DOI:
10.1039/D0CS00443J
(Review Article)
Chem. Soc. Rev., 2021,
50, 528-555
The rise of metal–organic polyhedra
Received
2nd July 2020
First published on 9th November 2020
Abstract
Metal–organic polyhedra are a member of metal–organic materials, and are together with metal–organic frameworks utilized as emerging porous platforms for numerous applications in energy- and bio-related sciences. However, metal–organic polyhedra have been significantly underexplored, unlike their metal–organic framework counterparts. In this review, we will cover the topologies and the classification of metal–organic polyhedra and share several suggestions, which might be useful to synthetic chemists regarding the future directions in this rapid-growing field.
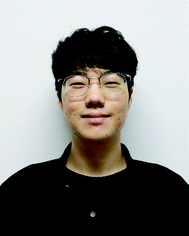
Soochan Lee
| Soochan Lee received his BS degree from the Department of Chemistry at Ulsan National Institute of Science and Technology (UNIST) in 2017. He started a MS/PhD combined program in chemistry at UNIST. He is currently a PhD candidate in chemistry under the direction of Prof. Wonyoung Choe. His research focuses on the design and synthesis of new types of porous materials, including metal–organic polyhedra and zeolitic frameworks, for energy-related applications. |
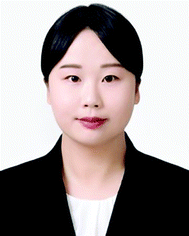
Hyein Jeong
| Hyein Jeong received her BS from the Department of Chemistry at Ulsan National Institute of Science and Technology (UNIST) in 2018 and MS degree from the same department at the same school under the direction of Prof. Wonyoung Choe. Her research focuses on the design and synthesis of new types of metal–organic materials, such as metal–organic polyhedra and zeolitic-imidazolate frameworks, for finding novel properties of the materials. |
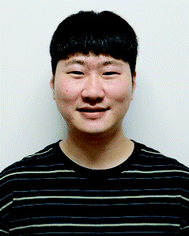
Dongsik Nam
| Dongsik Nam received his BS degree in chemistry (2016) from the Ulsan National Institute of Science and Technology (UNIST). He started a combined M.S./PhD program in chemistry at UNIST. He is currently a PhD candidate under the supervision of Prof. Wonyoung Choe. His research focuses on the design of new porous solids by self-assembly of metal–organic polyhedra. |
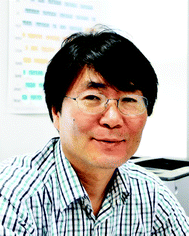
Myoung Soo Lah
| Myoung Soo Lah attended Seoul National University, Korea, for his BSc and MSc and earned his PhD in chemistry from the University of Michigan, Ann Arbor, in 1991. After his postdoctoral research in macromolecular crystallography from the same university, he became a faculty member of the department of chemistry, Hanyang University in 1992 and then moved to Ulsan National Institute of Science and Technology in 2010, where he is presently a professor of the department of chemistry. He is interested in the development of metal–organic systems such as MOPs and MOFs for application in the areas of storage, capture/separation, and catalysis. |
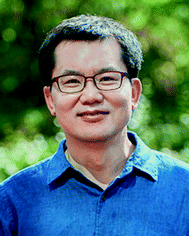
Wonyoung Choe
| Wonyoung Choe received his BS, MS, and PhD from Seoul National University, Seoul National University, and University of Michigan, Ann Arbor, respectively. After finishing his postdoctoral work at Iowa State University and Lawrence Livermore National Laboratory, USA, he became an assistant professor at University of Nebraska-Lincoln. In 2012, he moved to Korea and joined Ulsan National Institute of Science and Technology as a faculty member. Throughout his career, his main research interests have been solids, ranging from intermetallics to soft materials such as metal–organic frameworks and metal–organic polyhedra, for both fundamental and sustainable science. |
