DOI:
10.1039/D0BM01172J
(Paper)
Biomater. Sci., 2021,
9, 238-251
Optimizing microenvironment by integrating negative pressure and exogenous electric fields via a flexible porous conductive dressing to accelerate wound healing†
Received
14th July 2020
, Accepted 6th November 2020
First published on 10th November 2020
Abstract
Wound healing is a complex and sequential biological process that involves multiple stages. Current treatments for nonhealing or chronic wounds are unsatisfactory as they exert a single effect on one specific activity. Herein, we constructed a silver nanowire (AgNW)-based, three-dimensional (3D), porous foam dressing that is flexible and conductive. This conductive foam dressing was composed of AgNWs modified with a stable hydrophobic coating and porous polyurethane (PU), providing a skeleton to support the 3D conductive networks. The AgNWs-PU foam dressing exhibited favorable biocompatibility, outstanding electrical properties, excellent bending-compression durability, and long-term stability under wet conditions, making it suitable for wound treatment. Via the conductive foam dressing, negative pressure and exogenous wound directional electric fields (EFs) could be integrated for simultaneous implementation, and the artificial jointly constructed microenvironment promoted wound healing in a system. This novel “all-in-one” device presented intrinsic multifunctionality, including the drainage of pus and necrotic tissue, mitigation of inflammation, promotion of cell proliferation, direction of keratinocyte migration, and induction of angiogenesis. An immunohistochemical assay and western blot analysis illustrated that the angiogenesis and cell proliferation pathways in the tissue were significantly activated when this novel therapy was adopted. More importantly, the practical performance of this “all-in-one” device was demonstrated by assessment of full-thickness defect wounds in model pigs. Comparing the percentage of residual wound area after administration of traditional treatment (25.82 ± 3.52%) and the novel treatment (3.07 ± 1.23%) demonstrated the promising applications of this novel treatment in clinical wound healing.
1. Introduction
Wound healing results from overlapping and interacting biological processes, including hemostasis, inflammation, proliferation, and remodeling.1,2 Any disorder in these processes will lead to chronic or even nonhealing wounds.3 Damage to the barrier function generates an unsealed wound, preventing protection against further environmental damage or pathogens, causing health issues in patients.4 Thus, timely and effective therapies for wound healing are urgently needed. Currently, a large number of modern biomaterials and medical technologies have been designed for medical treatments, and can be classified by function: debridement,5,6 antibacterial effects,7,8 promotion of cell proliferation,9 and improvement of local perfusion.10,11 However, these therapies are limited because they have a single specific function, and comprehensive strategies to improve wound healing are laking. Therefore, it is highly imperative to develop an ideal treatment with multifunctionality and multitargeted applications to accelerate wound healing.
Chronic wounds characterized by long-lasting inflammation obstruct even stop the healing process.12,13 The management of the wound microenvironment to accelerate transition of the healing phase from inflammation to proliferation and remodeling plays a vital role in wound treatment.14 Negative pressure wound therapy (NPWT) is believed to be an effective method because it supports the adequate drainage of pus and necrotic tissue, mitigates inflammation, and enhances perfusion by continuous negative pressure suction.15–17 However, when wounds enter the proliferation and remodeling stage, sufficient keratinocyte proliferation to migrate from the wound margin to the center is needed to achieve re-epithelialization. In most cases, re-epithelialization failure is due to impaired keratinocyte migration rather than inadequate proliferation.18 Many recent studies on keratinocyte migration focused on cell behavior control demonstrate that wound EFs are an overriding signal that directs the cell migration.19,20 When a wound occurs, lateral wound EFs are formed from the surrounding intact tissue toward the center of the wound. The electrical signals provided by wound EFs can mediate the redistribution of various receptors and kinases, trigger relevant molecular signal activation, and promote cell directional protrusion.21 In turn, abnormal wound EFs or their tendency to collapse are the crucial causes of difficulty in the repair of problematic wounds.22 Thus, the synergistic combination of NPWT and exogenous directed wound EFs is extremely likely to successfully accelerate wound healing. Moreover, considering that the four wound healing phases overlap and interact, these two methods should exert simultaneous implementation. NPWT serves as an exudate drainage and inflammation control system, while exogenous wound EFs enhance or replenish the electrical signals to facilitate re-epithelialization. Construction of an artificial wound microenvironment that supports drainage, controls inflammatory, and facilitates proliferation and re-epithelialization within one system is a promising and novel strategy. Notably, although electrical stimulation (ES) has become a mature clinical therapy, a great difference between ES and wound EFs remains. Compared with wound EFs, ES therapy has no constant direction or intensity, which greatly limits its ability to direct keratinocyte migration. Thus, an updated strategy should apply exogenous directed EFs, the direction of which is consistent with lateral wound EFs. However, this combination is difficult to achieve due to limitation of implementation path of exogenous wound EFs. Conventional metal electrodes cause unsatisfactory contact in terms of a mechanical mismatch with human tissues. Moreover, the wound exudate is extremely likely to corrode the electrode, leading to unstable conduction and toxic degradation products.
The key to combining NPWT and exogenous EFs in wound treatment is constructing a flexible conductive foam dressing to realize both draining necrosis and the application of annular oriented EFs on wounds. Existing electrically conductive materials, including conductive polymers,23 carbon nanotubes,24,25 and graphene,26,27 can be used as conductive components to prepare flexible conductive materials, but the exogenous EF applications mediated by those materials have limited effects since the use of only a 2D network,28 a substantially reduced conductivity by due to shape deformation,29,30 or difficulty in forming long-term stable and conformal interfaces between the two material types.31 As highly conductive metallic nanowires, silver nanowires (AgNWs) offer potential applications in flexible conductive foam dressing due to their high conductivity, flexibility modification, mechanical robustness, and low-cost processing.32–34 More importantly, silver has been demonstrated to exhibit excellent antibacterial activity and, is more toxic to microorganisms and less toxic to mammalian cells.35 Therefore, AgNWs are most suitable as conductive components to construct conductive foam dressing. In addition, the polyurethane (PU) sponge which has been used in NPWT, has an interconnected and porous structure and can act as a skeleton to support 3D conductive networks. Besides, the instability of silver nanowires polyurethane (AgNWs-PU) under wet conditions can be modified with hydrophobic surface modification techniques, such as 1H,1H,2H,2H-perfluorooctyltriethoxysilane (TPFS) coating.
Herein, we constructed a highly conductive, flexible, AgNWs-PU foam wound dressing via a solution-based method. With AgNWs-PU as the conductive section and ordinary PU foam dressing as nonconductor, annular exogenous EFs whose negative pole is in the wound center and positive pole is in the wound margin can be successfully applied on wounds. Based on its porous structure, bending-compression durability, and long-term stability under wet conditions, NPWT can be simultaneously applied. This “all-in-one” treatment system could optimize the artificial wound microenvironment at multiple stages of the wound healing process. NPWT reduced inflammation during the inflammatory phase, while exogenous wound EFs stimulated cell proliferation and angiogenesis during the proliferation phase and electrical signals could direct the keratinocyte migration to achieve re-epithelialization during the remodeling phase. This concise and efficient therapy can avoid the necessity of frequent dressing changes, decreasing the cost and difficulty of patient care. In addition, the conductive and nonconductive PU foam dressing can be freely assembled according to the shape and size of the wound (Fig. 1E), leading to convenience in clinical practice. Furthermore, enhanced wound healing effects were detected on larger diameter full-thickness skin defects in Bama pigs, indicating that this updated multifunctional therapeutic system offers great clinical application potential.
