (min) |
1 : 0.2 : 1 |
6.96 ± 0.02 |
7.81 ± 3.14 |
1 : 0.5 : 1 |
7.01 ± 0.01 |
6.36 ± 2.86 |
1 : 0.8 : 1 |
7.27 ± 0.01 |
5.47 ± 1.27 |
1 : 1 : 1 |
7.48 ± 0.02 |
5.23 ± 0.51 |
1 : 1.5 : 1 |
7.62 ± 0.01 |
4.41 ± 0.38 |
1 : 2 : 1 |
7.84 ± 0.03 |
3.95 ± 0.16 |
1 : 1 : 0.2 |
7.47 ± 0.02 |
9.34 ± 0.42 |
1 : 1 : 0.5 |
7.42 ± 0.01 |
8.89 ± 0.47 |
1 : 1 : 0.8 |
7.38 ± 0.02 |
5.65 ± 0.39 |
1 : 1 : 1.5 |
7.30 ± 0.03 |
3.43 ± 0.51 |
1 : 1 : 2 |
7.13 ± 0.05 |
3.21 ± 0.43 |
1.5 : 1 : 1 |
7.42 ± 0.01 |
6.70 ± 0.29 |
2 : 1 : 1 |
7.41 ± 0.02 |
9.64 ± 0.76 |
3 : 1 : 1 |
7.42 ± 0.02 |
12.93 ± 0.82 |
3 : 1 : 2 |
7.46 ± 0.01 |
7.51 ± 0.43 |
3 : 1 : 3 |
7.45 ± 0.04 |
3.22 ± 0.21 |
As TTP/TSP has poor water solubility, the proper addition of poloxamer F68 can increase the water solubility of TTP/TSP to make it disperse better in hydrogel solution. As illustrated in Table 2 and Fig. S8D,† when the amount of F68 was fixed at 0.5 wt%, the gelation time gradually increased with an increase in drug loading. Therefore, an appropriate drug loading capacity, with a suitable gelation time, of SS/TTP or TSP of 3
:
1 was selected. The amount of poloxamer F68 had almost no influence on the gelation time, and 0.5 wt% poloxamer F68 can better increase the solubility of TTP/TSP; therefore 0.5 wt% poloxamer F68 was chosen. Ultimately, the ratio of CS/GP/SS was determined to be 1
:
1
:
1 (v/v/v), with TTP/SS or TSP/SS of 3
:
1 (w/v), and 0.5 wt% poloxamer F68. The DLCs of the optimal TTP and TSP hydrogels, determined via HPLC, were 7.66% and 7.73%, respectively.
Table 2 The preparation and optimization of the drug-loaded CS/GP/SS thermosensitive hydrogel
TTP or TSP/SSa (w/v) |
Poloxamer F68 (wt%) |
pH |
Gelation time at 37 °C (min) |
The ratio of CS/GP/SS was fixed at 1 : 1 : 1 (v/v/v); the concentrations of CS, GP, and SS were 3.5% (w/v), 3.5% (w/v), and 50% (w/v), respectively; SS refers to SS solution.
|
1 : 5 |
0.5 |
7.46 ± 0.02 |
5.31 ± 0.13 |
1 : 3 |
0.5 |
7.47 ± 0.01 |
6.24 ± 0.25 |
1 : 1 |
0.5 |
7.46 ± 0.02 |
8.17 ± 0.47 |
1 : 3 |
0.2 |
7.48 ± 0.00 |
6.12 ± 0.28 |
1 : 3 |
1 |
7.48 ± 0.01 |
6.01 ± 0.42 |
TTP or TSP was physically embedded in the hydrogel and might interact with CS or SS through hydrogen bonds and van der Waals forces. FT-IR spectra of blank hydrogel, TTP hydrogel, and TSP hydrogel lyophilized powder are shown in Fig. 2C. The –OH stretching vibration peaks of blank hydrogel, TTP hydrogel, and TSP hydrogel samples appeared at 3290, 3315, and 3419 cm−1, respectively. The stretching vibration peaks of the ether bonds of the blank hydrogel were at 1072 and 1115 cm−1, while the stretching vibration peaks of the ether bonds of the TTP hydrogel and TSP hydrogel lyophilized powder were shifted to 1074 and 1117 cm−1, respectively. The stretching vibration peaks of the amide I and amide II bands of the blank hydrogel were at 1562 and 1661 cm−1, respectively. However, the stretching vibration peaks of the amide I bands of the TTP and TSP hydrogel lyophilized powder disappeared, and the stretching vibration peaks of the amide II bands were shifted to 1665/1672 cm−1. In addition, the stretching vibration peaks of ester bonds of TTP and TSP appeared at 1728 and 1736 cm−1, respectively. The characteristic stretching vibration peaks of CS and SS appeared in the FTIR spectra of the hydrogels, but compared with the blank hydrogel, the characteristic stretching vibration peaks of the TTP and TSP hydrogel lyophilized powder showed certain shifts, indicating that there was a certain level of interaction between CS, SS, and TTP or TSP. The existence of ester bond stretching vibration peaks of TTP and TSP proved that they maintained their structural integrity in hydrogel form. To investigate the ROS generation abilities after hydrogel formation, the DCFH method was applied. As described in Fig. 2D, after PpIX, TTP, and TSP were prepared as hydrogels, the ROS generation trends remained the same as before hydrogel formation, and the ROS generation abilities did not significantly change.
As shown in Fig. 3A, the hydrogels retained flowability at 25 °C. After incubation at 37 °C, the molecules of CS and SS in the hydrogels were crosslinked and the hydrogels were transformed from flexible sols into non-flowable semi-solid gels. SEM images of hydrogel lyophilized powder are shown in Fig. 3B–D. The addition of TTP and TSP resulted in denser heterogeneous porous network hydrogel structures, which may be attributed to the interaction of TTP and TSP with the macromolecules CS and SS in the hydrogel.
