DOI:
10.1039/D0CE01527J
(Paper)
CrystEngComm, 2021,
23, 69-81
2D MOF-derived porous NiCoSe nanosheet arrays on Ni foam for overall water splitting†
Received
18th October 2020
, Accepted 9th November 2020
First published on 9th November 2020
Abstract
The exploitation of low-cost, efficient and stable electrocatalysts for overall water splitting in alkaline media is critical for future renewable energy systems, yet still remains a great challenge. Herein, we report the in situ growth of porous NiCoSe nanosheet arrays derived from 2D MOFs on Ni foam through a simple ion-exchange reaction followed by a hydrothermal process. Benefiting from the unique 2D multi-porous framework with high electrochemical active surface area, conductivity and open channels for the release of gaseous products, the as-prepared NiCoSe nanosheet arrays exhibit excellent electrochemical activity with a low overpotential of 170 mV for the hydrogen evolution reaction (HER) at 10 mA cm−2 and 278 mV for the oxygen evolution reaction (OER) at 20 mA cm−2 and long-term stability. Furthermore, the full cell only requires 1.51 V to drive the current density of 10 mA cm−2 when the NiCoSe nanosheet arrays are used as both anode and cathode, which also exhibits distinguished durability for over 48 h. The present work presents a general strategy for the rational design and synthesis of multifunctional 2D porous electrocatalysts, which is also expected to extend to other noble-metal-free catalysts.
1. Introduction
The ever-increasing energy crisis and intensified environmental pollution has caused many researchers to develop clean and sustainable carbon-free green energy sources, such as solar, wind and water.1–4 Hydrogen energy has long been identified as a promising clean and sustainable energy source alternative to fossil fuels due to its abundance, renewability, high energy density, pollution-free nature and transportability.5–8 Recently, electrochemical water splitting has emerged as the most attractive strategy for producing hydrogen and oxygen from aqueous solutions.9–11 Generally, this process is governed by two half-reactions, namely hydrogen evolution reaction (HER) and oxygen evolution reaction (OER), taking place at the cathode and the anode, respectively.12,13 However, the kinetics of the OER is sluggish because of its intrinsically complex four-electron process, the high activation energy barrier during O–O bond formation and the complicated adsorption behavior of the intermediates on the electrode in comparison to the HER, which seriously impedes the overall efficiency of water splitting.14–17 Nowadays, Pt and IrO2 (or RuO2) have been proven as the state-of-the-art electrocatalysts for the HER and OER, respectively; however, their high cost, scarcity and poor durability greatly hinder their large-scale applications.18–20 Hence, tremendous efforts have been devoted to develop low-cost, highly efficient and abundant transition metal-based compounds (oxides, sulfides, selenides, phosphides, hydroxides etc.) as promising HER or OER electrocatalysts.21–25 For practical applications, it would be highly attractive to use bifunctional electrocatalysts in the same electrolyte for both the HER and OER simultaneously.26–28 Among these transitional metal catalysts, nickel- and cobalt-based selenides have sparked great interest due to their high intrinsic catalytic activities, abundant storage in nature, long-term stability and low cost.29–32 In particular, bimetallic nickel cobalt selenides exhibit improved electrochemical activity than monometallic nickel or cobalt selenides,33,34 which is commonly attributed to the following factors: (1) the outstanding exposure of active sites and defects,35 (2) remarkable conductivity36 and (3) open channels for the effective release of reaction products.37 Hence, in situ growth of porous bimetallic nickel cobalt selenide nanosheet arrays on Ni foam (NF) should be a good strategy because the nanosheet arrays would prevent the aggregation of nanosheets and provide more active sites effectively.38,39 The unique porous structure is beneficial for the penetration of the electrolyte and the expedient release of the generated hydrogen/oxygen gas bubbles.40,41 Moreover, the Ni foam can be used directly as the electrode because of its excellent electrical conductivity and the synergistic effects with the electrocatalysts.42
Metal–organic frameworks (MOFs) with a large surface area and tunable structure are constructed by the coordination of metal ions and organic ligands, which have been applied in the field of energy storage, molecular separation, sensing, drug delivery and photoelectrocatalysis.43–47 Although lots of 3D MOF-based electrocatalysts such as porous Cu3P@C,48 cobalt nanoparticles@NPC,49 carbon-incorporated porous honeycomb NiCoFe phosphide nanospheres50etc. have been developed, to the best of our knowledge, there is no report on 2D MOF-derived bimetallic nickel cobalt selenide electrocatalysts. Herein, we report a simple method to synthesize porous NiCoSe nanosheet arrays on Ni foam based on 2D ZIF-67 as highly efficient electrocatalysts for the HER, OER and overall water splitting. The fabrication route can be seen in Scheme 1.
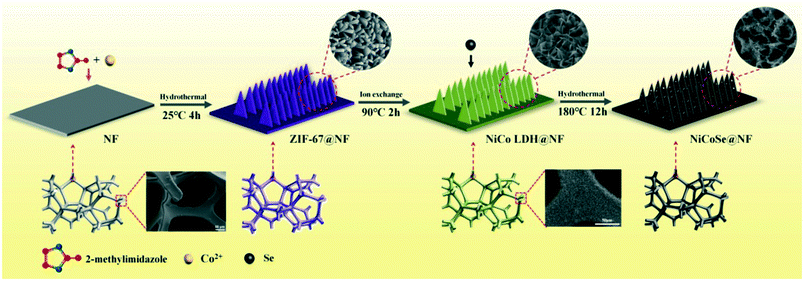 |
| Scheme 1 Schematic illustration of the synthesis of NiCoSe electrocatalysts. | |
2. Experimental section
2.1 Materials
Cobalt nitrate (Co(NO3)2·6H2O), 2-methylimidazole (C4H6N2), selenium powder, sodium hydroxide, hydrazine hydrate (N2H4·H2O), hydrochloric acid (HCl), acetone (CH3COCH3) and anhydrous alcohol (C2H6O) are all analytical grade (Aladdin) and used without further purification.
2.2 Treatment of the Ni foam
A piece of Ni foam (20 mm × 40 mm) was washed with 3.0 M HCl, acetone and deionized water several times to remove surface impurities and dried at 60 °C for 6 h in vacuum.
2.3 Synthesis of ZIF-67@NF
0.582 g of Co(NO3)2·6H2O (solution A) and 1.314 g of 2-methylimidazole (solution B) were dispersed in 40 ml of deionized water, respectively, followed by stirring for 10 min to dissolve them completely. Then, solution B was rapidly poured into solution A after stirring for 1 h (solution C). The cleaned Ni foam obtained above (20 mm × 40 mm) was immersed in solution C for 4 h at room temperature. Finally, the purple ZIF-67@NF was obtained, which was washed with deionized water and alcohol three times and dried at 60 °C for 6 h in vacuum. The calculated average mass loading was found to be ∼4.2 mg cm−2.
2.4 Synthesis of NiCo LDH@NF
The ZIF-67@NF was put into 100 ml of deionized water and ethanol (1
:
9 in volume ratio), which was subsequently kept at 90 °C for 2 h. After cooling to room temperature, the obtained light green NiCo LDH@NF was taken out, washed with deionized water and anhydrous alcohol several times and then dried at 60 °C for 6 h in vacuum. The average mass loading was calculated to be ∼1.6 mg cm−2.
2.5 Synthesis of NiCoSe@NF
In a typical process, x mmol of Se powder (x = 1, 2, 4, 6, 8, 10) and 16 mmol of NaOH were dissolved in a mixture of 5 ml of hydrazine hydrate and 10 ml of deionized water. The solution was then added into a 20 ml Teflon-lined stainless steel autoclave after stirring for 3 h. The NiCo LDH@NF was then immersed in the autoclave, which was sealed and heated at 180 °C for 12 h in an oven. After the experiment, the autoclave was cooled to room temperature. The resulting black NiCoSe@NF was washed several times with ethanol and deionized water and then dried at 60 °C for 6 h in vacuum. The average mass loading was calculated to be ∼4.7 mg cm−2. For comparison, we also treated Ni foam with 8 mmol Se powder by the same approach.