1. Introduction
Polyhedra were discovered in the early stages of civilization in the east and the west and are found in art, science, architecture, and humanities to describe not only tangible objects but also metaphysical concepts, thus becoming a form of language. Some of the interesting examples are as follows. Polyhedron-shaped stones traced back to 2000 BC were found in Scotland.1 Ancient people in Roman and Silla dynasties, although they were geometrically separated by the Eurasian continent, both played games with polyhedron-shaped dice.2,3 Plato related five regular polyhedra to elements of the world: earth (cube), air (octahedron), fire (tetrahedron), water (icosahedron), and ether (dodecahedron) in the dialogue Timaeus.4 Kepler modeled the solar system as nested polyhedra in his book Mysterium Cosmographicum, reflecting his theological ideas.5 It is striking to note that a report in 2003 has suggested a dodecahedron as the shape of the universe.6 Scientists have discovered polyhedra in nature at various length scales, from sub-nanometers and beyond, as exemplified by fullerenes,7 the capsids of viruses,8 quasi-crystals,9 and metal nanoparticles.10
The rational design and synthesis of polyhedra using molecules and metals through coordination-driven self-assembly have been a fascinating research topic for synthetic chemists. Specifically, metal–organic polyhedra (MOPs),11 assembled from organic linkers and metal-based secondary building units (SBUs),12 are the examples of such endeavors, becoming an emerging class of metal–organic materials targeted for applications such as catalysis,13,14 gas adsorption,15,16 bio-related applications,17,18 and membranes.19–21 Yaghi and O’Keeffe devised a blueprint for MOPs, including geometric requirements for linkers and SBUs.22 Thanks to in-depth knowledge of polyhedra and their self-assembly, accumulated over the past two decades, new MOPs are continuously reported in the literature, and will further flourish in existing and forthcoming applications.23
In this review, we focus on isolated MOPs instead of MOPs used as building blocks to build extended solids, i.e., metal–organic frameworks (MOFs). For the readers interested in the latter topic, there are several excellent reviews available already.24–26 Interestingly, MOPs have synthetic cousins, e.g., cage compounds built from metal cations, and organic (mostly pyridyl-based) linkers. Fujita and others published reviews on these compounds.27–29 For symmetry-guided synthesis approaches for MOPs and cage compounds, the reviews by Stang,30,31 Yaghi,22 and Raymond32 provide essential information. The article by Alvarez is certainly a good read, with collections of polyhedra in chemistry, art, and history.1 For readers interested in applications, there are several reviews on MOP applications up to 2016.33–35
This review has two goals: (i) to provide readers up-to-date information on types of MOPs, specifically, what they are and how they are classified, and (ii) to highlight new ideas/viewpoints for future directions in MOP assembly.
2. Classification of polyhedra
Polyhedra are three-dimensional objects with a finite number of vertices (corner points), edges (lines connecting pairs of vertices), and faces (polygonal surfaces). Polyhedra can be classified by their transitivity values (p,q,r), where p, q, and r are defined as the types of vertices, edges, and faces of the polyhedron, respectively (Table 1). The used three letter code for polyhedron topology as ‘xyz’ is from the three-letter code of the Reticular Chemistry Structure Resource (RCSR) database (Fig. 1).36 Popular polyhedra are regular, quasi-regular, and semi-regular ones.