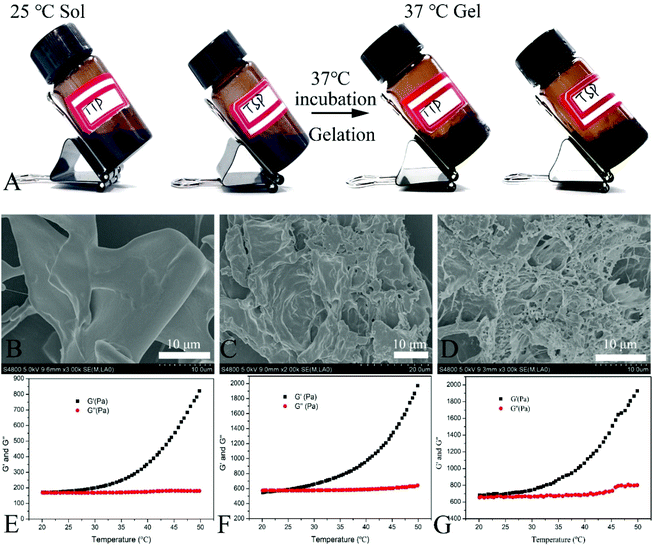 |
| Fig. 3 The characterization of the physical properties of blank hydrogel and TTP/TSP hydrogel samples. Hydrogels before gelation and after gelation (A). SEM images of blank hydrogel (B), TTP hydrogel (C), and TSP hydrogel (D). The rheological curves of blank hydrogel (E), TTP hydrogel (F), and TSP hydrogel (G). | |
Rheological analysis explained the process of the hydrogel solution transition from a sol state to a gel state (Fig. 3E–G). We tested the rheological behavior of hydrogel solutions from 20 °C to 50 °C. The elastic modulus (G′) and viscous modulus (G′′) increased slowly between 20–30 °C, however, when the temperature reached 30 °C, G′ changed drastically. As the temperature continued to increase, G′ increased sharply, which indicated that the hydrogel underwent a phase transition from a sol to a gel from 30–50 °C. The addition of TTP, TSP, and F68 increased both G′ and G′′, which may be ascribed to the existence of laminar pyrimidine and porphyrin structures in TTP and TSP. These components can result in physical conjugation, hydrogen bonds, and van der Waals forces with CS and SS, thus increasing the viscosity and elasticity of hydrogels. Furthermore, the addition of poloxamer F68 increased the viscoelasticity of the hydrogels.
Lysozyme is a component of the immune defense system of a healthy body, and it has the ability to dissociate bacterial cytoderm. In the human body, it exists in neutrophils, monocytes and macrophages, and mucosal secretions.34 Chitosan can be hydrolyzed by lysozyme and then be metabolized.35 Elastase is widely distributed in biological serum, neutrophils, macrophages, and other cells, and it is a broad-spectrum proteolytic enzyme that can hydrolyze SS and poloxamers.36 Lysozyme and elastase were chosen to study the in vitro degradation of blank hydrogel, TTP hydrogel, and TSP hydrogel. As depicted in Fig. S9,† the hydrogels in each group degraded by more than 60% in 7 days and by more than 80% in 10 days, which is a suitable degradation time for a hydrogel that will be applied in vivo. Accordingly, the hydrogels will not remain at the injection site for an excessive amount of time, thereby avoiding adverse effects due to treatment; they also will not cause drug leakage in advance due to excessive degradation, thus achieving the effects of sustained release.
3.4. TF-OH release under laser irradiation
Hydrophobic sulfide bonds are easily oxidized by ROS and converted into hydrophilic sulfone or sulfoxide groups; this in turn affects the ester bonds and makes them vulnerable to attack.37,38 TF-OH in the prepared TTP and TSP is released through ester bond breakage and then converted into TF and formaldehyde via enzymolysis in the biological system to provide synergetic chemotherapy efficiency.32 Hence, TF-OH was analyzed in order to evaluate the drug release behavior of drug-loaded hydrogels. In order to study the effects of ROS on the release of TF-OH, 10 mM H2O2 was added to the release medium. The results in Fig. 4 show that compared with TSP, the release of TF-OH from TTP hydrogel triggered by ROS was significantly increased, and under 630 nm laser irradiation, TF-OH leakage from TTP hydrogel was further accelerated. This indicated that the thioether bonds in TTP were easily oxidized by ROS to accelerate drug release, and under laser irradiation, the ROS generated by PpIX further accelerated the oxidation of the thioether bonds, so that TTP drug release was accelerated. Meanwhile, the long duration of drug release (TF-OH was almost completely released from TTP hydrogel within 10 days under laser irradiation) was attributed to barrier obstructions due to the network structure of the CS/SS/GP hydrogel. Long-term and laser-controlled drug release can meet the requirements for on-demand drug delivery. Laser irradiation can allow the drug to exert its efficiency at a specific time during treatment. This property can greatly improve patient compliance.
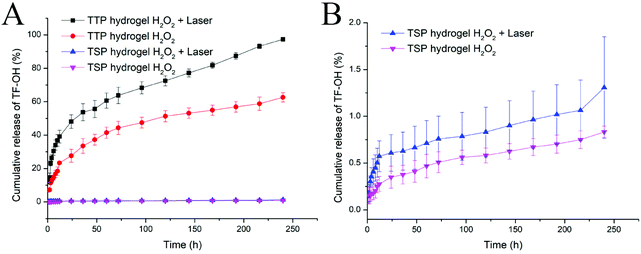 |
| Fig. 4 ROS- and laser-irradiation-triggered TF-OH release from TTP and TSP hydrogels (n = 3). (A) TF-OH release from TTP and TSP with 10 mM H2O2 and with/without laser irradiation. (B) TF-OH release from TSP with 10 mM H2O2 and with/without laser irradiation. | |
3.5. Intracellular ROS generation and the laser-irradiation-promoted inhibition of cellular proliferation
The generation of ROS in 4T1 cells measured via the DCFH-DA method is shown in Fig. 5A and Fig. S10.† PpIX hydrogel, TTP hydrogel, and TSP hydrogel hardly generated ROS when there was no laser irradiation. After 660 nm laser irradiation, strong green fluorescence was observed from the cells treated with hydrogels. This indicated that laser irradiation could allow PpIX, TTP, and TSP to generate vast amounts of ROS, subsequently converting DCFH into DCF. The fluorescence intensities of cells co-incubated with PpIX hydrogel and TTP hydrogel were similar, while the fluorescence intensity of cells co-incubated with TSP hydrogel was weaker; this indicated that TTP, with its thioether bonds, had a stronger ability to generate ROS than TSP. This was probably because TTP was more likely to undergo bond breakage and release PpIX after laser irradiation, afterwards producing ROS; therefore, the amounts of ROS generated by PpIX and TTP in the unaggregated state were similar.