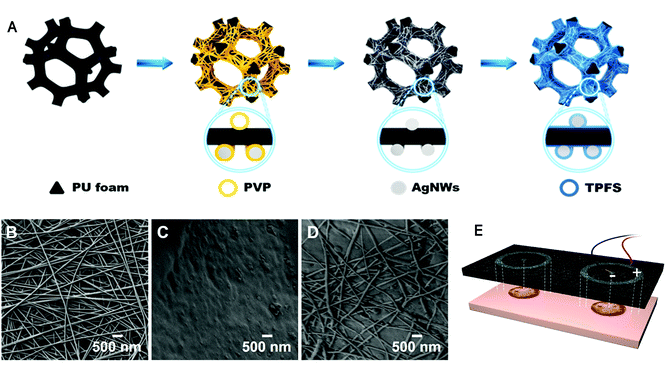 |
| Fig. 1 Synthesis of the conductive foam dressing. (A) Schematic illustration for the synthesis of TPFS-coated AgNWs-PU foam. (B–D) SEM images of AgNWs, bare PU foam, and TPFS-coated AgNWs-PU foam respectively. (E) The conductive and nonconductive foam dressings were freely assembled according to the shape and size of the wounds to apply annular exogenous EFs with a cathode in the wound center and the anode in the wound margin. | |
2. Materials and methods
2.1. Materials
The consumables (PU foam dressings, drainage tubes, adhesive sealing films, effusion containers) and negative-pressure trauma devices that make up the NPWT systems were manufactured by KCI (USA). AgNWs (diameter, 70 nanometers; length, 100–200 microns) were obtained from Pioneer Nanowires (Nanjing, China). Sodium borohydride (NaBH4), isopropanol, and TPFS were purchased from Sigma (USA). Dulbecco's Modified Eagle's medium (DMEM), phosphate-buffered saline (PBS), fetal bovine serum (FBS), and trypsin were all purchased from Gibco (USA). Anti-pPI3K, anti-vascular endothelial growth factor (VEGF), and anti-β-actin antibodies were purchased from Thermo Fisher (USA). Anti-Akt and anti-pAkt antibodies were purchased from Cell Signaling Technology (USA). Anti-PI3K, anti-Ki67, anti-E-cadherin, and anti-CD31 antibodies were purchased from Abcam (USA).
2.2. Preparation of AgNWs-PU
Bare PU foam manufactured by KCI (USA) was cleaned with deionized (DI) water and ethanol three times each, followed by complete drying at 60 °C for 2 h, and cut into small pieces 9.5 × 3 × 1 cm (anode) and 1 × 1 × 3 cm (cathode) in sizes. The AgNW dispersion obtained from Pioneer Nanowires was diluted with isopropanol to approximately 0.2 mg mL−1 before use. AgNW networks on bare PU foam were formed by spraying the diluted AgNW dispersion onto the substrate using an airbrush (Iwata, Japan). The AgNW dispersion was atomized into very small droplets by using pressurized nitrogen (N2). High-pressure N2 flow directed the droplets to penetrate the pores of the bare PU foam below. Keeping the nozzle-to-substrate distance constant, the airbrush spray was moved in a set pattern with respect to the substrate until the whole bare PU foam had been uniformly sprayed with AgNWs, after which the foam was transferred to a dry oven and heated at 60 °C for 30 min. This process was repeated several times until the required network conductivity was achieved. After spraying the AgNW dispersion, the foam was immersed in a 0.5 M NaBH4 solution for 30 min to remove surface polyvinylpyrrolidone (PVP) ligands produced an atomically clean Ag–Ag interface to ensure direct welding of NW–NW junction, which would greatly increasing the conductivity of the AgNW thin films, followed by rinsing with DI water and ethanol three times each and drying in a vacuum oven at 60 °C for 2 h.34 Finally, the obtained samples were immersed in absolute ethanol containing 0.5 vol% TPFS (97%, Sigma-Aldrich) for 30 min and annealed in a vacuum oven at 60 °C for 1 h.36
2.3. Characterization
The sample microstructure was captured using scanning electron microscopy (SEM, Zeiss Crossbeam 340) at an accelerating voltage of 2.0 kV. Energy-dispersive spectroscopy (EDS, Oxford Instruments) and X-ray photoelectron spectroscopy (XPS, ESCALAB 250Xi, Thermo, USA, Al-Kα radiation (h = 1486.6 eV)) were used to identify the purity and distribution of AgNWs. The dissolution of AgNWs was analyzed using inductively coupled plasma mass spectrometry (ICP-MS, Agilent 7800). The conductivity of the samples was measured by the two-probe method with a Keithley 2450 power supply under ambient conditions. Copper pieces were embedded and connected to the prepared the conductive foam with silver paste before infiltration with TPFS. Contact angles of water drops were measured in the air using the sessile drop method (Dataphysics DCAT 21, Germany). The attenuated total reflection Fourier transform infrared spectroscopy (FTIR) spectrum was collected on a Nicolet iS5 instrument at a resolution of 4 cm−1. The Instron 5982 (Instron Company, USA) electronic universal testing machine was used for compressive tests on cubic foams with a size of 30 × 30 × 30 mm at a strain rate of 1 mm min−1.
2.4.
In vitro degradation of AgNWs-PU foam
To determine Ag release, we soaked the TPFS-coated AgNWs-PU foam in PBS (pH 7.4) and measured Ag release by ICP-MS. Briefly, the TPFS-coated AgNWs-PU foam was cut into rectangular pieces with 50 × 50 × 30 mm3 in size, fixed onto the holder, and immersed in 150 mL of PBS at 37 ± 0.5 °C. Aliquots of release medium (5 mL each) were collected at 6 h, 12 h, 24 h, 3 d, 5 d, and 7 d. The solution was replenished immediately after sampling to maintain its volume.
2.5. Live and dead cell staining
Extract liquid from AgNWs-PU foam was made by adding TPFS-coated AgNWs-PU foam to DMEM containing 10% FBS at a ratio of 6 cm3 mL−1 and soaking at 37 °C for 48 h. One milliliter of cell suspension containing 1 × 104 3T3 cells (obtained from ATCC) was added to each well of a 24-well plate and cultured in a 5% CO2 incubator at 37 °C for 24 h. Then, the original medium was discarded, and AgNWs-PU foam extract and blank control solution (DMEM containing 10% FBS) were added to the different groups of wells (1 mL per well). After incubating for 24 h or 48 h, the original medium was discarded and the wells were washed three times with PBS. A live/dead cytotoxicity kit (L3224, Invitrogen) was used to detect the viability of the cells. After observation under a fluorescence microscope, the number of live/dead cells were calculated by ImageJ (National Institutes of Health, USA).
2.6. CCK8 assay
Extract liquid from AgNWs-PU foam was prepared by the method above, and diluted to different concentrations (10%, 50%, and 100%). One hundred microliters of a cell suspension containing 1000 3T3 cells was added to each well of a 96-well plate and cultured in a 5% CO2 incubator at 37 °C for 24 h. Then, the original medium was discarded, and AgNWs-PU foam extract and blank control solution (DMEM containing 10% FBS) were added to the different groups of wells (100 μL per well). After incubation for 48 h, 10 μL of CCK-8 reagent (ab228554, Abcam) was added to each well. The absorbance (optical density (OD)) at 450 nm and 650 nm was measured using an automatic microplate reader and used to calculate the cell proliferation rate at 6 h after the addition of CCK-8 reagent.
2.7. Pig characteristics and surgical approaches
Sixteen male Bama pigs aged 8–12 weeks, weighing 15–20 kg each whose skin was in good condition were used to establish an animal model. The pigs were housed in a pathogen-free, and temperature- and humidity-controlled facility with a 12 h day–night cycle in the vivarium at the animal center of the Third Military Medical University. All experimental procedures were reviewed and approved by the University Committee on Animal Resources. General anesthesia and analgesia procedures were performed based on the Bama pig guidelines provided by the University Committee on Animal Resources.