Table 1 Basic structural information for the eighteen polyhedra
Polyhedron |
Type of vertex, p |
Type of edge, q |
Type of face, r |
Number of vertices |
Number of edges |
Number of faces |
Dual polyhedron |
Symmetry |
tet
|
1 |
1 |
1 |
4 |
6 |
4 |
tet
|
T
d
|
oct
|
1 |
1 |
1 |
6 |
12 |
8 |
cub
|
O
h
|
cub
|
1 |
1 |
1 |
8 |
12 |
6 |
oct
|
O
h
|
dod
|
1 |
1 |
1 |
20 |
30 |
12 |
ico
|
I
h
|
ico
|
1 |
1 |
1 |
12 |
30 |
20 |
dod
|
I
h
|
cuo
|
1 |
1 |
2 |
12 |
24 |
14 |
rdo
|
O
h
|
ido
|
1 |
1 |
2 |
30 |
60 |
32 |
trc
|
I
h
|
rdo
|
2 |
1 |
1 |
14 |
24 |
12 |
cuo
|
O
h
|
trc
|
2 |
1 |
1 |
32 |
60 |
30 |
ido
|
I
h
|
trp
|
1 |
2 |
2 |
6 |
9 |
5 |
tbp
|
D
3h
|
twc
|
2 |
4 |
3 |
12 |
24 |
14 |
— |
D
3h
|
mtq-d
|
2 |
2 |
3 |
9 |
18 |
11 |
mtq
|
D
3h
|
bds
|
2 |
4 |
2 |
8 |
18 |
12 |
bds-d
|
D
2d
|
ghm
|
4 |
4 |
4 |
20 |
36 |
18 |
— |
D
2d
|
tct
|
2 |
3 |
3 |
9 |
21 |
14 |
tct-d
|
D
3h
|
hmg
|
5 |
5 |
3 |
23 |
42 |
21 |
— |
D
3h
|
csa
|
2 |
3 |
2 |
10 |
24 |
16 |
csa-d
|
D
4d
|
xum
|
4 |
5 |
4 |
26 |
48 |
24 |
— |
D
4d
|
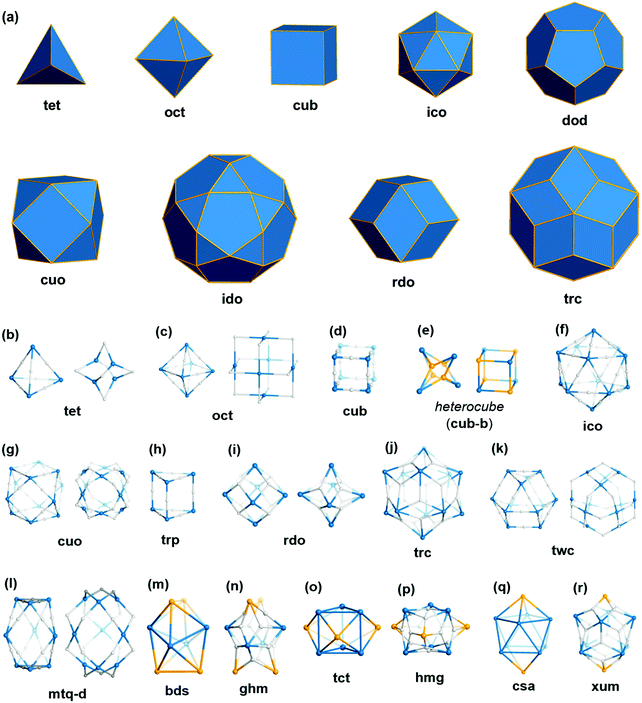 |
| Fig. 1 Description of the polyhedra covered in this review. The three boldfaced letters (xyz) are the RCSR code for the polyhedra.22 (a) Nine edge-transitive polyhedra, specifically the five regular solids, i.e., tetrahedron (tet), octahedron (oct), cube (cub), icosahedron (ico), dodecahedron (dod), two quasi-regular solids, i.e., cuboctahedron (cuo), icosidodecahedron (ido), and Catalan solids as duals of the quasi-regular solids, i.e., rhombic dodecahedron (rdo) and rhombic triacontahedron (trc). (b)–(r) The skeletal view of 17 polyhedra covered in this review, including the heterocube (cub-b), triangular prism (trp), anticuboctahedron (twc), hendecahedron (mtq-d), snub disphenoid and face-capped snub disphenoid (bds, ghm), triaugmented triangular prism and face-capped triaugmented triangular prism (tct, hmg), and gyroelongated square bipyramid and face-capped gyroelongated square bipyramid (csa, xum). | |
Regular (Platonic) polyhedra are vertex-transitive (one kind of vertex), edge-transitive (one kind of edge), and face-transitive (one kind of face). The tetrahedron (tet), cube (cub), octahedron (oct), dodecahedron (dod), and icosahedron (ico) are five different regular polyhedra with transitivity values of (1,1,1).
Quasi-regular polyhedra are vertex- and edge-transitive but not face transitive. The cuboctahedron (cuo) and icosidodecahedron (ido) are two different quasi-regular polyhedra with transitivity values of (1,1,2). The duals37 of quasi-regular polyhedra are face- and edge-transitive but not vertex-transitive. The dual of a cuo is a rhombic dodecahedron (rdo) of transitivity (2,1,1).
Semi-regular polyhedra are vertex-transitive but not edge-transitive polyhedra with regular polygonal faces. Truncated regular polyhedra (tte, tcu, tro, tdo, and tic) and prisms and antiprisms (trp, sap, hpr, hap) are semi-regular polyhedra. Semi-regular convex polyhedra excluding prisms and antiprisms are called Archimedean solids, and the duals of Archimedean solids are called Catalan solids.
When polyhedral species are assembled using inorganic and organic building blocks, becoming MOPs, the vertices of the MOPs are either polytopic inorganic or organic nodes (branch points in the organic building block), and the edges of the MOPs are the linkers between the inorganic nodes and/or between inorganic and organic nodes. To form finite polyhedral species, at least one of the building blocks must be bent or curved.