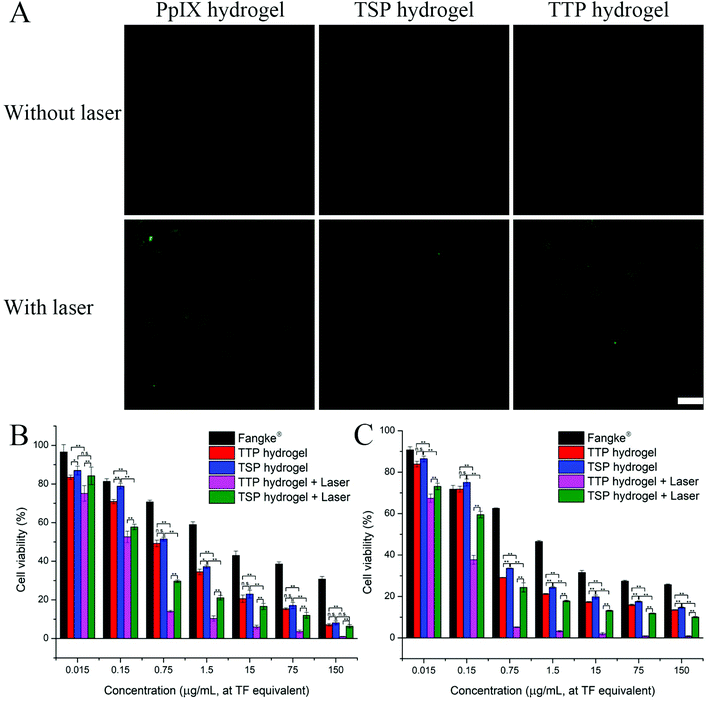 |
| Fig. 5 The in vitro cytotoxicity of Fangke® and the hydrogels, and the ROS generation abilities of the hydrogels (n = 3). ROS generation abilities of PpIX hydrogel, TTP hydrogel, and TSP hydrogel (A). A study of the cytotoxic effects of Fangke®, TTP hydrogel, and TSP hydrogel on 4T1 cells (B) and MCF-7 cells (C). Scale bar = 10 μm; *: p < 0.05, significant difference; **: p < 0.01, extremely significant difference; n.s.: p > 0.05, no significance. | |
ROS can induce cell apoptosis,39 and TTP is ROS-sensitive and can accelerate drug release under laser irradiation. Based on the fact that PpIX, TTP, and TSP can generate ROS both intracellularly and extracellularly, we investigated the cytotoxicities of TTP hydrogel and TSP hydrogel under laser irradiation and without laser irradiation. Fangke® was chosen as a control to study the anti-tumor abilities in vitro. The IC50 values of each group of preparations in relation to mouse breast-cancer 4T1 cells and human breast-cancer MCF-7 cells are listed in Table 3. The influence of the blank hydrogel on cytotoxicity was excluded, as shown in Fig. S11.† As seen in Fig. 5B and C, TTP hydrogel exhibited considerable cytotoxicity toward 4T1 cells and MCF-7 cells under laser irradiation. A combination of PDT and chemotherapy maximized the cytotoxicity of TTP hydrogel under laser irradiation conditions. The cytotoxicity of TTP hydrogel without laser irradiation was relatively weaker compared with under laser irradiation, and it was similar to that of TSP hydrogel without laser irradiation. Although TSP hydrogel can also produce a certain amount of ROS to promote cell apoptosis under laser irradiation, its capabilities were far inferior to TTP hydrogel under irradiation. Laser irradiation further accelerated drug release from TTP, in turn taking advantage of the combination of PDT and chemotherapy to a favorable extent. The cytotoxicity of Fangke® was greatly limited compared with TTP hydrogel and TSP hydrogel (Fig. 5 and Fig. S12†). In cells, TTP and TSP can decompose into TF, formaldehyde, and PpIX, which have a synergistic chemotherapeutic effect.32 The CI values of 4T1 cells and MCF-7 cells were calculated to be 0.39 and 0.20, respectively, and both were <1, indicating that TTP hydrogel and TSP hydrogel exerted synergistic anti-tumor effects from PDT and chemotherapy. In general, TTP hydrogel combined with laser irradiation was advantageous because: (a) photodynamic therapy facilitated the release of TF; (b) the synergistic chemotherapy of intracellular metabolites due to TF-OH promoted the efficiency of chemotherapy; and (c) chemotherapy and PDT synergistically promoted cell apoptosis.