Bama pigs were anesthetized by the inhalation of 1–2 vol% isoflurane (246885U, Abbott, China), and the depth of anesthesia was adjusted according to the surgical process until the end of the operation. With the pig in prone position and the spine as the axis of symmetry, a square area of skin with dimensions of 30 × 30 cm was selected for hair removal. The skin at a distance of 10 cm from the midline of the forelimbs on the back was prepared for aseptic surgery. A round, vertical, full-thickness skin defect with a diameter of 3 cm was created on both the left and right sides. The wounds were perpendicular to the midline of the spine, 25 cm from the midline of the forelimbs. After hemostasis was achieved, the wounds were cleaned with iodophor. Conductive foam (AgNWs-PU foam) and ordinary PU foam dressings were assembled, as shown in Fig. 7A. The center of the conductive wound dressing in the experimental group (AgNWs-PU/EF) was connected to the negative electrode of the signal generator, and the outer ring of the conductive dressing was connected to the positive electrode, which formed directional EFs from the margin to the center of the wound. Exogenous EFs (70% duty cycle, continuous application) with an applied intensity of +100 mV mm−1 were created in the direction of endogenous EFs of the wound (the wound margin was positive, and the wound center was negative). In the control group (AgNWs-PU), the wound dressing used that in the experimental group was applied, but the power source was not connected. In both the experimental and control groups, a biocompatible semipermeable membrane (KCI, USA) was applied to seal the wound surface and material. The semipermeable membrane was connected to a negative-pressure drainage closure device, and negative-pressure drainage at 75 mmHg was applied. In the blank control group, the wound was covered with ordinary gauze that was replaced every other day. All Bama pigs were reared in cages at room temperature (24 °C) and under 65% humidity and were provided sufficient food and drinking water. On the 7th day, five pigs were randomly selected from each group (5 wounds per group); the wound status was observed and recorded and wound tissues were collected for observation and analysis. The remaining 8 Bama pigs underwent dressing and electrode replacement and were kept in cages. On the 14th day after wounding, wound tissues from the remaining pigs were collected for observation and analysis.
2.8. Wound EFs detection
One hour after wounding, the EFs across the wound of all groups were detected. The positive and negative probes were spaced 1 mm apart to measure the EFs at different locations throughout the wound, including an area of normal skin, the wound center, and the wound margin. Before exogenous EFs were applied through the AgNWs-PU foam, the endogenous EFs of the wound margin was detected. After the signal generator was connected, the voltage was adjusted until the wound EFs value increased to 100 mV mm−1 for more than 3 times. Eight hours after wound formation, wound margin EFs were detected again in all groups. Similarly, on the 2nd, 3rd, 4th, 7th, and 14th day, we detected the wound margin EFs in all groups.
2.9. Western blot assay
After the wound tissues were lysed, the supernatant was collected by centrifugation. Equal quantities of proteins were electrophoresed on 10% SDS-PAGE gels and transferred to a PVDF membrane (Millipore, Corporation, MA), which was blocked and incubated with the primary antibody anti-PI3K (ab232997, Abcam), anti-pPI3K (PA5-104853, Thermo Fisher), anti-Akt (2971, Cell Signaling Technology), anti-pAkt (2972, Cell Signaling Technology), anti-VEGF (MA1-16626, Thermo Fisher) and β-actin (MA5-11866, Thermo Fisher) overnight at 4 °C. After washing, the membranes were incubated for 30 min at room temperature with HRP-conjugated secondary antibodies (G-21040, Thermo Fisher). The labeled proteins were visualized using an ECL kit (WP20005, Thermo Fisher).
2.10. Staining procedure
The wound tissues were soaked in 4% paraformaldehyde for 48 h and then embedded in paraffin for sectioning at 4 μm. After dewaxing, the tissue sections were subjected to hematoxylin and eosin (H&E) or immunohistochemical staining. After blocking in 5% goat serum in PBS for 1 h at room temperature, the sections were incubated with primary antibodies against Ki67 (ab15580, Abcam), K10 (ab9205, Abcam), E-cadherin (ab15148, Abcam), and CD31 (ab28364, Abcam) overnight. After washing three times with PBS, secondary antibodies were added for 1 h (dilution 1
:
100), and finally, an appropriate amount of 3,3′-diaminobenzidine (DAB) was added dropwise to develop the color. The slides were counterstained with Mayer's hematoxylin and then dehydrated and mounted. After observation under a microscope, the results were analyzed by Image–Pro Plus 6.0 (Media Cybernetics, Inc., Rockville, MA, USA).
2.11. Statistical analyses
Statistical differences were analyzed by one-way ANOVA. Figures were created using GraphPad Prism (GraphPad Prism, San Diego, CA). Differences were considered significant at P < 0.05 in all cases.
3. Results and discussion
3.1. Preparation and characterization of AgNWs-PU
The AgNWs-PU foam dressing used in this work was constructed according to the preparation process described in Fig. 1A. Due to the low surface energy of isopropanol and the 3D pore structure of the foam, the bare PU foam was completely infiltrated with the AgNWs solution in isopropanol after spraying. The average diameter of the AgNWs was found to be ∼70 nm (Fig. 1B). After spraying, the PVP insulation layer formed on the surface of the AgNWs limits the current transfer capability of the AgNW thin films. Various methods, including mechanical pressing,36 plasmonic welding,37 photochemical welding,38 and thermal annealing39 have been developed to remove the PVP ligands and produce a clean Ag–Ag interface to improve the conductivity of the AgNW thin films. However, because PU foam substrates cannot withstand these harsh conditions, a room temperature direct welding and chemical protection strategy reported by Ge et al. showed that PVP could be removed with a NaBH4 solution and that the as-formed AgNW thin films could be modified with dodecanethiol.40 In this study, the obtained sample was immersed in a 0.5 M NaBH4 solution for 30 min to remove PVP. To achieve stability under wet conditions, sample preparation was followed by surface modification with a dense and hydrophobic TPFS layer. AgNWs uniformly wrapped the PU foam microfibers (Fig. 1C) to form a conductive sponge with the AgNW nano/micronetwork structure (Fig. 1D). After modification, TPFS-coated AgNWs-PU retained the same pore size as the unmodified pure PU foam dressing (Fig. S1†), which maintained its drainage effect.
The electrical conductivity of the AgNWs-PU foam was regulated by the number of spray-coating cycles. The mass content of AgNWs in the PU foam increased almost linearly with the number of spray-coating cycles, as shown in Fig. 2A. Correspondingly, the resistance of the AgNWs-PU foam gradually decreased from approximately 1156 Ω to approximately 24.5 Ω as the number of spray-coating cycles increased from one to seven (Fig. 2B), which demonstrated that AgNWs-PU foam with various levels of conductivity could be obtained by simply adjusting the number of spray-coating cycles. In addition, the resistance hardly decreased after six spray-coating cycles, and the mass content of the AgNWs was only approximately 2.28 wt%. Good mechanical stability is the key to electrical robustness. To verify the reproducibility of the mechanical properties of the AgNWs-PU foam, stress–strain curves at the same strain rate (1 mm min−1) were obtained under displacement control mode on an electronic universal testing machine. The resultant compressive stress–strain curves in Fig. 2C, show that the stress almost completely returned to the initial point for each strain when the AgNWs-PU foam was unloaded, and very small residual strain was observed even for large strains of 66.7% and 83.3%. This shows that the AgNWs-PU foam exhibits excellent elasticity without plastic deformation. Comparison of Fig. 2C and Fig. S2† shows that the compressive strength of the AgNWs-PU foam was higher than that of bare PU foam, which is attributed to the high elastic modulus of AgNWs. Since the foam dressing was subjected to a pressure of approximately 75 mmHg during treatment, which corresponded to approximately 67% compression deformation, we studied the electrical conductivity under different compression deformations. The results showed that as the compression increased, the resistance decreased, and an ultralow resistance of 3.8 Ω was obtained under 83.3% compression deformation (Fig. 2D).