2.6 Material characterization
X-ray diffraction (XRD) patterns were obtained on a MAC Science MXP-18 X-ray diffractometer utilizing a Cu target radiation source. Transmission electron micrograph (TEM) and high-resolution transmission electron micrograph (HRTEM) images were acquired on a JEM-2100 electron microscope with an accelerating voltage of 200 kV. Field emission scanning electron microscope (FESEM) images, elemental mappings and energy dispersive X-ray spectroscopy (EDAX) images were obtained on a JEOL JSM-7800F instrument at 10.0 kV. X-ray photoelectron characterization was recorded on a Vg Escalab MK II Xray photoelectron spectrometer (XPS) using Mg Kα radiation (hν = 1253.6 eV) with a resolution of 1.0 eV. Taking the C 1s peak value of 284.60 eV as the standard, the binding energy value of the obtained sample was corrected.
2.7 Electrochemical characterization
HER/OER electrochemical performance tests were performed on a CHI 760E electrochemical workstation (Chenhua Corp., Shanghai) in a standard three-electrode system at ambient temperature, of which a graphite rod was used as the counter electrode, an Hg/HgO electrode as the reference electrode and the NiCoSe@NF sample (cut into pieces of 0.3 × 0.3 cm2) as the working electrode. In the two-electrode cell system, the as-prepared NiCoSe@NF served as both cathode and anode for overall water splitting. The electrolyte was 1.0 M KOH (pH 13.6) for all the electrochemical tests. Before the electrochemical experiments, the electrolyte was previously degassed with N2 for 30 min. All the potentials were converted to potentials versus the reversible hydrogen electrode (RHE) by using the following equation:
ERHE = ESCE + 0.098 + 0.059 × PH |
Linear sweep voltammetry (LSV) curves were tested at a scan rate of 1 mV s−1 to obtain the polarization curves, which attained steady state after several cycles. All measured polarization curves were iR-corrected. For comparison, commercial Pt/C or IrO2 was also prepared as the working electrode. In a typical process, 20 mg of commercial Pt/C (20 wt%) (or IrO2) powder was dispersed in a mixture of 60 μl of Nafion solution (1 wt%) and 540 μl of isopropanol solution, which was sonicated for 30 minutes. Then, 8 μl of the above solution was dropped on a piece of cleaned Ni foam (0.3 cm × 0.3 cm, catalyst loading ≈3 mg cm−2). The cycle durability was measured by the chronoamperometric response. Electrochemical impedance spectroscopy (EIS) measurements were carried out at a frequency ranging from 0.1 to 104 Hz.
3. Results and discussion
Fig. 1a shows the porous structure of the Ni foam. The contact angle test of the Ni foam can be seen in the inset in Fig. 1a. The Ni foam shows a hydrophobic surface (contact angle 93°). Fig. 1b shows the XRD pattern of ZIF-67. It can be found that all of the diffraction peaks are in line with previous reports.51 From the SEM image in Fig. 1c, it is clearly shown that numerous smooth nanosheets with a thickness of 160 nm are vertically arrayed on the surface of the Ni foam. As shown in the optical image (Fig. S1†), the color of the Ni foam transformed from silvery to purple, indicating the successful deposition of ZIF-67 on the surface of the Ni foam. Then the ZIF-67 precursor was put into the ethanol aqueous solution, which would produce both H+ and OH− ions through hydrolysis. On the one hand, the H+ ions induced the etching of ZIF-67 and produced the Co2+ ions; on the other hand, the OH− ions promoted the precipitation of Ni2+ and Co2+ ions to form the NiCo layered double hydroxide (NiCo LDH). As the hydrolysis reaction went on, the ZIF-67 was fully etched and porous nanosheet arrays with a thickness of 330 nm were formed (Fig. 1d–f). Compared with the original ZIF-67 nanosheets, the NiCo LDH nanosheets became rough and superhydrophilic (inset in Fig. 1d), which could improve the wettability of electrocatalysts.39,52,53 The energy dispersive spectrum (EDS) mappings (Fig. 1g–i) recorded from Fig. 1f indicate that Co, Ni and O elements are distributed in all of the NiCo LDH nanosheets uniformly. This also confirms that the content of the cobalt ions is higher than that of the nickel ions (coming from the Ni foam). All these observations suggest that the NiCo LDH nanosheets are successfully synthesized.
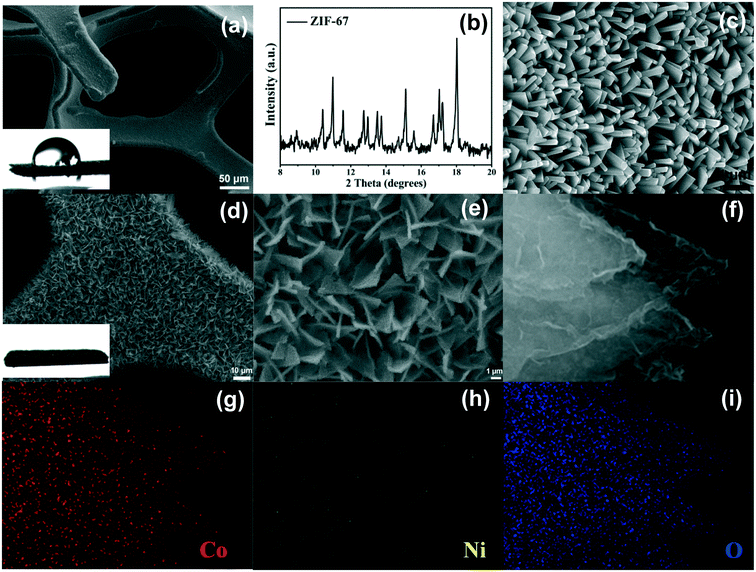 |
| Fig. 1 (a) SEM image of Ni foam; (b) XRD pattern and (c) SEM image of ZIF-67; (d and e) SEM images of NiCo LDH on Ni foam; (f) FESEM image of NiCo LDH and the corresponding elemental mappings of (g) Co, (h) Ni and (i) O elements for NiCo LDH. | |
The detailed internal microstructure of the NiCo LDH nanosheets was further examined by TEM. As can be seen in Fig. 2a, all of the nanosheets of ZIF-67 are etched into the nearly transparent porous NiCo LDH nanosheets with a rambling edge. The HRTEM image shows well-resolved lattice fringes with lattice distances of 0.26 nm, corresponding to the (012) plane of NiCo LDH54,55 (Fig. 2b).
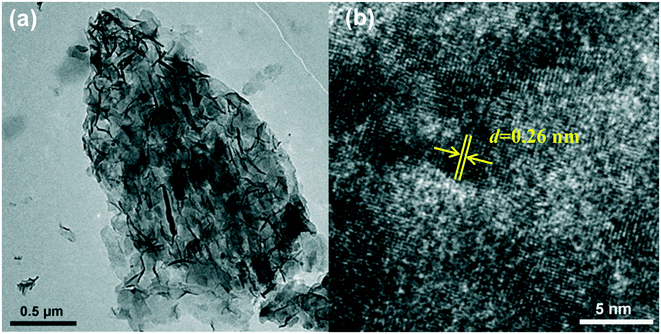 |
| Fig. 2 (a) and (b) TEM and HRTEM images of NiCo LDH. | |
Subsequently, the NiCo LDH nanosheets were treated with Se powder at 180 °C in the autoclave. The surface color of the Ni foam became black (Fig. S1†). During this selenization process, the OH− ions in the solution reacted with selenium to form the Se2− ions. The formed Se2− ions would react quickly with the Ni2+/Co2+ ions of NiCo LDH via the Se2−/OH− exchange reaction to construct the NiCoSe nanosheets. As shown in Fig. 3a, the characteristic peaks of NiCoSe match well with those of NiSe (JCPDS No. 02-0892) and CoSe (JCPDS No. 89-2004). Both NiSe and CoSe belong to the hexagonal crystal system with the space group P63/mmc, and the atomic radii of Ni and Co are close.56 Therefore, the NiSe and CoSe samples show similar diffraction patterns. Except for three diffraction peaks at 2θ = 44.31°, 49.81° and 76.26° arising from the Ni foam, no diffraction peak of NiCo hydroxides or oxides is found in the XRD spectra, which further confirms that NiCo LDH has been converted to NiCoSe successfully. For comparison, we also treated the Ni foam with Se powder by the same approach. The results manifest the growth of NiSe only (Fig. 3a, brown line).