Table 3 IC50 values of Fangke® injection, TTP hydrogel, and TSP hydrogel treatment on 4T1 cells and MCF-7 cells (n = 3)
Treatment group |
IC50 |
4T1 |
MCF-7 |
Fangke® injection, 24 h |
32.067 ± 7.073 |
— |
Fangke® injection, 48 h |
10.442 ± 1.353 |
2.803 ± 0.165 |
Fangke® injection, 72 h |
1.628 ± 0.181 |
— |
TTP hydrogel, 48 h |
0.684 ± 0.042 |
0.398 ± 0.018 |
TSP hydrogel, 48 h |
1.053 ± 0.072 |
0.535 ± 0.033 |
TTP hydrogel + laser, 48 h |
0.071 ± 0.010 |
0.045 ± 0.003 |
TSP hydrogel + laser, 48 h |
0.246 ± 0.034 |
0.137 ± 0.009 |
3.6. Intracellular TF release and remaining TTP/TSP
Theoretically, after the hydrogel is internalized by cells, TF can be quickly released from the ROS-sensitive prodrug after laser irradiation. In order to verify this theory, the intracellular TF content levels released from TTP hydrogel and TSP hydrogel were investigated under laser irradiation and without laser irradiation. As shown in Fig. 6B, the amount of TF released from TTP hydrogel after laser irradiation was significantly higher than without laser irradiation. There was no significant difference between the amount of TF released from TSP after laser irradiation and without laser irradiation. And can be seen from Fig. 6D, the remaining amount of TTP was significantly reduced after laser irradiation, and the residual amounts of TSP were not significantly different with and without laser irradiation. This phenomenon indicated that the intracellular ROS and the ROS generated by TTP under laser irradiation accelerated the release of TF from the ROS-sensitive prodrug TTP. According to the negative correlation between the TTP content and TF content released from TTP under laser irradiation in cells, it can be inferred that the cells internalized TTP dimers. Moreover, the amounts of hydrogels internalized by cells were time- and concentration-dependent (Fig. 6B–E).
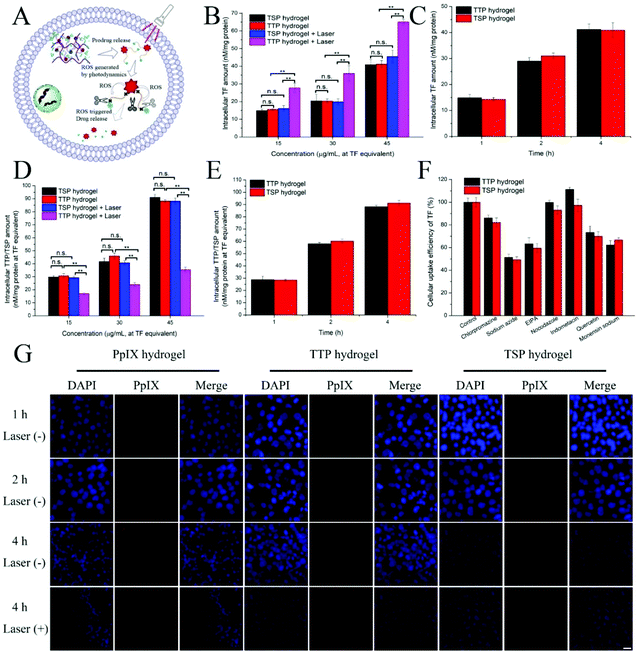 |
| Fig. 6 Intracellular TF release and the 4T1 cell cellular uptake (n = 3). (A) A schematic diagram of a laser promoting TF release from the prodrug hydrogel in tumor cells. (B) TF levels in 4T1 cells co-incubated with TTP hydrogel and TSP hydrogel with/without laser irradiation. (C) TF levels in 4T1 cells after co-incubation with hydrogels for 1, 2, and 4 h. (D) The remaining TTP and TSP in 4T1 cells co-incubated with hydrogels with/without laser irradiation. (E) The remaining TTP and TSP in 4T1 cells after co-incubation with hydrogels for 1, 2, and 4 h. (F) TF levels in 4T1 cells after co-incubation with different inhibitors for 4 h. (G) The cellular uptake of hydrogels with/without laser irradiation imaged via CSLM. *: p < 0.05, significant difference; **: p < 0.01, extremely significant difference; n.s.: p > 0.05, no significance. Scale bar = 10 μm. | |
3.7. Cellular uptake
After the cells were incubated with TTP hydrogel for 4 h, the fluorescence intensity of cells irradiated with a laser was higher than from those without laser irradiation (Fig. 6G and Fig. S13†). This showed that after 630 nm laser irradiation, TTP was affected by ROS and PpIX was released, thereby enhancing the fluorescence intensity. This phenomenon is consistent with the in vitro fluorescence spectra results relating to PpIX, TTP, and TSP (Fig. 1F, and Fig. S7A, C†).
The cellular uptake mechanism was studied via incubating cells with various uptake inhibitors. Chlorpromazine can inhibit clathrin-mediated endocytosis; sodium azide is an energy inhibitor, which can reduce the production of intracellular adenosine triphosphate (ATP); EIPA can inhibit Na+/H+ exchange and macropinocytosis; nocodazole can inhibit F-action polymerization and induce actin depolymerization; indomethacin can inhibit caveolin-mediated endocytosis; quercetin can inhibit clathrin-independent and caveolin clathrin-independent-mediated endocytosis; monensin sodium can destroy the Golgi apparatus and inhibit protein transport in cells. It can be seen from Fig. 6F that the inhibitory effects of chlorpromazine, quercetin and monensin sodium on cellular uptake were relatively weak, while sodium azide and EIPA had potent inhibitory effects on cellular uptake. Indomethacin and nocodazole hardly reduced the uptake amounts of TTP and TSP hydrogels by cells, indicating that the uptake of hydrogels mainly involved energy pathways, Na+/H+ exchange, and micropinocytosis. It was also affected by clathrin-mediated endocytosis, clathrin-independent and caveolin-independent-mediated endocytosis, and protein transport. Therefore, the cellular uptake of TTP and TSP hydrogels was an energy-dependent process mediated by clathrin, clathrin-independent, and caveolin-independent endocytosis, involving Na+/H+ exchange and macropinocytosis.