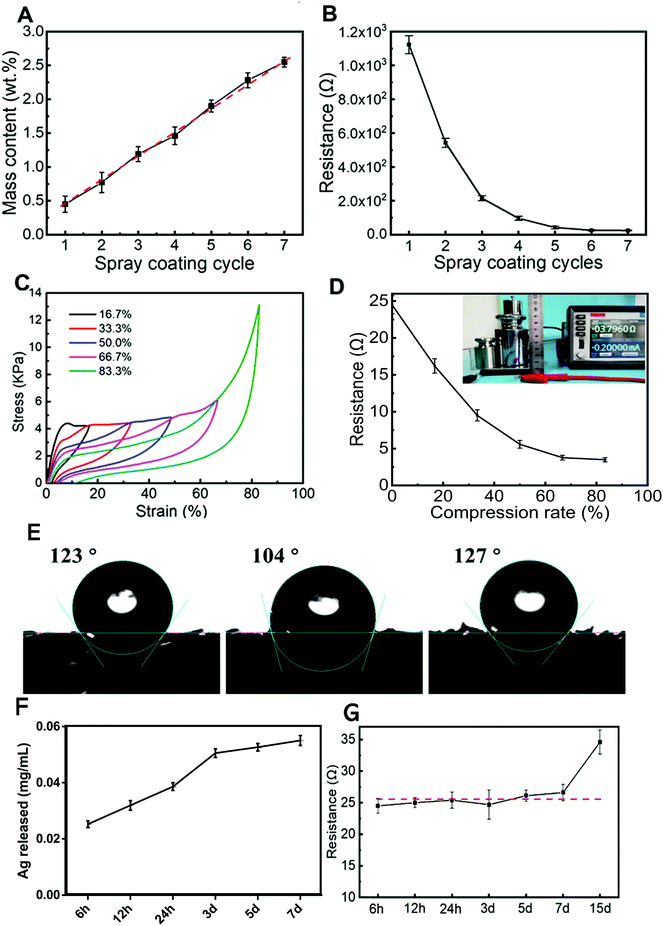 |
| Fig. 2 Physical characterization of the conductive foam dressing. (A) Mass loading of AgNWs in PU foam versus number of spray-coating cycles. (B) Resistance as a function of number of spray-coating cycles. (C) Compressive stress–strain curves of TPFS-coated AgNWs-PU foam under different strains. (D) Resistance variation of TPFS-coated AgNWs-PU foam under different compression conditions. (E) The water contact angle of bare PU foam (left), PU loaded AgNWs foam (middle), and TPFS-coated AgNWs-PU foam (right). (F) The total silver release behavior of TPFS-coated AgNWs-PU foam dressing as a function of immersion time. (G) The resistance of TPFS-coated AgNWs-PU foam as a function of immersion time. | |
The wettability of the foam was evaluated by measuring the water contact angles on the surface by a sessile drop technique. The contact angles for the bare PU foam, PU loaded AgNWs foam, and TPFS-coated AgNWs-PU foam were 123°, 104°, and 127°, respectively (Fig. 2E). TPFS improved the hydrophobicity of the PU foam, which promoted the stability of AgNWs in a wet environment, and the hydrophobicity is also conducive to the antibacterial properties of the foam.41 To study the stability of the AgNWs-PU foam dressing, the release behavior of the AgNWs in PBS was determined by ICP-MS (Fig. 2F). As the soaking time increased, the release of the silver slowly increased, but the total concentration of silver released after 7 days of immersion was only 0.055 mg mL−1. This demonstrated the stability of the composite, which was mainly due to the protection provided by the hydrophobic TPFS coating. This was also supported by the electrical performance, which remained quite stable even after 7 days of immersion (Fig. 2G).
EDS elemental mapping (Fig. 3A) showed the distribution of TPFS-coated AgNWs on the surface of the PU foam. Further elemental information on the AgNWs was obtained by XPS (Fig. 3B). The relevant high-resolution Ag 3d XPS spectra contained two peaks at 368.2 eV and 374.2 eV corresponding to Ag 3d5/2 and Ag 3d3/2 respectively. The highest level of the characteristic metallic silver (Ag0) 3d5/2 binding energy signature was at 368.2 eV, indicating that the AgNWs-PU foam mostly consisted of metallic silver. To understand the protective effect of the TPFS molecules, we investigated the surface properties of the AgNW films using FTIR spectroscopy. Fig. S3† shows the FTIR spectra for bare PU foam, PU loaded AgNWs foam, and TPFS-coated AgNWs-PU foam. The FTIR results suggested that the TPFS molecules were densely packed, and thus notably affecting the hydrophobicity of the film.
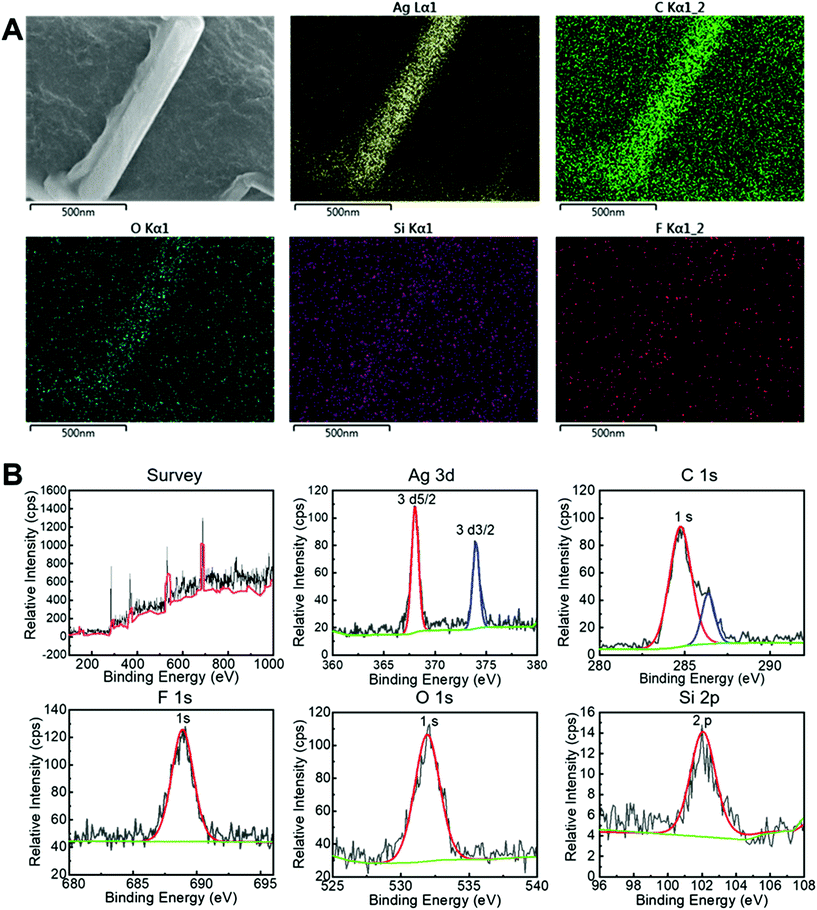 |
| Fig. 3 EDS and XPS of the conductive foam dressing. (A) EDS analyses of TPFS-coated AgNWs-PU foam. (B) XPS spectrum of TPFS-coated AgNWs-PU foam. | |
3.2. Biocompatibility of AgNWs-PU
The cytotoxicity of the AgNWs-PU foam was first studied by live and dead cell staining. The live cells were stained green, and the dead cells were stained red, which was observed with a fluorescence microscope (Fig. 4A). After the extracted liquid of AgNWs-PU foam cultured with cells for 24 h or 48 h, the live cell rates remained extremely high (Fig. 4B), and few dead cells were observed, which was almost the same as the results obtained in blank control group (P > 0.05). Meanwhile, the CCK8 assay was used to detect the cytotoxicity of AgNWs-PU. As shown in Fig. 4C, relative cell growth (RCG) in the AgNWs-PU foam extract was more than 80% at all monitored time points. According to the relationship between the RCG and the cytotoxicity grade specified in the United States Pharmacopeia,42 an RCG ≥80% indicates a cytotoxicity grade of 1, indicating the acceptability of AgNWs-PU foam for application in covering wounds.