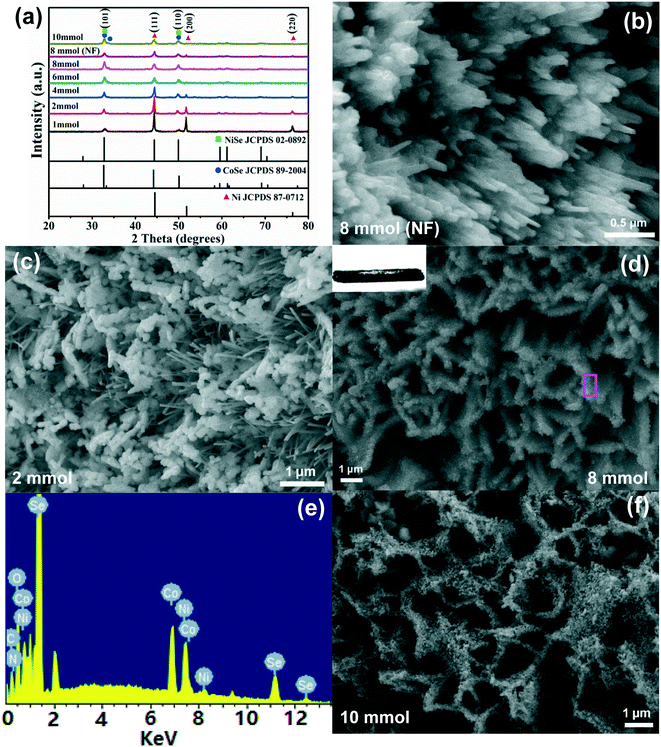 |
| Fig. 3 (a) XRD pattern of NiCoSe (1 mmol), NiCoSe (2 mmol), NiCoSe (4 mmol), NiCoSe (6 mmol), NiCoSe (8 mmol), NiSe (8 mmol) and NiCoSe (10 mmol); SEM images of (b) NiSe (8 mmol), (c) NiCoSe (2 mmol), (d) NiCoSe (8 mmol) and (f) NiCoSe (10 mmol); (e) EDX spectrum of NiCoSe (8 mmol). | |
The scanning electron microscope (SEM) images present the morphology of the NiCoSe samples by using different molar masses of selenium powder. It is worth noting that NiSe nanowire (NW) arrays can be obtained by direct selenization of Ni foam without the presence of NiCo LDH (Fig. 3b). When 2 mmol of selenium powder are used, the as-obtained sample exhibits both NiSe NWs with a diameter of about 50 nm and a nanosheet-like morphology composed of nanoparticles with an average diameter of about 190 nm (Fig. 3c). When the dosage is increased to 8 mmol, nanosheets with a thickness of 400 nm are well maintained (Fig. 3d). The NiCoSe (8 mmol) nanosheets also exhibit a small contact angle of nearly 0°, which can promote full contact between NiCoSe and the electrolyte (inset in Fig. 3d). Moreover, the superhydrophilic surface would facilitate the release of gas bubbles and keep sufficient electrode working area.57 The EDAX spectrum verifies the existence of both Co and Ni elements with a molar ratio of 10.77
:
9.97 (Fig. 3e), and the amount of the Se element increased as the dosage of Se powder increased (Fig. S2†), suggesting that NiCoSe is successfully synthesized. Moreover, the EDS mappings of the NiCoSe (8 mmol) nanosheets (Fig. S3†) indicate that the Ni, Co and Se elements are homogeneously distributed in the whole nanosheets. On further increasing the dosage of Se powder to 10 mmol, the excess Se2− ions react with NiCo LDH, forming uniform and ultrathin nanosheets with a thickness of 230 nm. The results indicate that there is a strong dependence of morphology on the dosage of selenium powder.
TEM, HRTEM and SAED measurements were further carried out to obtain insight into the morphology and crystal structures of the synthesized materials. Fig. 4a and c show the TEM images of NiCoSe (8 mmol, 10 mmol) nanosheets stripped down from the Ni foam, which is in accord with the SEM observations. As could be observed in Fig. 4a, the nanosheets consist of nanoparticles, indicating that selenization does not damage the morphology of nanosheets. Specifically, their arrangement creates an interconnected porous structure, which is beneficial for the adsorption of the electrolyte, the volume expansion associated with the redox reactions, the charge transfer process and gas release. The HRTEM image (Fig. 4b) shows well-defined lattice fringes with the spacing value of 0.27 nm, corresponding to the (101) planes of NiCoSe.33 As presented in Fig. 4c and d, the NiCoSe (10 mmol) nanosheets display wrinkles, confirming the ultrathin morphology, and the corresponding HRTEM shows lattice fringes with an interplanar distance of 0.27 nm.33 In addition, the spot-like type SAED patterns (insets in Fig. 4b and d) confirm the single crystalline nature of the sample, which is consistent with the results obtained from XRD.
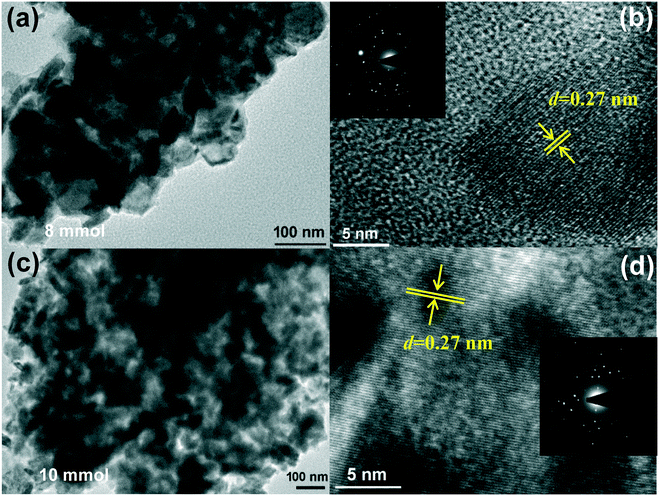 |
| Fig. 4 (a) and (b) TEM, HRTEM and FFT images of NiCoSe (8 mmol); (c) and (d) TEM, HRTEM and FFT images of NiCoSe (10 mmol). | |
The near-surface elemental composition and the valence state of the as-synthesized NiCoSe electrocatalysts were further evaluated by X-ray photoelectron spectroscopy (XPS), which can be seen in Fig. 5. The XPS survey spectra (Fig. 5a) show the existence of Ni, Co, Se, O, and C elements. The C 1s and O 1s signals arise from the unavoidable surface adsorption or slight surface oxidation.58 The high-resolution XPS spectrum of Co 2p for NiCo LDH (Fig. 5b, red line) shows two spin–orbit doublets at 781.7 and 797.19 eV, which can be ascribed to the 2p 3/2 and 2p 1/2 orbitals of Co 2p.59 The peaks at 786.3 and 803.0 eV are the corresponding satellite (identified as Sat.) bands.60,61 After the selenization reaction, the peak at 778.35 eV for NiCoSe (2 mmol) and NiCoSe (8 mmol) is due to Co0, which can be attributed to the strong reducibility of superhydride.59 As displayed in the Ni 2p spectrum (Fig. 5c, blue line), two main peaks centered at 855.8 and 873.6 eV along with two satellite peaks at 861.5 and 880 eV can be ascribed to Ni 2p 3/2 and Ni 2p 1/2 for NiCoSe (8 mmol), suggesting the existence of Ni2+ ions.62–65 The binding energies at 852.9 and 870.3 eV can be assigned to the metallic Ni 2p arising from the Ni foam substrate.58 In contrast, the spin–orbit splitting values of Ni 2p spectra for NiCoSe (2 mmol) and NiCoSe (8 mmol) are almost the same, indicating that the Ni2+ ions maintain the divalent state during the surface selenization reaction. In addition, the peak intensity of the Ni 2p for the NiCoSe nanosheets is higher than that for the NiCo LDH, proving the conversion of some of the metallic Ni to NiCoSe after the hydrothermal process. For the Se 3d high-resolution XPS spectra (Fig. 5d), two peaks located at 54.3 and 55.2 eV are attributed to the 3d 5/2 and 3d 3/2 of Se2− ions,61,63 whereas the peak at 59.2 eV can be assigned to the Se–O bonds because of the surface oxidation.66
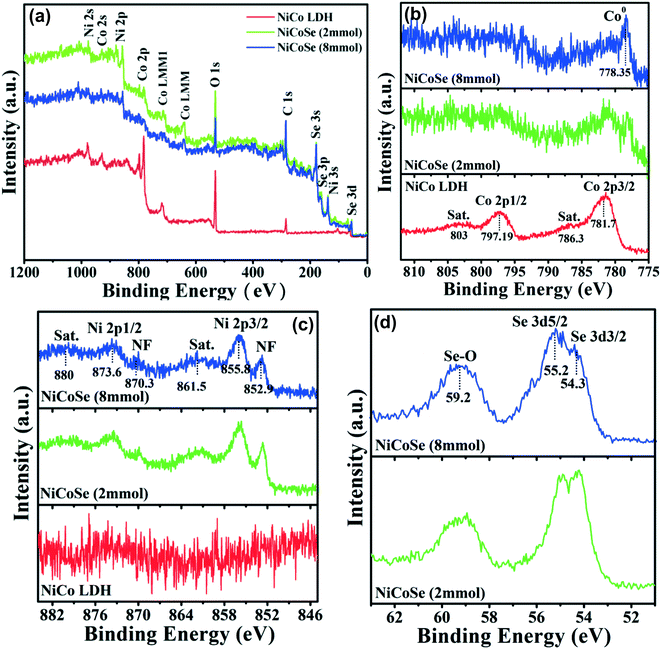 |
| Fig. 5 (a) XPS survey spectra of NiCo LDH, NiCoSe (2 mmol) and NiCoSe (8 mmol); (b–d) high-resolution binding energy spectra of Co 2p, Ni 2p and Se 3d, respectively. | |