3. SBUs and linkers for MOP construction
Careful choice of organic linkers and metal SBUs is the key to build the desired MOP topology. We would like to show the representative SBUs and organic linkers in Fig. 2–5. For convenience, k-coordinated nodes (nodes with k links) are referred to as k-c nodes.38 In this review, we will cover 3-c, 4-c, and 5-c metal-based SBUs (Fig. 2), 2-c organic linkers (linear and bent) (Fig. 3 and 4, respectively), and unusual organic linkers as 3-c nodes (Fig. 5) for MOP assembly. The combination of these structure building blocks shows 14 polyhedra, as shown in Table 2.
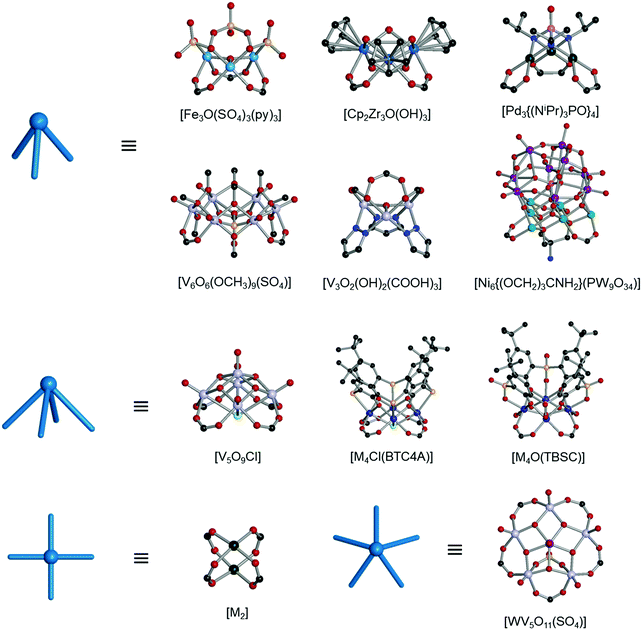 |
| Fig. 2 The list of metal cluster-based SBUs. The first and second rows show the 3-c nodes with 60° coordination angles, [Fe3O(SO4)3(py)3], [Cp2Zr3O(OH)3], [Pd3{(NiPr)3PO}4], [V6O6(OCH3)9(SO4)], [V3O2(OH)2(COOH)3], and [Ni6{(OCH2)3CNH2}(PW9O34)]. The third row shows the 4-c nodes with 60° coordination angles, [V5O9Cl], [M4Cl(BTC4A)], and [M4O(TBSC)]. The fourth row shows the 4-c node with a square-planar coordination geometry, [M2], and the 5-c node with a 60° coordination angle, [WV5O11(SO4)]. | |
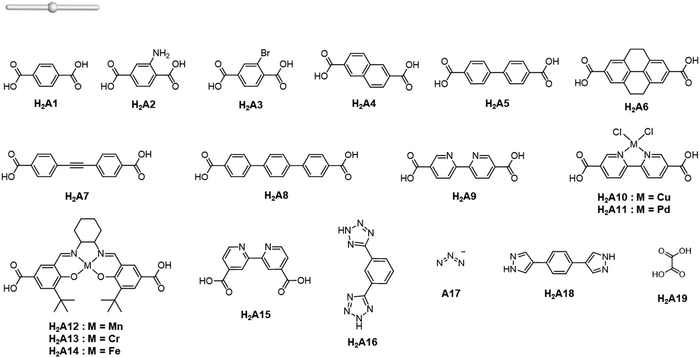 |
| Fig. 3 Linear ditopic organic ligands as linear linkers to construct MOPs. H2A1: 1,4-benzenedicarboxylic acid; H2A2: 2-amino-1,4-benzenedicarboxylic acid; H2A3: 2-bromo-1,4-benzenedicarboxylic acid; H2A4: 2,6-naphthalenedicarboxylic acid; H2A5: 1,1′-biphenyl-4,4′-dicarboxylic acid; H2A6: 4,5,9,10-tetrahydropyrene-2,7-dicarboxylic acid; H2A7: 