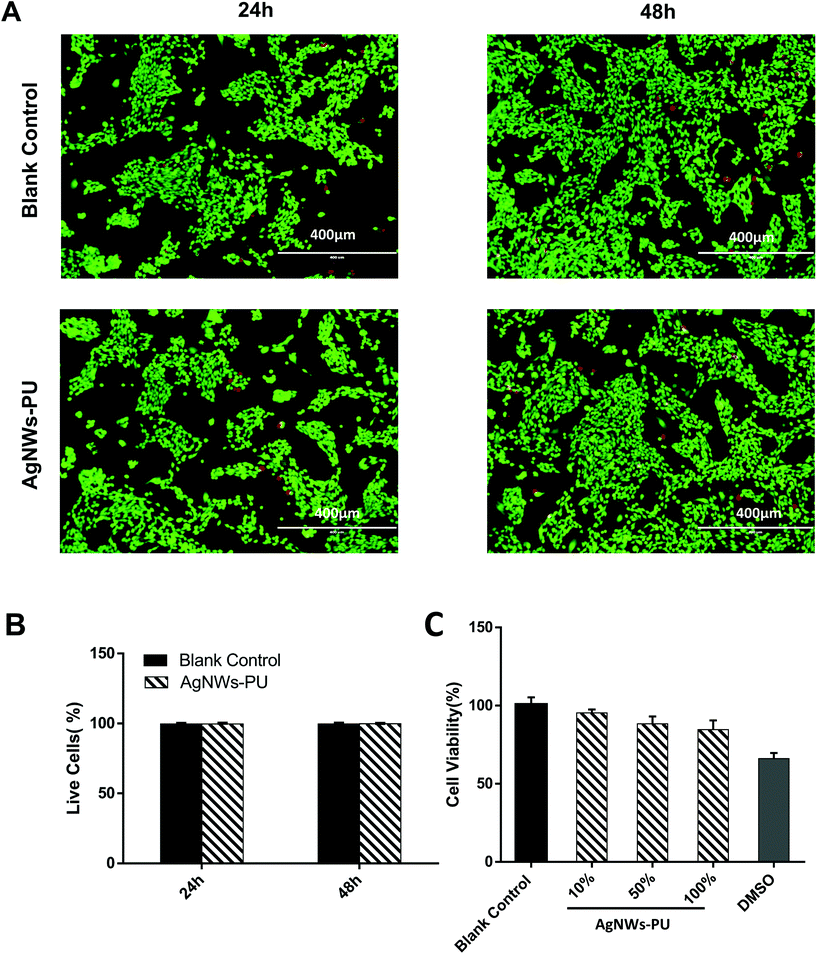 |
| Fig. 4 Biocompatibility of the conductive foam dressing. (A) Live/dead cell staining to detect the effect of AgNWs-PU foam on cell viability. Live cells were stained green, and dead cells were stained red. (B) Quantitative analysis of live/dead cell staining. (C) Detection of TPFS-coated AgNWs-PU foam dressing cytotoxicity by CCK-8 assay. | |
Some studies have reported that silver nanoparticles (AgNPs) display size-, shape-, and surface area-dependent cytotoxicity.43,44 The ultra-high length diameter ratio of rod-shaped AgNWs, which are characterized by a large size but small specific surface area, resulted in a smaller surface area for the release of Ag+. Moreover, the AgNWs were modified with a hydrophobic surface of TPFS, resulting in the enhanced chemical stability of the AgNWs and decreased Ag+ release.45
3.3. Application of exogenous EFs via AgNWs-PU foam
Compared with the direct application of direct current, a 70% pulse current decreased the Joule effect while maintaining the EF strength to a level equivalent to that of direct current EFs. One hour after wound creation, the EFs of the wound margin were detected. The center of each circular wound with a diameter of 3 cm was used as the origin (X = 0, Y = 0) and a square area with dimensions of 4.5 × 4.5 cm, including the normal skin near the wound. Then, the EF values (Z-axis values) at each point in the wound margin and wound surface (X and Y were the distance from the origin) were measured. In this way, 3D schematic diagrams of wound EFs were drawn to better describe the wound EFs and changes in the wound EFs after the application of exogenous EFs. The EFs of the blank control group in the area of normal skin and at the center of the wound were very low (Fig. 5A, 3.36 ± 2.32 mV mm−1 and 9.69 ± 2.22 mV mm−1, respectively) and the highest EF was at the wound margin (Fig. 5A, 81.19 ± 3.62 mV mm−1). Similarly, in the AgNWs-PU foam group, the EFs at the three points above were 2.45 ± 1.42 mV mm−1, 11.29 ± 3.33 mV mm−1, and 83.53 ± 6.84 mV mm−1 respectively (Fig. 5A). Correspondingly, for the AgNWs-PU/EF foam group (Fig. 5A), the EFs of the normal skin and wound center were 5.26 ± 3.96 mV mm−1 and 14.17 ± 3.42 mV mm−1, respectively, while the highest EF of 187.14 ± 10.69 mV mm−1 was recorded at the wound margin after the application of exogenous EFs. Compared with the EFs of blank control group, the EFs at the wound margin were increased to nearly 100 mV mm−1 (Fig. 5A). The endogenous wound EFs gradually decreased with increasing distance from the margin of the wound. When the pulse power was 6 V with a 70% duty cycle, use of the AgNWs-PU foam to apply exogenous EFs at a strength of 100 mV mm−1 superimposed on the endogenous wound EFs maintained the total EF strength at approximately 200 mV mm−1, which proved that the exogenous EFs had been successfully applied (Fig. 5B). So that the exogenous EFs could optimize wound microenvironment by providing stronger wound electrical signals. At 8 h after wounding, the wound margin EFs were detected again. Real-time EF values of the wounds were detected at five other time points: on the 2nd, 3rd, 4th, 7th, and 14th day after injury. On the 7th day, the endogenous wound EFs without an exogenous EFs in the AgNWs-PU/EF group were 37.86 mV mm−1 (Fig. 5B), lower than that in the AgNWs-PU group (42.26 mV mm−1) and blank group (61.48 mV mm−1) (Fig. 5C). Similarly, on the 14th day, the endogenous wound EFs without exogenous EFs in the AgNWs-PU/EF group were18.69 mV mm−1 (Fig. 5B), lower than that in the AgNWs-PU group (27.20 mV mm−1) and blank group (36.88 mV mm−1) (Fig. 5C). However, after exogenous EFs were applied, the total EF strength in the AgNWs-PU/EF foam group were always higher than those in the AgNWs-PU foam group and blank control group at all time points (Fig. 5C).
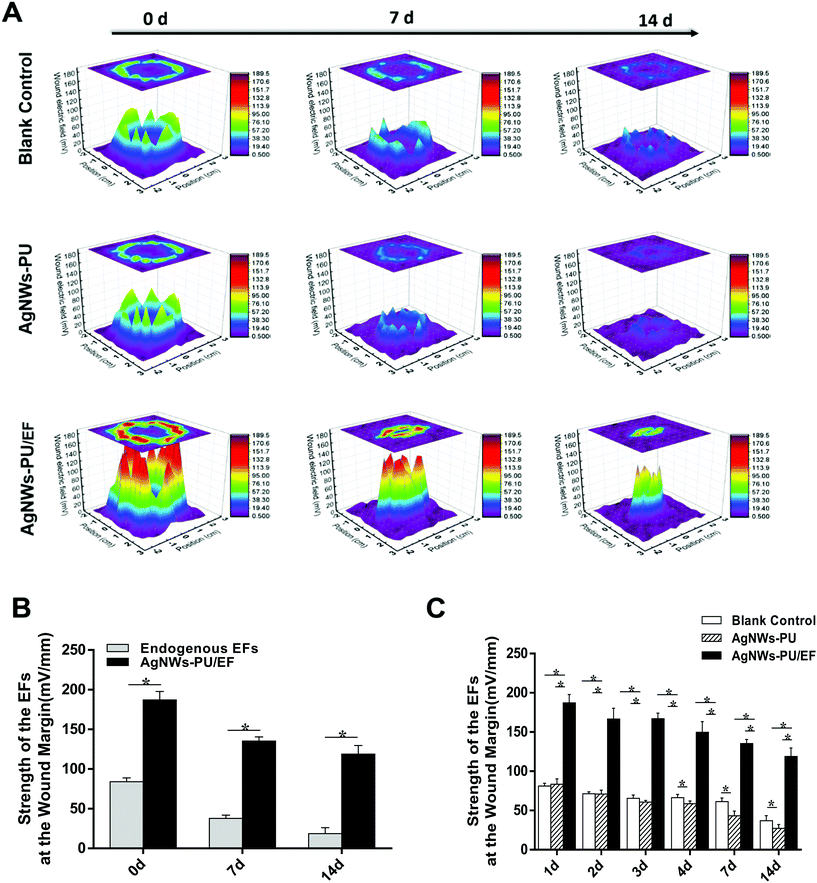 |
| Fig. 5 Summary of the effect of electric fields on pig wounds. (A) Electrical fields of the wounds and normal nearby skin were detected to draw voltage profiles over the wounds. The three-dimensional profile of the surface potential indicates that the numerical value was spatially regulated. (B) The strength of wound EFs changed before (endogenous EFs) and after the application of exogenous EFs. (C) The wound EF strength on the 1st, 2nd, 3rd, 4th, 7th and14th days. *P < 0.05. | |
The transepithelial potential of the normal epidermis is the basis of wound EF formation. Due to the directional transport of ion pumps, cations (Na+) are transported to the epidermal basal side and anions (Cl−) are transported to the epidermis, creating transepithelial potential.46 When a wound occurs, a current is driven out of the injury, and the flow of this current generates endogenous EFs.47 As the process of wound healing continues, more and more keratinocytes migrate from the wound margin to the wound center. When the keratinocytes have migrated to the appropriate locations, they begin to proliferate and differentiate, resulting in the structural rearrangement and layers of keratinocytes become thicker. Some studies have reported that due to the thickening of the keratinocytes layer and increased organization of the cell arrangement, which increase the transepidermal resistance to current flow, endogenous wound EFs gradually decrease.48–50 Attenuation of the endogenous EFs was closely related to healing time and speed, which provide an indication of the state of wound healing.51
3.4. Effect on cell proliferation
First, wound healing requires sufficient epidermal cells to cover the wound. Negative pressure on the wound surface produces mechanical traction, inducing the proliferation of epidermal cells, fibroblasts, and vascular endothelial cells.15,17 When cells are stimulated with growth factor, the PI3K/Akt pathway mediates primary signaling to induce cell proliferation. The result of western blot analysis on the 7th day showed that the expression of PI3K and Akt remained stable, while phosphorylated PI3K and phosphorylated Akt were upregulated in the tissues subjected to exogenous wound EFs (Fig. S4†). This finding indicated that exogenous wound EFs promoted cell proliferation by triggering the PI3K/Akt pathway, which was consistent with previous in vitro studies.20,21 Ki67 is a cell proliferation-related antigen in the nucleus and a highly specific cell marker of proliferation. We used immunohistochemical staining to detect positive Ki67 staining at different time points in the different groups as an indicator of the cell proliferation status. As shown in Fig. 6A and B, the AgNWs-PU/EF foam group showed the highest positive staining result on the 7th day, which was 1.23 times that in the AgNWs-PU foam group and 2.03 times that in the blank group. Positive staining for Ki67 on the 14th day was 142% higher in the AgNWs-PU/EF foam group than in the blank group, while that of the AgNWs-PU foam group was 85% higher than that of the blank group.