The electrocatalytic HER performance of the samples was assessed using a standard three-electrode cell in 1 M KOH solution. The products grown on the Ni foam substrate were directly used as the working electrode. For comparison, the bare NF and commercial Pt/C spread on the NF were also measured under the same conditions. Fig. 6a and b show the iR-corrected linear sweep voltammetry (LSV) curves on a reversible hydrogen electrode (RHE) scale. As expected, the commercial Pt/C on NF shows the best catalytic performance (70 mV), while the bare NF exhibits the lowest activity (278 mV). After selenization, the NiCoSe (8 mmol) sample only needs an overpotential of 170 mV to attain a current density of 10 mA cm−2, which is lower than those of NiCoSe (1 mmol) (231 mV), NiCoSe (2 mmol) (236 mV), NiCo LDH (229 mV), NiCoSe (4 mmol) (222 mV), NiCoSe (6 mmol) (182 mV) and NiCoSe (10 mmol) (193 mV). To investigate the HER kinetics mechanism, the Tafel slopes of the catalysts are calculated. As shown in Fig. 6c, the Tafel slope of NiCoSe (8 mmol) is 82.3 mV dec−1, which is less than those of NF (153.4 mV dec−1), NiCoSe (1 mmol) (106.9 mV dec−1), NiCoSe (2 mmol) (130.9 mV dec−1), NiCoSe (4 mmol) (130.3 mV dec−1), NiCo LDH (128.3 mV dec−1), NiCoSe (6 mmol) (107.4 mV dec−1) and NiCoSe (10 mmol) (88.8 mV dec−1), suggesting that the HER process follows the Volmer–Heyrovsky mechanism (the Tafel slope value between 40 and 120 mV dec−1).67
| H2O + e− + * ⇌ Hads + OH− Volmer reaction | (1) |
| Hads + H2O + e− ⇌ H2 + OH− + * Heyrovsky reaction | (2) |
where * stands for the catalytic active site and H
ads donates the adsorbed hydrogen on the catalyst surface. Such an overpotential and Tafel slope value are also lower than those of previously reported electrode materials (Table S1
†), indicating the excellent HER electrocatalytic activity and faster HER kinetics of NiCoSe (8 mmol). It can be attributed to the following reasons: (1) the
in situ self-supported NiCoSe nanosheets on 3D NF without using polymer binders could prevent the blocking of active sites and facilitate the transfer of electrons; (2) the 3D porous structure makes more surface area contact with the electrolyte and results in more exposed active sites, facilitating the hydrogen generation. Electrochemical impedance spectroscopy (EIS) measurement was also performed to evaluate the interfacial reactions and the charge transfer kinetics during the HER process (
Fig. 6d). The smaller semicircular radius implies a smaller charge transfer resistance (
Rct). It can be clearly seen that the NiCoSe (8 mmol) nanosheets exhibit the smallest
Rct of 2.614 Ω, suggesting the fastest electron transfer ability on the surface of the electrode. Moreover, the HER stability of the NiCoSe (8 mmol) nanosheets can be observed in
Fig. 6e and f. Under the current density of 10 mA cm
−2, the potential is maintained for over 40 h without obvious change (
Fig. 6e). Simultaneously, the polarization curve is retained for 1000 cycles at a sweep rate of 10 mV s
−1 in 1 M KOH (
Fig. 6f).
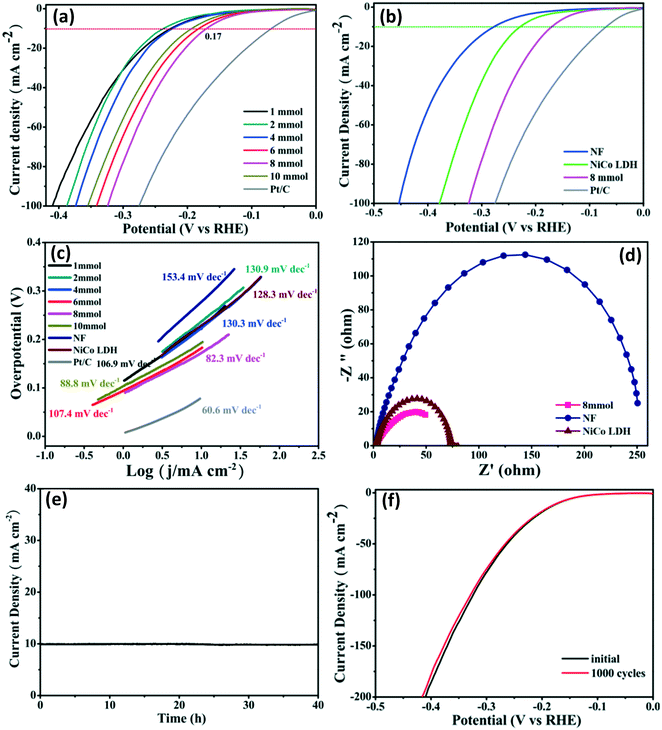 |
| Fig. 6 (a)–(c) LSV curves and the corresponding Tafel plots of NiCoSe (1 mmol), NiCoSe (2 mmol), NiCoSe (4 mmol), NiCoSe (6 mmol), NiCoSe (8 mmol), NiCoSe (10 mmol), NF, NiCo LDH and Pt/C for the HER; (d) electrochemical impedance spectroscopy of NiCoSe (8 mmol), NF and NiCo LDH; (e) chronoamperometry (I–t) curve of NiCoSe for the HER at 10 mA cm−2 in 1 M KOH; (f) LSV curves before and after the durability test. | |
The electrocatalytic OER performance of the as-prepared electrocatalysts was tested in a standard three-electrode system in N2-saturated 1 M KOH electrolyte. The bare NF, NiCo LDH precursor and commercial IrO2 coated on NF were also tested under the same conditions for comparison. As can be seen from the polarization curves in Fig. 7a, the NiCoSe nanosheets show better OER electrocatalytic activity than NiCo LDH, IrO2 and NF. Among all the NiCoSe samples, the NiCoSe (2 mmol) nanosheets exhibit the highest OER electrocatalytic activity with the lowest overpotential of 278 mV to reach the current density of 20 mA cm−2. The OER activities of the previously reported electrocatalysts supported on NF are also summarized in Table S2,† which clearly demonstrates the excellent OER performance of the NiCoSe (2 mmol) nanosheets. The OER kinetics was also assessed using Tafel plots and electrochemical impedance spectroscopy (EIS). The NiCoSe (2 mmol) nanosheets exhibit the lowest Tafel slope (92 mV dec−1, Fig. 7b) and the smallest Rct value (2.325 Ω, Fig. 7c), implying the favorable catalytic reaction kinetics and the rapid charge-transfer rate during the OER process, respectively. The excellent OER performance is mainly attributed to the unique morphology and high activity. Firstly, the NiSe NWs are synthesized on the Ni foam, not only increasing the transfer of electrons from the NF to the topmost NiSe NWs but also providing more active sites to increase the OER activity. Secondly, the nanosheets composed of nanoparticles allow the penetration of water molecules, leading to full contact between the NiCoSe (2 mmol) and electrolyte. In addition, the internal gap between the nanoparticles is suitable for the release of the gaseous products. Similar to the HER case, the NiCoSe (2 mmol) sample also exhibits excellent OER stability with a slight decay after 40 h (Fig. 7e), which is supported by the almost overlapping LSV curves before and after durability testing (inset in Fig. 7e).