3.8. TF and its prodrug concentration in rat plasma
In order to evaluate the new TF prodrugs (TTP and TSP) more comprehensively, their in vivo pharmacokinetics were studied. Tegafur can be rapidly metabolized in vivo, and its plasma t1/2 in rats is only about 2.6 h,40 which is consistent with our research, as shown in Fig. 7A and Table 4. After TF was synthesized in the form of prodrugs (TTP and TSP), the t1/2 values increased to 3.98 ± 0.62 h and 4.27 ± 0.60 h, respectively. Also, the area under the plasma concentration–time curves (AUC0–t) increased 2.52- and 1.75-fold, respectively. The higher AUC and t1/2 values can increase the possibility that TF reaches tumor sites. When TTP and TSP were prepared as hydrogels, it took a certain amount of time for TTP and TSP to enter tumor cells after they were released from the hydrogels. The increase in circulation time in vivo allows as much TTP or TSP as possible to enter the tumor cells to exert their effects. The t1/2 values of TTP and TSP were 3.47 ± 0.69 h and 3.38 ± 0.41 h, and their circulation times in vivo were extended to 36 h (Fig. 7B), which can ensure that the majority of TTP and TSP released from the hydrogels is internalized by tumor cells before being degraded.
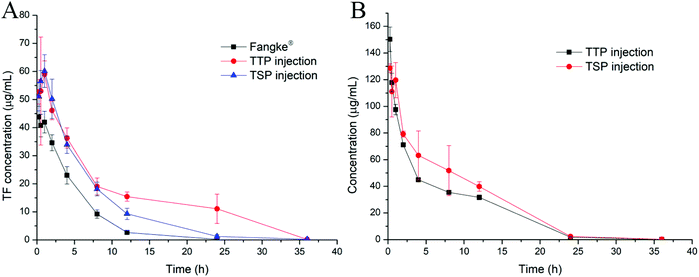 |
| Fig. 7 Pharmacokinetics study using SD rats (n = 6). (A) The TF plasma concentration-time curves based on Fangke®, TTP injection, and TSP injection treatment. (B) The TTP and TSP plasma concentration-time curves based on TTP injection and TSP injection treatment. | |
Table 4 Pharmacokinetic parameters of TF, TTP, and TSP from different injection treatments (n = 6)
Detected |
AUC0–t (μg mL−1 h−1) |
t
1/2 (h) |
MRT0–t (h) |
CL (mL h−1 g−1) |
TF from Fangke® |
243.29 ± 19.25 |
2.76 ± 0.35 |
4.11 ± 0.15 |
0.21 ± 0.02 |
TF from TTP injection |
612.39 ± 55.80 |
3.98 ± 0.62 |
11.07 ± 0.51 |
0.08 ± 0.01 |
TF from TSP injection |
425.41 ± 42.21 |
4.27 ± 0.60 |
5.82 ± 0.57 |
0.12 ± 0.01 |
TTP from TTP injection |
834.94 ± 13.96 |
3.47 ± 0.69 |
6.71 ± 0.03 |
0.18 ± 0.00 |
TSP from TSP injection |
1042.74 ± 127.13 |
3.38 ± 0.41 |
7.01 ± 0.29 |
0.15 ± 0.02 |
3.9. Biocompatibility, biodegradability, and tissue distribution
Crucial criteria for biomaterials are that they maintain good biocompatibility and biodegradability. As indicated in Fig. S14,† no obvious histological changes were found in tumor tissue samples from the normal saline group and the blank hydrogel group, indicating that the hydrogel had excellent biocompatibility and would not cause tissue inflammation. The biodegradability of the hydrogel was confirmed, as more than 80% of the hydrogel was degraded within 7 days intratumorally, as shown in Fig. S15.†
After TTP hydrogel and TSP hydrogel released their prodrugs, the distributions of TTP and TSP in vivo determine their anti-tumor effects. The ex vivo distributions of PpIX hydrogel, TTP hydrogel, and TSP hydrogel after intratumoral injection were studied. As shown in Fig. 8A, within 3 days of intratumoral injection, fluorescence signals were only observed in tumors after treatment with PpIX hydrogel, TTP hydrogel, and TSP hydrogel. On the 5th day, the fluorescence signals were mainly focused in the tumors that were treated with TTP hydrogel and TSP hydrogel, and there was slight fluorescence signal metastasis. The permeability of the tumor tissue ensured that TTP and TSP could be quickly and evenly distributed in the tumor tissue after being released from the hydrogels.41,42 The long residence-time of TTP hydrogel and TSP hydrogel enabled as many prototypical dimers to enter the tumor cells and then exerted their efficacy.43 The concentrations of TF, TTP, and TSP in the plasma, major organs, and tumors of tumor-bearing mice after intratumoral injection are shown in Table S1.† Neither TF nor TTP nor TSP was detected in the plasma and major organs, indicating that after the intratumoral injection of the hydrogels, the hydrogels did not spread to major organs due to biological barriers. The slow release from the hydrogels and the rapid degradation of TTP and TSP in plasma resulted in no drugs being detected in plasma.