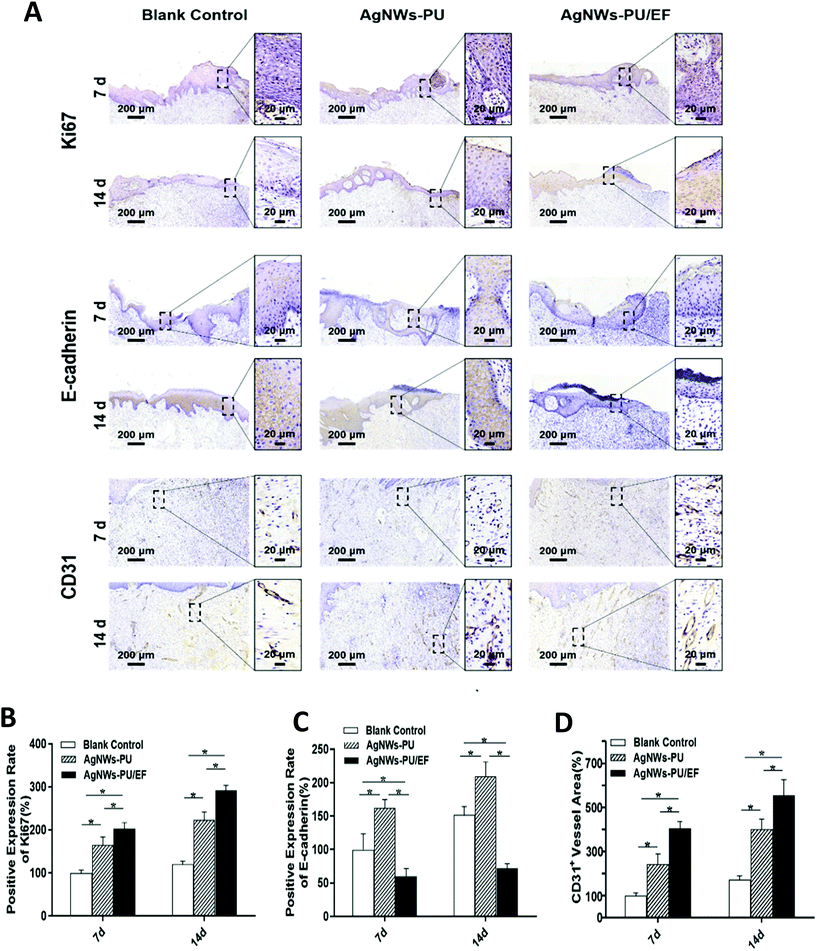 |
| Fig. 6
In vivo assessments of the different sample treatments on proliferation, migration, and angiogenesis. (A) Immunohistochemical staining to determine Ki67, E-cadherin, and CD31 positivity in the wound tissue on the 7th and 14th days after wounding in the blank control, AgNWs-PU, and AgNWs-PU/EF groups. Images were analyzed to quantify positive Ki67 (B), E-cadherin (C), and CD31 (D) expression. *P < 0.05. | |
3.5. Effect on cell migration
Epidermal migration is the rate-limiting step of re-epithelialization. E-cadherin, a calcium ion-dependent cell adhesion glycoprotein that is mainly distributed in epithelial tissues, mediates cell adhesion and connection. Downregulated E-cadherin expression can weaken the adhesion between cells, accelerating the migration of epidermal cells. On the 7th and 14th days, the expression level of E-cadherin in the AgNWs-PU/EF foam group was lower than that in the AgNWs-PU foam group and blank control group. The expression level of E-cadherin in the AgNWs-PU foam group was the highest among all groups (Fig. 6A and C). These results indicate that during wound healing, enhanced wound EFs can downregulate the expression of E-cadherin to weaken intercellular adhesion, promoting cell migration.
Re-epithelialization begins with the migration of keratinocytes from the wound margin, followed by proliferation to cover the wound. When keratinocytes migrate to the right location, they proliferate, differentiate, and rearrange, strengthening intercellular adhesion.1 In early re-epithelialization, wound EFs enhance keratinocyte motility and direct migration, and after sufficient keratinocytes migrate to the wound center, exogenous EFs are physiologically attenuated, resulting in differentiated, rearranged, and compact keratinocytes. At that time, E-cadherin expression is increased to enhance intercellular adhesion.52 Compared with that in the blank control group, the degree of wound healing in AgNWs-PU group was higher due to the NPWT (Fig. 7). Furthermore, keratinocyte proliferation in the AgNWs-PU group was higher than that in the blank control group after keratinocytes migrated to the wound (Fig. 6B). As more and more keratinocytes gathered on the wound center, enhanced intercellular interaction caused the increased expression of E-cadherin and structural remodeling.