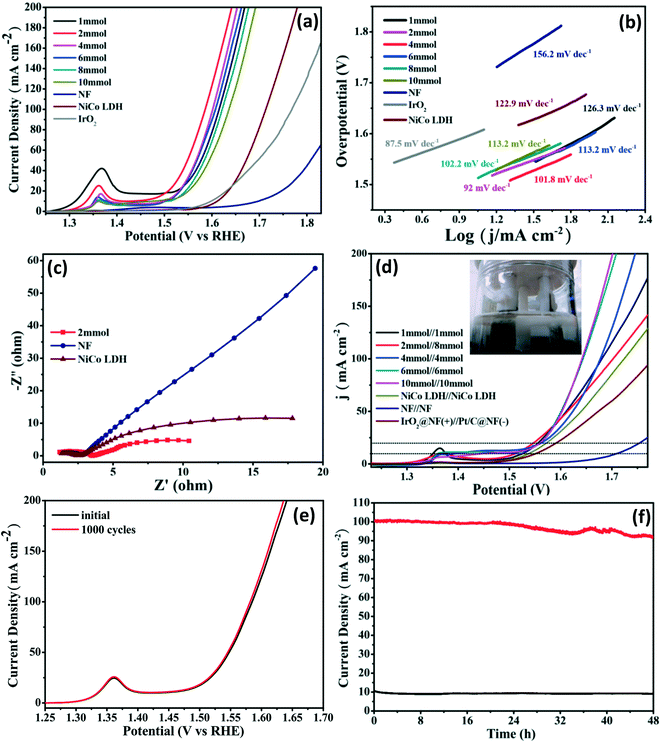 |
| Fig. 7 (a) and (b) LSV curves and the corresponding Tafel plots of NiCoSe (1 mmol), NiCoSe (2 mmol), NiCoSe (4 mmol), NiCoSe (6 mmol), NiCoSe (8 mmol), NiCoSe (10 mmol), NF, NiCo LDH and IrO2 for the OER; (c) electrochemical impedance spectroscopy of NiCoSe (2 mmol), NF and NiCo LDH; (d) LSV curves of NiCoSe (1 mmol)‖NiCoSe (1 mmol), NiCoSe (2 mmol)‖NiCoSe (8 mmol), NiCoSe (4 mmol)‖NiCoSe (4 mmol), NiCoSe (6 mmol)‖NiCoSe (6 mmol), NiCoSe (10 mmol)‖NiCoSe (10 mmol), NiCo LDH‖NiCo LDH, NF‖NF and IrO2@NF(+)‖Pt/C@NF(−) for overall water splitting; (e) chronoamperometry (I–t) curve of NiCoSe for the OER at 10 mA cm−2 in 1 M KOH (inset: LSV curves before and after the durability test); (f) chronopotentiometry (I–t) curves of the overall water splitting at 10 mA cm−2 and 100 mA cm−2. | |
Encouraged by the superior HER and OER performance of the NiCoSe nanosheets, a two-electrode cell was assembled for assessing the overall water splitting behavior in 1 M KOH solution, in which the as-synthesized NiCoSe samples were applied as both cathodes and anodes. The full cell of 2 mmol‖8 mmol (red line) requires a low overpotential of 1.51 V to initiate a current density of 10 mA cm−2 (1.54 V at 20 mA cm−2) (Fig. 7d), which is lower than those of NF‖NF (1.71 V), commercial IrO2@NF(+)‖Pt/C@NF(−) (1.55 V) and NiCo LDH//NiCo LDH (1.54 V). We captured the digital image of the working full cell of 2 mmol‖8 mmol (inset in Fig. 7d), in which the vigorous gas release behavior can be observed. Remarkably, the electrocatalytic performance of the full cell of 2 mmol‖8 mmol is also better than those of previously reported bifunctional electrocatalysts (Table S3†). As shown in Fig. 7f, the electrocatalysts produce a stable current density of 10 and 100 mA cm−2 for 48 h, confirming the long-term stability of the as-prepared NiCoSe nanosheets.
Further, XRD and SEM analyses were performed after the HER and OER tests to prove the active site formation and structural changes. The XRD patterns indicate that the structure of NiCoSe nanosheets has no obvious change after the HER and OER tests (Fig. 8a, c and e). The SEM image of NiCoSe (8 mmol) nanosheets after the HER (Fig. 8b) and NiCoSe (10 mmol) nanosheets after the OER (Fig. 8f) indicate that the original morphology is substantially maintained after 1000 cycles. It is worth noting that the crystallinity of the nanosheets become worse. The metal cations would be oxidized to the higher charge states during the OER reaction, resulting in a layer of NiOOH formed on the top of NiSe NWs, which is revealed by the SEM image of NiCoSe (2 mmol) nanosheets (Fig. 8d). These highly oxidative metal cations are proved as the electrocatalytic active sites.68
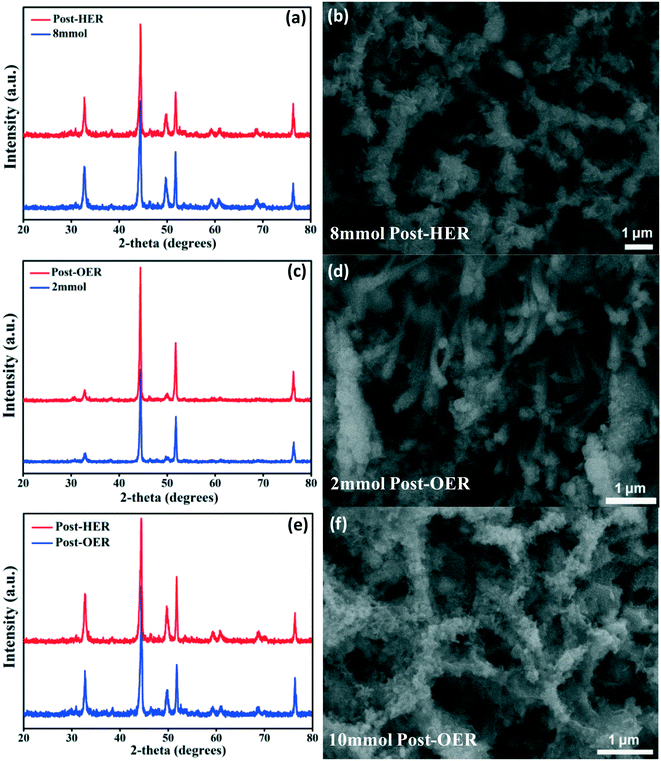 |
| Fig. 8 (a) and (b) XRD pattern and SEM image of NiCoSe (8 mmol) of the post-HER test; (c) and (d) XRD pattern and SEM image of NiCoSe (2 mmol) of the post-OER test; (e) and (f) XRD pattern and SEM image of NiCoSe (10 mmol) of the post-HER and post-OER tests. | |
Conclusions
In summary, novel 2D porous NiCoSe nanosheet arrays were grown on Ni foam using ZIF-67 as a precursor for overall water splitting. They exhibited outstanding bifunctional electrocatalytic performances and superior durability for both the HER and OER. The needed overpotential was 170 mV for HER and 278 mV for OER to reach the current density of 10 mA cm−2 and 20 mA cm−2. Owing to the excellent bifunctional catalytic activity, an efficient water electrolyzer was obtained using NiCoSe as both anode and cathode, yielding a current density of 10 mA cm−2 at a low voltage of 1.51 V as well as long-term stability. The excellent OER, HER, and overall water splitting performance in alkaline solution is attributed to the highly exposed electrochemical surface area, mesoporous structure, and the synergistic effect between NiCoSe nanosheet arrays and Ni foam.