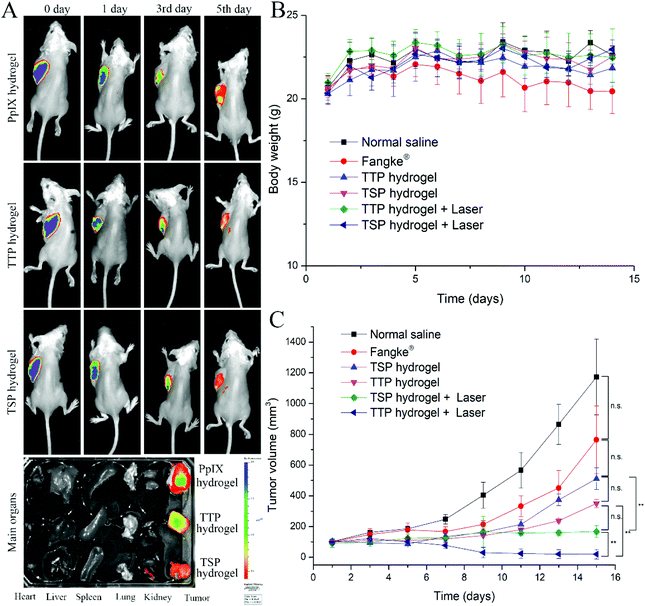 |
| Fig. 8 Hydrogel biodistribution and pharmacodynamics studies (n = 6). (A) The in vivo live imaging of tumor-bearing mice after in situ injection with DiR-loaded PpIX hydrogel, TTP hydrogel, and TSP hydrogel after 0, 1, 3, and 5 days, and the ex vivo imaging of major organs 5 days after in situ injection. (B) Body weight changes of mice during treatment. (C) Tumor volume changes of mice during treatment. | |
3.10. PDT- and chemotherapy-mediated tumor apoptosis in vivo
ROS play a vital role in the occurrence, development, and apoptosis of tumor cells. Low levels of ROS can promote tumor-cell growth, while high levels of ROS can cause tumor-cell apoptosis.44,45 Studies have shown that ROS have a synergistic anti-tumor effect with chemotherapy.46,47 In order to explore the effects of chemotherapy and PDT, the anti-tumor effects of TTP hydrogel and TSP hydrogel under laser irradiation and without laser irradiation were investigated, and the market preparation of the tegafur injection Fangke® was used as a control to evaluate the anti-tumor efficacies. As shown in Fig. 8C, tumor sizes in the normal saline group and Fangke® group snowballed during treatment. TF has a weak therapeutic effect on tumors, and it is often used in combination with other anti-tumor drugs, such as uracil, paclitaxel, and 7-ethyl-10-hydroxycamptothecin (SN-38), to treat tumors.48 Compared with the Fangke® group, the anti-tumor efficacies in the TTP hydrogel group, TSP hydrogel group, TTP hydrogel with laser group, and TSP hydrogel with laser group were improved, as demonstrated in Fig. 8C and Fig. 9A–D.
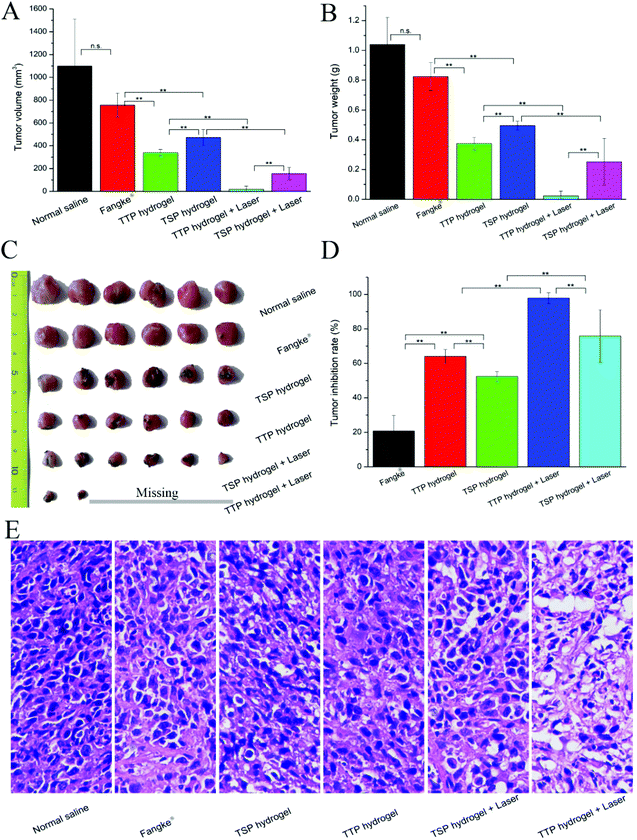 |
| Fig. 9 Pharmacodynamics and histological studies (n = 6). (A) Tumor volumes after dissection. (B) Tumor weights after dissection. (C) Tumor imaging after 14 days of treatment. (D) Tumor inhibition rates calculated based on tumor weights. (E) Histological investigations via H&E staining after 14 days of treatment. | |
The tumor volumes and weights from mice in the TTP hydrogel with laser irradiation group and TSP hydrogel with laser irradiation group were significantly reduced in comparison with the non-laser treatment groups (Fig. 9A and B). The increase in the tumor inhibition rate in the TTP hydrogel with laser irradiation group (Fig. 9D) should be attributed to the synergistic effect of chemotherapy and PDT. In addition, TTP and TSP produced formaldehyde while releasing TF in cells; these are synergistically chemotherapeutic. With and without laser irradiation, the chemotherapeutic efficiencies of TTP hydrogel were apparently better than those of TSP hydrogel. This may be because the AUC of TF released by TTP was higher than that released by TSP (Table 4). After 14 days of treatment, some tumors in the TTP hydrogel with laser irradiation group disappeared completely (Fig. 9C), and large areas of necrosis appeared in the tumor tissue (Fig. 9E and Fig. S14†); this proved that the chemotherapeutic drug TF was quickly released from TTP intratumorally after laser irradiation, and it acted synergistically with PDT to maximize the anti-tumor efficiency. The weight loss of mice in the Fangke® treatment group indicated that the intravenous administration of Fangke® had slight side effects on the tumor-bearing mice (Fig. 8B), which was confirmed by H&E analysis of the main organs, as shown in Fig. S16,† where slight splenic toxicity and nephrotoxicity were found in the Fangke® group.
4. Conclusions
A ROS-sensitive TF-PpIX heterodimer TTP was synthesized and loaded into an intratumorally injectable thermosensitive hydrogel. Under laser irradiation, the high levels of ROS generated by the PpIX portion accelerated the cleavage of the dimer. The released TF and generated ROS synergistically supported chemotherapy and photodynamic therapy, thereby enhancing the anti-tumor efficiency. The laser-irradiation-responsive and long-term drug release allowed TTP hydrogel to achieve on-demand drug administration and significantly reduced the systemic toxicity of the chemotherapy drug. Precise intratumoral drug delivery improved compliance, while greatly improving the anti-tumor efficacy. Anti-tumor experiments in vitro and in vivo showed that TF-mediated chemotherapy combined with PpIX-mediated photodynamic therapy resulted in extremely high synergistic tumor suppression. The efficacy of the chemotherapy drug TF, which is often used in combinational chemotherapy or adjuvant chemotherapy agent due to its poor therapeutic effects, was dramatically enhanced. In short, the TTP thermosensitive intratumorally injectable hydrogel possesses great potential for anti-tumor chemotherapy and can provide an effective strategy for breast-cancer treatment.