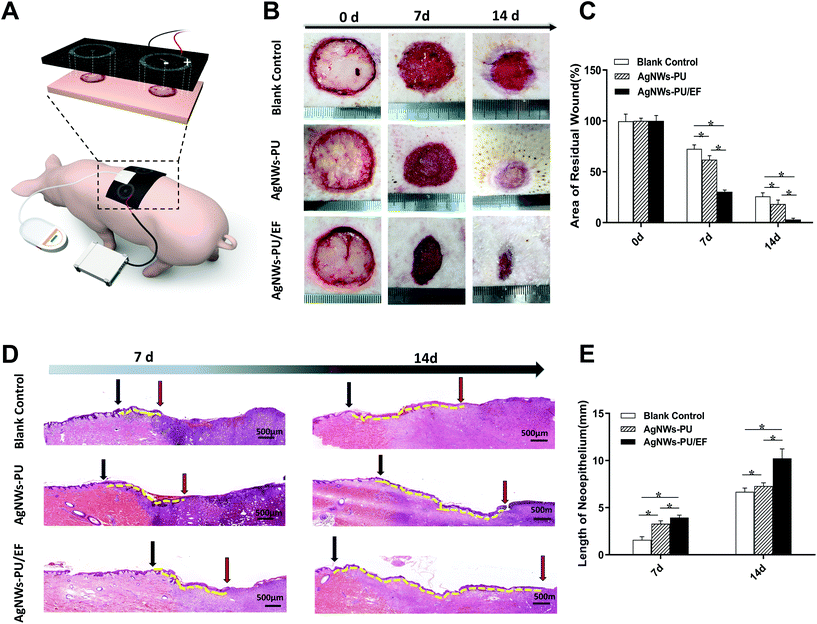 |
| Fig. 7 Therapeutic efficacies of the different treatments on wound healing in a pig model. (A) AgNWs-PU foam and ordinary PU foam dressings were assembled according to image A, with one wound connected to the power supply used to apply exogenous EFs. The pig wound was connected to a negative-pressure device. (B) Residual wound area in each group. (C) Statistical analysis of the residual wound percentage. (D) H&E staining to measure the length of the new epithelium. The yellow curve indicates the new epidermis length. (E) Statistical analysis the length of the new epithelium. *P < 0.05. | |
3.6. Effect on neovascularization
Angiogenesis is crucial for wound healing because wounds require an adequate blood supply for nutrition. Previous studies have shown that NPWT can promote VEGF secretion, thus inducing angiogenesis. Wound EFs can also stimulate endothelial cells to secrete VEGF. After exogenous EFs were applied for 5 min, the VEGF concentration was observed to increase in vitro cell experiments.53 Our results showed that the expression of VEGF was significantly upregulated in the tissues upon exogenous EF application, which verified the function of exogenous EFs in inducible VEGF secretion (Fig. S4†). CD31, a marker of vascular endothelial differentiation, is distributed in vascular endothelial cells. The expression of CD31 can be used as an indicator of the degree of vascularization. In this study, wound tissues were assessed by immunohistochemical staining for CD31 on the 7th and 14th days. As shown in Fig. 6A, positively stained blood vessels were observed in the wounds in all groups. However, compared to the blank control group, the AgNWs-PU/EF group exhibited 1.67 times vascular density on the 7th day and 1.37 times vascular density on the 14th days, while that of the AgNWs-PU foam group was 4.06 times on the 7th day and 3.21 times on the 14th day compared to vascular density in the blank control group on the corresponding days (Fig. 6D). Our results demonstrated that this combined therapy could optimize the wound microenvironment by inducing VEGF secretion to promote wound neovascularization. Moreover, the application of exogenous EFs with NPWT to facilitate wound angiogenesis exhibited a greater effect than the application of NPWT alone.
3.7. Effect on inflammation inhibition
At the inflammation stage, NWPT is advantageous for draining wound exudate and pus, and wound EFs can reduce the release of inflammatory factors.54 Inflammatory cells in the H&E staining images taken on the 7th day of wound formation were counted to assess the inflammatory state of the wound (Fig. S5†). Fewer inflammatory cells were observed in the AgNWs-PU/EF group (27 ± 4 cells) than in the AgNWs-PU group (61 ± 6 cells) and blank group (152 ± 8 cells), indicating that wound inflammation had been effectively inhibited by the combined treatment to optimize the wound microenvironment.
3.8. Effect on wound healing in a pig model
The residual wound area is the most intuitive indicator of wound healing. On the 7th and 14th days after surgery, there was a statistically significant difference in the percentage of residual wound area among the groups. The residual wound area percentage in each group (residual area/original area of the wound × 100%) was calculated on the 7th day. Fig. 7B and C show that the AgNWs-PU/EF foam group had the lowest residual wound area percentage (30.30 ± 1.75%), followed by the AgNWs-PU foam group (61.94 ± 3.86%), while the blank group had the largest residual wound area percentage (72.54 ± 3.89%). On the 14th day, this difference was even greater. The percentage of the residual wound area in the AgNWs-PU/EF foam group was only 3.07 ± 1.23%, that in the AgNWs-PU foam group was 18.35 ± 3.83%, and that in the blank group was 25.82 ± 3.52%.
The new epidermis length of a wound is the most intuitive indicator of the degree of the re-epithelialization. Concurrent with the above analysis, we observed new epidermis by H&E staining. As shown in Fig. 7D and E, on the 7th day, the new epidermis length was 3.94 ± 0.26 mm in the AgNWs-PU/EF foam group, 3.29 ± 0.32 mm in the AgNWs-PU foam group, and 1.59 ± 0.34 mm in the blank group, and these differences were significant (P < 0.05). On the 14th day, the difference in new epidermis length was greater. The length in the AgNWs-PU/EF foam group was 10.23 ± 1.01 mm, that in the AgNWs-PU group was 7.29 ± 0.34 mm, and that in the blank group was 6.68 ± 0.41 mm, and these differences were significant (P < 0.05). In addition, morphological extension of new epithelium in the AgNWs-PU/EF foam group showed better directionality towards the center of the wounds with a more ordered cell distribution.
4. Conclusion
This study focused on constructing a 3D foam dressing with the ability to facilitate both conduction and drainage by combining AgNWs with an ultra-high length–diameter ratio with PU foam such that negative pressure and exogenous wound EFs simultaneously promoted wound healing. This combined therapeutic system showed enhanced effects on necrotic tissue drainage, cell proliferation and migration, and angiogenesis by optimizing the wound microenvironment, thus facilitating wound healing at multiple stages. Supported by additional in vivo experiments in Bama pigs, the results of our study not only demonstrated the innovation of existing wound treatment methods but also provide a basis for follow-up clinical research.
Conflicts of interest
The authors declare no conflict of interests.
Acknowledgements
This work was supported in part by the National Natural Science Foundation of China (81873936), the National Key Research and Development Plan (2016YFC1101100), the Chongqing Natural Science Foundation (cstc2019jscx-msxmX0101), and the Clinical Technology Innovation and Cultivation Project of Army Medical University (CX2019JS102).
References
- G. C. Gurtner, S. Werner, Y. Barrandon and M. T. Longaker, Nature, 2008, 453, 314–321 CrossRef CAS.
- S. A. Eming, P. Martin and M. Tomic-Canic, Sci. Transl. Med., 2014, 6, 265sr266 Search PubMed.
- P. Heher, S. Muhleder, R. Mittermayr, H. Redl and P. Slezak, Adv. Drug Delivery Rev., 2018, 129, 134–147 CrossRef CAS.
- P. Martin, Science, 1997, 276, 75–81 CrossRef CAS.
- I. Mataro, G. Delli Santi, P. Palombo, R. D'Alessio and M. Vestita, Ann. Burns Fire Disasters, 2017, 30, 313–316 CAS.
- C. Waycaster and C. T. Milne, J. Med. Econ., 2013, 16, 976–986 CrossRef.
- H. Xu, Z. Fang, W. Tian, Y. Wang, Q. Ye, L. Zhang and J. Cai, Adv. Mater., 2018, 11, 1801100 CrossRef.
- S. Hou, Y. Liu, F. Feng, J. Zhou, X. Feng and Y. Fan, Adv. Healthcare Mater., 2020, 9, 1901041 CrossRef CAS.
- M. B. Dreifke, A. A. Jayasuriya and A. C. Jayasuriya, Mater. Sci. Eng., C, 2015, 48, 651–662 CrossRef CAS.
- J. Zhou, M. Ni, X. Liu, Z. Ren and Z. Zheng, Med. Sci. Monit., 2017, 23, 555–562 CrossRef CAS.
- Y. Chen, Y. Wu, J. Gao, Z. Zhang, L. Wang, X. Chen, J. Mi, Y. Yao, D. Guan, B. Chen and J. Dai, ACS Appl. Mater. Interfaces, 2017, 9, 5173–5180 CrossRef CAS.
- A. S. MacLeod and J. N. Mansbridge, Adv. Wound Care, 2016, 5, 65–78 CrossRef.
- M. J. Carter, Appl. Health Econ. Health Policy, 2014, 12, 373–389 CrossRef.