Conflicts of interest
The authors declare that they have no conflict of interest.
Acknowledgements
This work was supported by the National Natural Science Foundation of China (No. 61705079), the Program for the Development of Science and Technology of Jilin Province (Item No. 20190701021GH, 20190103039JH) and the Thirteenth Five-Year Program for Science and Technology of the Education Department of Jilin Province (Item No. JJKH20191019KJ, JJKH20190994KJ).
References
- S. Chu and A. Majumdar, Opportunities and challenges for a sustainable energy future, Nature, 2012, 488, 294–303 CrossRef CAS
.
- Z. W. Seh, J. Kibsgaard, C. F. Dickens, I. Chorkendorff, J. K. Nørskov and T. F. Jaramillo, Combining theory and experiment in electrocatalysis: insights into materials design, Science, 2017, 355, 4998 CrossRef
.
- S. Yang, J. C. Yao, Y. N. Quan, M. Y. Hu, R. Su, M. Gao, D. L. Han and J. H. Yang, Monitoring the charge transfer process in a Nd-doped semiconductor based on photoluminescence and SERS technology, Light: Sci. Appl., 2020, 9, 1–7 CrossRef CAS
.
- A. Landman, H. Dotan, G. E. Shter, M. Wullenkord, A. Houaijia, A. Maljusch, G. S. Grader and A. Rothschild, Photoelectrochemical water splitting in separate oxygen and hydrogen cells, Nat. Mater., 2017, 16, 646–651 CrossRef CAS
.
- A. Alinezhad, L. Gloag, T. M. Benedetti, S. Cheong, R. F. Webster, M. Roelsgaard, B. B. Iversen, W. Schuhmann, J. J. Gooding and R. D. Tilley, Direct growth of highly strained Pt islands on branched Ni nanoparticles for improved hydrogen evolution reaction activity, J. Am. Chem. Soc., 2019, 141, 16202–16207 CrossRef CAS
.
- Y. Yan, T. He, B. Zhao, K. Qi, H. F. Liu and B. Y. Xia, Metal/covalent–organic frameworks-based electrocatalysts for water splitting, J. Mater. Chem. A, 2018, 6, 15905–15926 RSC
.
- J. Yan, Z. Yao, M. Jaroniecb and Z. Q. Shi, Design of electrocatalysts for oxygen- and hydrogen-involving energy conversion reactions, Chem. Soc. Rev., 2015, 44, 2060–2086 RSC
.
- M. G. Schultz, T. Diehl, G. P. Brasseur and W. Zittel, Air pollution and climate-forcing impacts of a global hydrogen economy, Science, 2016, 302, 624–627 CrossRef
.
- N. Mahmood, Y. Yao, J. W. Zhang, L. Pan, X. Zhang and J. J. Zou, Electrocatalysts for hydrogen evolution in alkaline electrolytes: Mechanisms, challenges, and prospective solutions, Adv. Sci., 2018, 5, 1700464 CrossRef
.
- K. S. Joya, Y. F. Joya, K. Ocakoglu and R. Krol, Water-splitting catalysis and solar fuel devices: Artificial leaves on the move, Angew. Chem., Int. Ed., 2013, 52, 10426–10437 CrossRef CAS
.
- A. P. Wu, Y. Xie, H. Ma, C. G. Tian, Y. Gu, H. J. Yan, X. M. Zhang, G. Y. Yang and H. G. Fu, Integrating the active OER and HER components as the heterostructures for the efficient overall water splitting, Nano Energy, 2018, 44, 353–363 CrossRef CAS
.
- J. Bao, Z. L. Wang, J. F. Xie, L. Xu, F. C. Lei, M. Guan, Y. Zhao, Y. P. Huang and H. M. Li, A ternary cobalt-molybdenum-vanadium layered double hydroxide nanosheet array as an efficient bifunctional electrocatalyst for overall water splitting, Chem. Commun., 2019, 55, 3521–3524 RSC
.
- X. X. Zou and Y. Zhang, Noble metal-free hydrogen evolution catalysts for water splitting, Chem. Soc. Rev., 2015, 44, 5148–5180 RSC
.
- H. T. Yuan, Y. Z. Wang, C. X. Yang, Z. Z. Liang, M. X. Chen, W. Zhang, H. Q. Zheng and R. Cao, Ultra-thin Co-Fe layered double hydroxide hollow nanocubes for efficient electrocatalytic water oxidation, ChemPhysChem, 2019, 20, 2964–2967 CrossRef CAS
.
- J. G. Li, H. C. Sun, L. Lv, Z. S. Li, X. Ao, C. H. Xu, Y. Li and C. D. Wang, Metal–organic framework-derived hierarchical (Co,Ni)Se2@NiFe LDH hollow nanocages for enhanced
oxygen evolution, ACS Appl. Mater. Interfaces, 2019, 11, 8106–8114 CrossRef CAS
.
- X. Y. Ding, W. W. Li, H. P. Kuang, M. Qu, M. Y. Cui, C. H. Zhao, D. C. Qi, F. E. Oropeza, K. H. L. Zhang and K. H. L. Zhang, An Fe stabilized metallic phase of NiS2 for the highly efficient oxygen evolution reaction, Nanoscale, 2019, 11, 23217–23225 RSC
.
- W. J. Xu, F. L. Lyu, Y. C. Bai, A. Q. Gao, J. Feng, Z. X. Cai and Y. D. Yin, Porous cobalt oxide nanoplates enriched with oxygen vacancies for oxygen evolution reaction, Nano Energy, 2018, 43, 110–116 CrossRef CAS
.
- J. Q. Zhang, Y. F. Zhao, X. Guo, C. Chen, C. L. Dong, R. S. Liu, C. P. Han, Y. D. Li, Y. Gogotsi and G. X. Wang, Single platinum atoms immobilized on an MXene as an efficient catalyst for the hydrogen evolution reaction, Nat. Catal., 2018, 1, 985–992 CrossRef CAS
.
- S. Laha, Y. Lee, F. Podjaski, D. Weber, V. Duppel, L. M. Schoop, F. Pielnhofer, C. Scheurer, K. Müller, U. Starke, K. Reuter and B. V. Lotsch, Ruthenium oxide nanosheets for enhanced oxygen evolution catalysis in acidic medium, Adv. Energy Mater., 2019, 9, 1803795 CrossRef
.
- Y. Lee, J. Suntivich, K. J. May, E. E. Perry and Y. Shao Horn, Synthesis and activities of rutile IrO2 and RuO2 nanoparticles for oxygen evolution in acid and alkaline solutions, J. Phys. Chem. Lett., 2012, 3, 399–404 CrossRef CAS
.
- F. Zeng, C. Broicher, J. P. Hofmann, S. Palkovits and R. Palkovits, Facile synthesis of sulfur-containing transition metal (Mn, Fe, Co, and Ni) (Hydr)oxides for efficient oxygen evolution reaction, ChemCatChem, 2019, 12, 710–716 CrossRef
.