Conflicts of interest
There are no conflicts to declare.
Acknowledgements
This work was supported by the Basic Research Projects of Liaoning Provincial Education Department (L2021LQN001) and the Disruptive Technologies Innovation Fund of Shenyang Pharmaceutical University (DFJJ2018208).
References
- F. Bray, J. Ferlay, I. Soerjomataram, R. L. Siegel, L. A. Torre and A. Jemal, CA-Cancer J. Clin., 2018, 68, 394–424, DOI:10.3322/caac.21492.
- W. Chen, K. Sun, R. Zheng, H. Zeng, S. Zhang, C. Xia, Z. Yang, H. Li, X. Zou and J. He, Chin. J. Cancer Res., 2018, 30, 1–12, DOI:10.21147/j.issn.1000-9604.2018.01.01.
- S. Allis, A. Reali, G. Mortellaro, F. Arcadipane, S. Bartoncini and M. G. R. Redda, Tumori J., 2012, 98(5), 543–549 CrossRef.
- K. A. Hartmann, I. Waap, W. Audretsch, K. Muskalla, M. Rezai, J. von der Haar and G. Schmitt, Oncol. Rep., 1998, 5(2), 503–506 CAS.
- D. R. Holmes and R. Zimmerman, J. Surg. Oncol., 2017, 116(7), 824–830, DOI:10.1002/jso.24734.
- Q. Chen, Y. Yang, X. Lin, W. Ma, G. Chen, W. Li, X. Wang and Z. Yu, Chem. Commun., 2018, 54(42), 5369–5372, 10.1039/c8cc02791a.
- C. X. Yang, L. Xing, X. Chang, T. J. Zhou, Y. Y. Bi, Z. Q. Yu, Z. Q. Zhang and H. L. Jiang, Mol. Pharm., 2020, 17(4), 1300–1309, DOI:10.1021/acs.molpharmaceut.9b01318.
- Y. Sun, W. Ma, Y. Yang, M. He, A. Li, L. Bai, B. Yu and Z. Yu, Asian J. Pharm. Sci., 2019, 14(6), 581–594, DOI:10.1016/j.ajps.2019.04.005.
- L. Tang, Z. Wang, Q. Mu, Z. Yu, O. Jacobson, L. Li, W. Yang, C. Huang, F. Kang, W. Fan, Y. Ma, M. Wang, Z. Zhou and X. Chen, Adv. Mater., 2020, 32, 33, DOI:10.1002/adma.202002739.
- J.-X. Zhou and L.-Z. Lin, Chin. J. Integr. Tradit. West. Med., 2008, 28(12), 1133–1140 Search PubMed.
- S.-K. Low, S. Chung, A. Takahashi, H. Zembutsu, T. Mushiroda, M. Kubo and Y. Nakamura, Cancer Sci., 2013, 104(8), 1074–1082, DOI:10.1111/cas.12186.
- Z. Zhang, M. Yu, T. An, J. Yang, M. Zou, Y. Zhai, W. Sun and G. Cheng, Pharm. Res., 2019, 37(1), 4, DOI:10.1007/s11095-019-2709-1.
- W. Kelder, G. A. P. Hospers and J. T. M. Plukker, Expert Rev. Anticancer Ther., 2006, 6(5), 785–794, DOI:10.1586/14737140.6.5.785.
- Y.-F. Xiao, F.-F. An, J.-X. Chen, J. Yu, W.-W. Tao, Z. Yu, R. Ting, C.-S. Lee and X.-H. Zhang, Small, 2019, 15(38) DOI:10.1002/smll.201903121.
- Y. Yang, Y. Yu, H. Chen, X. Meng, W. Ma, M. Yu, Z. Li, C. Li, H. Liu, X. Zhang, H. Xiao and Z. Yu, ACS Nano, 2020, 14(10), 13536–13547, DOI:10.1021/acsnano.0c05541.
- J. Tian, B. Huang, M. H. Nawaz and W. Zhang, Coord. Chem. Rev., 2020, 420, 213410, DOI:10.1016/j.ccr.2020.213410.
- D. Kessel, J. Clin. Med., 2019, 8(10), 1581, DOI:10.3390/jcm8101581.
- W. Ma, S.-N. Sha, P.-L. Chen, M. Yu, J.-J. Chen, C.-B. Huang, B. Yu, Y. Liu, L.-H. Liu and Z.-Q. Yu, Adv. Healthcare Mater., 2020, 9(1), 1901100, DOI:10.1002/adhm.201901100.
- C. Luo, B. Sun, C. Wang, X. Zhang, Y. Chen, Q. Chen, H. Yu, H. Zhao, M. Sun, Z. Li, H. Zhang, Q. Kan, Y. Wang, Z. He and J. Sun, J. Controlled Release, 2019, 302, 79–89, DOI:10.1016/j.jconrel.2019.04.001.
- X. Dong, C. Wei, T. Liu, F. Lv and Z. Qian, ACS Appl. Mater. Interfaces, 2016, 8(8), 5104–5113, DOI:10.1021/acsami.5b11493.
- H. Cheng, R.-R. Zheng, G.-L. Fan, J.-H. Fan, L.-P. Zhao, X.-Y. Jiang, B. Yang, X.-Y. Yu, S.-Y. Li and X.-Z. Zhang, Biomaterials, 2019, 188, 1–11, DOI:10.1016/j.biomaterials.2018.10.005.
- W. Song, J. Kuang, C.-X. Li, M. Zhang, D. Zheng, X. Zeng, C. Liu and X.-Z. Zhang, ACS Nano, 2018, 12(2), 1978–1989, DOI:10.1021/acsnano.7b09112.
- T. J. Zhou, L. Xing, Y. T. Fan, P. F. Cui and H. L. Jiang, J. Controlled Release, 2019, 309, 82–93, DOI:10.1016/j.jconrel.2019.07.028.