- Z. Deng, F. Shi, Z. Zhou, F. Sun, M. H. Sun, Q. Sun, L. Chen, D. Li, C. Y. Jiang, R. Z. Zhao, D. Cui, X. J. Wang, Y. F. Jing, S. J. Xia and B. M. Han, Toxicol. Appl. Pharmacol., 2019, 366, 83–95 CrossRef CAS.
- P. Agarwal, R. Kukrele and D. Sharma, J. Clin. Orthop. Trauma, 2019, 10, 845–848 CrossRef.
- S. Maurya and P. S. Bhandari, Adv. Wound Care, 2016, 5, 379–389 CrossRef.
- I. Sahin, M. Eski, C. Acikel, R. Kapaj, D. Alhan and S. Isik, Ann. Burns Fire Disasters, 2012, 25, 92–97 CAS.
- K. J. Simpson, L. M. Selfors, J. Bui, A. Reynolds, D. Leake, A. Khvorova and J. S. Brugge, Nat. Cell Biol., 2008, 10, 1027–1038 CrossRef CAS.
- C. D. McCaig, A. M. Rajnicek, B. Song and M. Zhao, Physiol. Rev., 2005, 85, 943–978 CrossRef.
- M. Zhao, Semin. Cell Dev. Biol., 2009, 20, 674–682 CrossRef CAS.
- M. Zhao, B. Song, J. Pu, T. Wada, B. Reid, G. Tai, F. Wang, A. Guo, P. Walczysko, Y. Gu, T. Sasaki, A. Suzuki, J. V. Forrester, H. R. Bourne, P. N. Devreotes, C. D. McCaig and J. M. Penninger, Nature, 2006, 442, 457–460 CrossRef CAS.
- Y. Shen, T. Pfluger, F. Ferreira, J. Liang, M. F. Navedo, Q. Zeng, B. Reid and M. Zhao, Sci. Rep., 2016, 6, 26525 CrossRef CAS.
- C. Quijada, Materials, 2020, 13, 2344 CrossRef CAS.
- A. Capezza, R. L. Andersson, V. Strom, Q. Wu, B. Sacchi, S. Farris, M. S. Hedenqvist and R. T. Olsson, ACS Omega, 2019, 4, 3458–3468 CrossRef CAS.
- H. Zhang, X. Sun, M. A. Hubbe and L. Pal, Carbohydr. Polym., 2019, 222, 115013 CrossRef CAS.
- H. B. Yao, J. Ge, C. F. Wang, X. Wang, W. Hu, Z. J. Zheng, Y. Ni and S. H. Yu, Adv. Mater., 2013, 25, 6692–6698 CrossRef CAS.
- Q. Meng, Y. Yu, J. Tian, Z. Yang, S. Guo, R. Cai, S. Han, T. Liu and J. Ma, Nanotechnology, 2020, 31, 465502 CrossRef CAS.
- P. Lee, J. Lee, H. Lee, J. Yeo, S. Hong, K. H. Nam, D. Lee, S. S. Lee and S. H. Ko, Adv. Mater., 2012, 24, 3326–3332 CrossRef CAS.
- Z. Chen, W. Ren, L. Gao, B. Liu, S. Pei and H. M. Cheng, Nat. Mater., 2011, 10, 424–428 CrossRef CAS.
- J. Park, S. Wang, M. Li, C. Ahn, J. K. Hyun, D. S. Kim, D. K. Kim, J. A. Rogers, Y. Huang and S. Jeon, Nat. Commun., 2012, 3, 916 CrossRef.
- G. S. Jeong, D. H. Baek, H. C. Jung, J. H. Song, J. H. Moon, S. W. Hong, I. Y. Kim and S. H. Lee, Nat. Commun., 2012, 3, 977 CrossRef.
- C. Yang, H. Gu, W. Lin, M. M. Yuen, C. P. Wong, M. Xiong and B. Gao, Adv. Mater., 2011, 23, 3052–3056 CrossRef CAS.
- B. Y. Ahn, E. B. Duoss, M. J. Motala, X. Guo, S. I. Park, Y. Xiong, J. Yoon, R. G. Nuzzo, J. A. Rogers and J. A. Lewis, Science, 2009, 323, 1590–1593 CrossRef CAS.
- S. Zhang, H. Liu, S. Yang, X. Shi, D. Zhang, C. Shan, L. Mi, C. Liu, C. Shen and Z. Guo, ACS Appl. Mater. Interfaces, 2019, 11, 10922–10932 CrossRef CAS.
- L. Xu, R. Bai, X. Cheng, A. Shao, L. Chen, S. Qu and C. Chen, J. Biomed. Nanotechnol., 2018, 14, 564–574 CrossRef CAS.
- L. Hu, H. S. Kim, J. Y. Lee, P. Peumans and Y. Cui, ACS Nano, 2010, 4, 2955–2963 CrossRef CAS.
- E. C. Garnett, W. Cai, J. J. Cha, F. Mahmood, S. T. Connor, M. Greyson
Christoforo, Y. Cui, M. D. McGehee and M. L. Brongersma, Nat. Mater., 2012, 11, 241–249 CrossRef CAS.
- W. Xiong, H. Liu, Y. Chen, M. Zheng, Y. Zhao, X. Kong, Y. Wang, X. Zhang, X. Kong, P. Wang and L. Jiang, Adv. Mater., 2016, 28, 7167–7172 CrossRef CAS.
- M. Lagrange, D. P. Langley, G. Giusti, C. Jimenez, Y. Brechet and D. Bellet, Nanoscale, 2015, 7, 17410–17423 RSC.
- Y. Ge, X. Duan, M. Zhang, L. Mei, J. Hu, W. Hu and X. Duan, J. Am. Chem. Soc., 2018, 140, 193–199 CrossRef CAS.
- Y. Lu, Y. Wu, J. Liang, M. R. Libera and S. A. Sukhishvili, Biomaterials, 2015, 45, 64–71 CrossRef CAS.
-
T. Sandle, U.S. Pharmacopeial Convention, 2014 Search PubMed.
- A. R. Gliga, S. Skoglund, I. O. Wallinder, B. Fadeel and H. L. Karlsson, Part. Fibre Toxicol., 2014, 11, 11 CrossRef.
- P. M. Favi, M. M. Valencia, P. R. Elliott, A. Restrepo, M. Gao, H. Huang, J. J. Pavon and T. J. Webster, J. Biomed. Mater. Res., Part A, 2015, 103, 3940–3955 CrossRef CAS.
- E. J. Park, J. Yi, Y. Kim, K. Choi and K. Park, Toxicol. in Vitro, 2010, 24, 872–878 CrossRef CAS.
- R. Nuccitelli, Curr. Top. Dev. Biol., 2003, 58, 1–26 CrossRef.
- I. S. Foulds and A. T. Barker, Br. J. Dermatol., 1983, 109, 515–522 CrossRef CAS.
- L. F. Jaffe and R. Nuccitelli, J. Cell Biol., 1974, 63, 614–628 CrossRef CAS.
- D. D. Sta Iglesia, E. J. Cragoe Jr. and J. W. Vanable Jr., J. Exp. Zool., 1996, 274, 56–62 CrossRef CAS.
- R. Kucerova, P. Walczysko, B. Reid, J. Ou, L. J. Leiper, A. M. Rajnicek, C. D. McCaig, M. Zhao and J. M. Collinson, J. Cell Physiol., 2011, 226, 1544–1553 CrossRef CAS.
- R. Nuccitelli, P. Nuccitelli, C. Li, S. Narsing, D. M. Pariser and K. Lui, Wound Repair Regener., 2011, 19, 645–655 CrossRef.
- K. Campbell and J. Casanova, Development, 2016, 143, 4291–4300 CrossRef CAS.
- M. Zhao, H. Bai, E. Wang, J. V. Forrester and C. D. McCaig, J. Cell Sci., 2004, 117, 397–405 CrossRef CAS.
- S. G. Gurgen, O. Sayin, F. Cetin and A. Tuc Yucel, Inflammation, 2014, 37, 775–784 CrossRef CAS.
Footnotes |
† Electronic supplementary information (ESI) available. See DOI: 10.1039/d0bm01172j |
‡ These authors contribute equally to this work. |
|
This journal is © The Royal Society of Chemistry 2021 |
Click here to see how this site uses Cookies. View our privacy policy here.