- E. Aslan, A. Sarilmaz, F. Ozel, I. Hatay Patir and H. H. Girault, Catalytic hydrogen evolution by molybdenum-based ternary metal sulfide nanoparticles, ACS Appl. Nano Mater., 2019, 2, 7204–7213 CrossRef CAS
.
- I. S. Kwon, I. H. Kwak, T. T. Debela, H. G. Abbas, Y. C. Park, J. P. Ahn, J. Park and H. S. Kang, Se-rich MoSe2 nanosheets and their superior electrocatalytic performance for hydrogen evolution reaction, ACS Nano, 2020, 14, 6295–6304 CrossRef CAS
.
- D. X. Yang, W. Q. Hou, Y. J. Lu, W. L. Zhang and Y. F. Chen, Cobalt phosphide nanoparticles supported within network of N-doped carbon nanotubes as a multifunctional and scalable electrocatalyst for water splitting, J. Energy Chem., 2021, 52, 130–138 CrossRef
.
- Y. H. Liu, Z. Y. Jin, X. Q. Tian, X. Q. Li, Q. Zhao and D. Xiao, Core-shell copper oxide@nickel/nickel-iron hydroxides nanoarrays enabled efficient bifunctional electrode for overall water splitting, Electrochim. Acta, 2019, 318, 695–702 CrossRef CAS
.
- H. H. Yang, Y. Huang, W. Y. Teoh, L. J. Jiang, W. J. Chen, L. Zhang and J. H. Yan, Molybdenum selenide nanosheets surrounding nickel selenides sub-microislands on nickel foam as high-performance bifunctional electrocatalysts for water splitting, Electrochim. Acta, 2020, 349, 136336 CrossRef CAS
.
- L. Cao, Z. H. Li, K. M. Su, M. L. Zhang and B. W. Cheng, Rational design of hollow oxygen deficiency-enriched NiFe2O4@N/rGO as bifunctional electrocatalysts for overall water splitting, J. Energy Chem., 2021, 54, 595–603 CrossRef
.
- Y. Z. Li, S. W. Li, J. Hu, Y. Y. Zhang, Y. C. Du, X. J. Han, X. Liu and P. Xu, Hollow FeCo-FeCoP@C nanocubes embedded in nitrogen-doped carbon nanocages for efficient overall water splitting, J. Energy Chem., 2021, 53, 1–8 CrossRef
.
- Y. Li, Y. G. Gao, S. Yang, C. Q. Wu and Y. W. Tan, Anion-modulated nickel-based nanoheterostructures as high performance electrocatalysts for hydrogen evolution reaction, J. Mater. Chem. A, 2020, 8, 12013–12027 RSC
.
- S. Anantharaj and S. Noda, Nickel selenides as pre-catalysts for electrochemical oxygen evolution reaction: A review, Int. J. Hydrogen Energy, 2020, 45, 15763–15784 CrossRef CAS
.
- N. Xue, Z. Lin, P. K. Li, P. Diao and Q. F. Zhang, Sulfur-doped CoSe2 porous nanosheets as efficient electrocatalysts for the hydrogen evolution reaction, ACS Appl. Mater. Interfaces, 2020, 12, 28288–28297 CrossRef CAS
.
- M. Yang, Y. Y. Yang, K. Z. Wang, S. W. Li, F. Feng, K. Lan, P. B. Jiang, X. K. Huang, H. L. Yang and R. Li, Facile synthesis of CoSe nanoparticles encapsulated in N-doped carbon nanotubes-grafted N-doped carbon nanosheets for water splitting, Electrochim. Acta, 2020, 337, 135685 CrossRef CAS
.
- K. L. Ao, J. C. Dong, C. H. Fan, D. Wang, Y. B. Cai, D. W. Li, F. L. Huang and Q. F. Wei, Formation of yolk-shelled nickel-cobalt selenide dodecahedral nanocages from metal-organic frameworks for efficient hydrogen and oxygen evolution, ACS Sustainable Chem. Eng., 2018, 6, 10952–10959 CrossRef CAS
.
- Z. B. Feng, E. P. Wang, S. Huang and J. M. Liu, A bifunctional nanoporous Ni-Co-Se electrocatalyst with a superaerophobic surface for water and hydrazine oxidation, Nanoscale, 2020, 12, 4426–4434 RSC
.
- W. X. Chen, Y. W. Zhang, G. L. Chen, Y. M. Zhou, X. Xiang and K. K. Ostrikov, Interface coupling of Ni-Co layered double hydroxide nanowires and cobalt-based zeolite organic frameworks for efficient overall water splitting, ACS Sustainable Chem. Eng., 2019, 7, 8255–8264 CrossRef CAS
.
- J. Y. Zhang, L. Lv, Y. F. Tian, Z. S. Li, X. Ao, Y. C. Lan, J. J. Jiang and C. D. Wang, Rational design of cobalt-iron selenides for highly efficient electrochemical water oxidation, ACS Appl. Mater. Interfaces, 2017, 9, 33833–33840 CrossRef CAS
.
- M. L. Qin, S. M. Li, Y. Z. Zhao, C. Y. Lao, Z. L. Zhang, L. Liu, F. Fang, H. Y. Wu, B. R. Jia, Z. W. Liu, W. Wang, Y. Liu and X. H. Qu, Unprecedented synthesis of holey 2D layered double hydroxide nanomesh for enhanced oxygen evolution, Adv. Energy Mater., 2019, 9, 1803060 CrossRef
.
- R. Xu, R. Wu, Y. M. Shi, J. F. Zhang and B. Zhang, Ni3Se2 nanoforest/Ni foam as a hydrophilic, metallic, and self-supported bifunctional electrocatalyst for both H2 and O2 generations, Nano Energy, 2016, 24, 103–110 CrossRef CAS
.
- K. M. Xiao, L. Zhou, M. F. Shao and M. Wei, (Ni,Co)0.85Se nanosheet arrays derived from layered double hydroxides toward largely enhanced overall water splitting, J. Mater. Chem. A, 2018, 6, 7585–7591 RSC
.
- J. J. Xu, Y. J. Zhao, M. Y. Li, G. L. Fan, L. Yang and F. Li, A strong coupled 2D metal-organic framework and ternary layered double hydroxide hierarchical nanocomposite as an excellent electrocatalyst for the oxygen evolution reaction, Electrochim. Acta, 2019, 307, 275–284 CrossRef CAS
.
- D. L. Jiang, W. X. Ma, R. Yang, B. Quan, D. Li, S. C. Meng and M. Chen, Nickel-manganese bimetallic phosphides porous nanosheet arrays as highly active bifunctional hydrogen and oxygen evolution electrocatalysts for overall water splitting, Electrochim. Acta, 2020, 329, 135121 CrossRef CAS
.
- W. Zhang, D. H. Li, L. Z. Zhang, X. L. She and D. J. Yang, NiFe-based nanostructures on nickel foam as highly efficiently electrocatalysts for oxygen and hydrogen evolution reactions, J. Energy Chem., 2019, 39, 39–53 CrossRef
.
- R. E. Ejikeme, L. Dong, J. Y. Wang, L. L. Wang, W. Yan and J. J. Zhang, MOF-deviated zinc-nickel-cobalt ZIF-67 electrode material for high-performance symmetrical coin-shaped supercapacitors, J. Colloid Interface Sci., 2020, 574, 140–151 CrossRef
.
- Y. Feng, Z. K. Wang, W. D. Fan, Z. X. Kang, S. Feng, L. L. Fan, S. Q. Hu and D. F. Sun, Engineering the pore environment of metal-organic framework membranesviamodification of the secondary building unit for improved gas separation, J. Mater. Chem. A, 2020, 8, 13132–13141 RSC
.
- Q. Wei, J. Sun, P. Song, J. Li, Z. X. Yang and Q. Wang, Spindle-like Fe2O3/ZnFe2O4 porous nanocomposites derived from metalorganic frameworks with excellent sensing performance towards triethylamine, Sens. Actuators, B, 2020, 347, 128205 CrossRef
.