- J. Wang, Y. Zhang, N. Jin, C. Mao and M. Yang, ACS Appl. Mater. Interfaces, 2019, 11(12), 11136–11143, DOI:10.1021/acsami.8b21488.
- S. Gou, Y. Huang, Y. Wan, Y. Ma, X. Zhou, X. Tong, J. Huang, Y. Kang, G. Pan, F. Dai and B. Xiao, Biomaterials, 2019, 212, 39–54, DOI:10.1016/j.biomaterials.2019.05.012.
- B. Crivelli, S. Perteghella, E. Bari, M. Sorrenti, G. Tripodo, T. Chlapanidas and M. L. Torre, Soft Matter, 2018, 14(4), 546–557, 10.1039/c7sm01631j.
- J. Wu, K. Zheng, X. Huang, J. Liu, H. Liu, A. R. Boccaccini, Y. Wan, X. Guo and Z. Shao, Acta Biomater., 2019, 91, 60–71, DOI:10.1016/j.actbio.2019.04.023.
- H. Xu, S. Huang, J. Wang, Y. Lan, L. Feng, M. Zhu, Y. Xiao, B. Cheng, W. Xue and R. Guo, Int. J. Biol. Macromol., 2019, 137, 433–441, DOI:10.1016/j.ijbiomac.2019.06.246.
- P. Pankongadisak and O. Suwantong, RSC Adv., 2018, 8(70), 40219–40231, 10.1039/c8ra07255h.
- A. Li, J. Zhao, J. Fu, J. Cai and P. Zhang, Asian J. Pharm. Sci., 2019 DOI:10.1016/j.ajps.2019.08.001.
- R. Zhang, X. Q. Song, R. P. Liu, Z. Y. Ma and J. Y. Xu, J. Med. Chem., 2019, 62(9), 4543–4554, DOI:10.1021/acs.jmedchem.9b00128.
- D. Engel, A. Nudelman, N. Tarasenko, I. Levovich, I. Makarovsky, S. Sochotnikov, I. Tarasenko and A. Rephaeli, J. Med. Chem., 2008, 51(2), 314–323, DOI:10.1021/jm7009827.
- S. Sardar, S. Chaudhuri, P. Kar, S. Sarkar, P. Lemmens and S. K. Pal, Phys. Chem. Chem. Phys., 2015, 17(1), 166–177, 10.1039/c4cp03749a.
- D. M. Chipman and N. Sharon, Science, 1969, 165(3892), 454–465, DOI:10.1126/science.165.3892.454.
- D. Mawad, C. Warren, M. Barton, D. Mahns, J. Morley, B. T. Pham, N. T. Pham, S. Kueh and A. Lauto, Carbohydr. Polym., 2015, 121, 56–63, DOI:10.1016/j.carbpol.2014.12.008.
- I. Petit, M. Szyper-Kravitz, A. Nagler, M. Lahav, A. Peled, L. Habler, T. Ponomaryov, R. S. Taichman, F. Arenzana-Seisdedos, N. Fujii, J. Sandbank, D. Zipori and T. Lapidot, Nat. Immunol., 2002, 3(7), 687–694, DOI:10.1038/ni813.
- J. Wang, X. Sun, W. Mao, W. Sun, J. Tang, M. Sui, Y. Shen and Z. Gu, Adv. Mater., 2013, 25(27), 3670–3676, DOI:10.1002/adma.201300929.
- C. Xiao, J. Ding, L. Ma, C. Yang, X. Zhuang and X. Chen, Polym. Chem., 2015, 6(5), 738–747, 10.1039/c4py01156b.
- W.-X. Qiu, M.-K. Zhang, L.-H. Liu, F. Gao, L. Zhang, S.-Y. Li, B.-R. Xie, C. Zhang, J. Feng and X.-Z. Zhang, Biomaterials, 2018, 161, 81–94, DOI:10.1016/j.biomaterials.2018.01.037.
- I. Yamamiya, K. Yoshisue, Y. Ishii, H. Yamada and K. Yoshida, J. Pharm. Pharmacol., 2014, 66(12), 1686–1697, DOI:10.1111/jphp.12304.
- A. Dhaliwal and G. Zheng, Theranostics, 2019, 9(26), 8091–8108, DOI:10.7150/thno.37204.
- V. Torchilin, Adv. Drug Delivery Rev., 2011, 63(3), 131–135, DOI:10.1016/j.addr.2010.03.011.
- F. Chen, F. Zhang, D. Shao, W. Zhang, L. Zheng, W. Wang, W. Yang, Z. Wang, J. Chen, W.-F. Dong, F. Xiao and Y. Wu, Appl. Mater. Today, 2020, 19, 100558, DOI:10.1016/j.apmt.2019.100558.
- F. Weinberg, N. Ramnath and D. Nagrath, Cancers, 2019, 11(8), 1191, DOI:10.3390/cancers11081191.
- P. Jia, C. Dai, P. Cao, D. Sun, R. Ouyang and Y. Miao, RSC Adv., 2020, 10(13), 7740–7750, 10.1039/c9ra10539e.
- J. Li, Y. Liu, X. Li, G. Liang, C. Ruan and K. Cai, Nanoscale Horiz., 2020, 5(2), 350–358, 10.1039/c9nh00490d.
- W. Yin, W. Ke, W. Chen, L. Xi, Q. Zhou, J. F. Mukerabigwi and Z. Ge, Biomaterials, 2019, 195, 63–74, DOI:10.1016/j.biomaterials.2018.12.032.
- J. Kitayama, H. Ishigami, H. Yamaguchi and S. Emoto, Ann. Surg. Oncol., 2013, 20, S34–S35 Search PubMed.
Footnote |
† Electronic supplementary information (ESI) available. See DOI: 10.1039/d0bm01519a |
|
This journal is © The Royal Society of Chemistry 2021 |
Click here to see how this site uses Cookies. View our privacy policy here.