- S. Javanbakht, A. Hemmati, H. Namazi and A. Heydari, Carboxymethylcellulose-coated 5-fluorouracil@MOF-5 nano-hybrid as a bio-nanocomposite carrier for the anticancer oral delivery, Int. J. Biol. Macromol., 2020, 155, 876–882 CrossRef CAS
.
- W. Zhang, R. Li, X. Zhao, Z. Chen, A. W. K. Law and K. Zhou, A cobalt-based metal-organic framework as cocatalyst on BiVO4 photoanode for enhanced photoelectrochemical water oxidation, ChemSusChem, 2018, 11, 2710–2716 CrossRef CAS
.
- J. Rong, J. C. Xu, F. X. Qiu, Y. Zhu, Y. Y. Fang, J. C. Xu and T. Zhang, Sea urchin-like MOF-derived formation of porous Cu3P@C as an efficient and stable electrocatalyst for oxygen evolution and hydrogen evolution reactions, Adv. Mater. Interfaces, 2019, 6, 1900502 CrossRef
.
- K. Nath, K. Bhunia, D. Pradhan and K. Biradha, MOF-templated cobalt nanoparticles embedded in nitrogen-doped porous carbon: a bifunctional electrocatalyst for overall water splitting, Nanoscale Adv., 2019, 1, 2293–2302 RSC
.
- X. J. Wei, Y. H. Zhang, H. C. He, L. Peng, S. H. Xiao, S. R. Yao and P. Xiao, Carbon-incorporated porous honeycomb NiCoFe phosphide nanospheres derived from a MOF precursor for overall water splitting, Chem. Commun., 2019, 55, 10896–10899 RSC
.
- Y. Ge, H. Chu, J. Chen, P. Zhuang, Q. Feng, W. R. Smith, P. Dong, M. Ye and J. Shen, Ultrathin MoS2 nanosheets decorated hollow CoP heterostructures for enhanced hydrogen evolution reaction, ACS Sustainable Chem. Eng., 2019, 7, 10105–10111 CrossRef CAS
.
- L. Yang, H. Li, Y. Yu, Y. Wu and L. Zhang, Assembled 3D MOF on 2D nanosheets for self-boosting catalytic synthesis of N-doped carbon nanotube encapsulated metallic Co electrocatalysts for overall water splitting, Appl. Catal., B, 2020, 271, 118939 CrossRef CAS
.
- K. N. Dinh, X. L. Sun, Z. F. Dai, Y. Zheng, P. L. Zheng, J. Yang, J. W. Xu, Z. G. Wang and Q. Y. Yan, O2 Plasma and cation tuned nickel phosphide nanosheets for highly efficient overall water splitting, Nano Energy, 2018, 54, 82–90 CrossRef CAS
.
- S. F. Sun, C. D. Lv, W. Z. Hong, X. Zhou, F. G. Wu and G. Chen, Dual tuning of composition and nanostructure of hierarchical hollow nanopolyhedra assembled by NiCo-layered double hydroxide nanosheets for efficient electrocatalytic oxygen evolution, ACS Appl. Energy Mater., 2018, 2, 312–319 CrossRef
.
- Q. X. Ma, B. L. Li, F. R. Huang, Q. Pang, Y. B. Chen and J. Z. Zhang, Incorporating iron in nickel cobalt layered double hydroxide nanosheet arrays as efficient oxygen evolution electrocatalyst, Electrochim. Acta, 2019, 317, 684–693 CrossRef CAS
.
- H. C. Chen, M. Q. Fan, C. Li, G. L. Tian, C. J. Lv, D. Chen, K. Y. Shu and J. J. Jiang, One-pot synthesis of hollow NiSe-CoSe nanoparticles with improved
performance for hybrid supercapacitors, J. Power Sources, 2016, 329, 314–322 CrossRef CAS
.
- Z. Y. Lu, W. Zhu, X. Y. Yu, H. C. Zhang, Y. J. Li, X. M. Sun, X. W. Wang, H. Wang, J. M. Wang, J. Luo, X. D. Lei and L. Jiang, Ultrahigh hydrogen evolution performance of under-water “superaerophobic” MoS2 nanostructured electrodes, Adv. Mater., 2014, 26, 2683–2687 CrossRef CAS
.
- C. Tang, N. Y. Cheng, Z. H. Pu, W. Xing and X. P. Sun, NiSe nanowire film supported on nickel foam: An efficient and stable 3D bifunctional electrode for full water splitting, Angew. Chem., Int. Ed., 2015, 54, 9351–9355 CrossRef CAS
.
- X. Y. Wang, Y. Zhou, Y. X. Tuo, Y. Lin, Y. G. Yan, C. Chen, Y. P. Li and J. Zhang, Synthesis and identifying the active site of Cu2Se@CoSe nano-composite for enhanced electrocatalytic oxygen evolution, Electrochim. Acta, 2019, 320, 134589 CrossRef CAS
.
- Y. X. Huang, Z. H. Wang, Y. Jiang, S. J. Li, Z. H. Li, H. Q. Zhang, F. Wu, M. Xie, L. Li and R. J. Chen, Hierarchical porous Co0.85Se@reduced graphene oxide ultrathin nanosheets with vacancy-enhanced kinetics as superior anodes for sodium-ion batteries, Nano Energy, 2018, 53, 524–535 CrossRef CAS
.
- C. L. Jiao, X. J. Bo and M. Zhou, Electrocatalytic water splitting at nitrogen-doped carbon layers-encapsulated nickel cobalt selenide, J. Energy Chem., 2019, 34, 161–170 CrossRef
.
- C. X. Lu, Y. Yan, T. F. Zhai, Y. Z. Fan and W. Zhou, 2-nm-Thick NiCo LDH@NiSe single-crystal nanorods grown on Ni foam as integrated electrode with enhanced areal capacity for supercapacitors, Batteries Supercaps, 2020, 3, 534–540 CrossRef CAS
.
- H. C. Sun, J. G. Li, L. Lv, Z. S. Li, X. Ao, C. H. Xu, X. Y. Xue, G. Hong and C. D. Wang, Engineering hierarchical CoSe/NiFe layered-double-hydroxide nanoarrays as high efficient bifunctional electrocatalyst for overall water splitting, J. Power Sources, 2019, 425, 138–146 CrossRef CAS
.
- X. Shi, H. Wang, S. Ji, V. Linkov, F. S. Liu and R. F. Wang, CoNiSe2 nanorods directly grown on Ni foam as advanced cathodes for asymmetric supercapacitors, Chem. Eng. J., 2019, 364, 320–327 CrossRef CAS
.
- Z. M. Sun, L. Lin, C. Y. Nan, H. F. Li, G. B. Sun and X. J. Yang, Amorphous boron oxide coated NiCo layered double hydroxide nanoarrays for highly efficient oxygen evolution reaction, ACS Sustainable Chem. Eng., 2018, 6, 14257–14263 CrossRef CAS
.
- H. B. Wang, Y. S. Sun, F. Ma, L. Zhou, H. F. Li, L. Zhang, G. J. Chen, Y. K. Xu, Y. N. Chen, K. W. Xu and D. Y. Ma, Se molarity tuned composition and configuration of Ni3Se2/NiSe core-shell nanowire heterostructures for hydrogen evolution reaction, J. Alloys Compd., 2020, 819, 153056 CrossRef CAS
.
- Z. D. Huang, J. H. Liu, Z. Y. Xiao, H. Fu, W. D. Fan, B. Xu, B. Dong, D. Liu, F. N. Dai and D. F. Sun, A MOF-derived coral-like NiSe@NC nanohybrid: an efficient electrocatalyst for the hydrogen evolution reaction at all pH values, Nanoscale, 2018, 10, 22758–22765 RSC
.
- L. Trotochaud, S. L. Young, J. K. Ranney and S. W. Boettcher, Nickel-iron oxyhydroxide oxygen-evolution electrocatalysts: The role of intentional and incidental iron incorporation, J. Am. Chem. Soc., 2014, 136, 6744–6753 CrossRef CAS
.
Footnote |
† Electronic supplementary information (ESI) available. See DOI: 10.1039/d0ce01527j |
|
This journal is © The Royal Society of Chemistry 2021 |
Click here to see how this site uses Cookies. View our privacy policy here.