4,4′-(ethyne-1,2-diyl)dibenzoic acid; H2A8: 1,1′:4′,1′′-terphenyl-4,4′′-dicarboxylic acid; H2A9: 2,2′-bipyridine-5,5′-dicarboxylic acid; H2A10: copper dichloride 2,2′-bipyridine-5,5′-dicarboxylic acid; H2A11: palladium dichloride 2,2′-bipyridine-5,5′-dicarboxylic acid; H2A12: N,N′-bis(3-tert-butyl-5-(carboxyl))salicylidene-1,2-cyclohexanediamine-manganese; H2A13: N,N′-bis(3-tert-butyl-5-(carboxyl))salicylidene-1,2-cyclohexanediamine-chromium; H2A14: N,N′-bis(3-tert-butyl-5-(carboxyl))salicylidene-1,2-cyclohexanediamine-iron; H2A15: 2,2′-bipyridine-4,4′-dicarboxylic acid; H2A16: 1,3-di(1H-tetrazol-5-yl)benzene; A17: azide; H2A18: 1,4-di(1H-pyrazol-4-yl)benzene; and H2A19: oxalic acid. | |
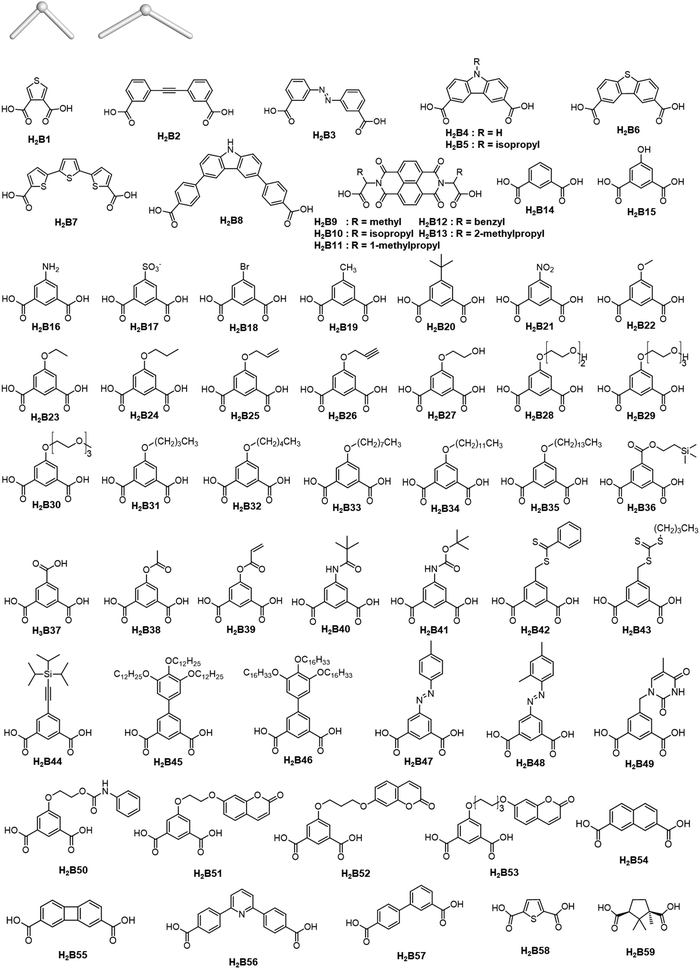 |
| Fig. 4 Bent ditopic organic ligands as bent linkers to construct MOPs. H2B1: thiophene-3,4-dicarboxylic acid; H2B2: 3,3′-(1,2-ethynediyl)dibenzoic acid; H2B3: azobenzene-3,3′-dicarboxylic acid; H2B4: 9H-carbazole-3,6-dicarboxylic acid; H2B5: 9-isopropyl-9H-carbazole-3,6-dicarboxylic acid; H2B6: 2,8-dibenzothiophenedicarboxylic acid; H2B7: 2,2′:5′,2′′-terthiophene-5,5′′-dicarboxylic acid; H2B8: 4,4′-(9H-carbazole-3,6-diyl)dibenzoic acid; H2B9: 2,2′-(1,3,6,8-tetraoxo-1,3,6,8-tetrahydrobenzo[lmn][3,8]phenanthroline-2,7-diyl)dipropionic acid; H2B10: 2,2′-(1,3,6,8-tetraoxo-1,3,6,8-tetrahydrobenzo[lmn][3,8]phenanthroline-2,7-diyl)bis(3-methylbutanoic acid); H2B11: 2,2′-(1,3,6,8-tetraoxo-1,3,6,8-tetrahydrobenzo[lmn][3,8]phenanthroline-2,7-diyl)bis(3-methylpentanoic acid); H2B12: 2,2′-(1,3,6,8-tetraoxo-1,3,6,8-tetrahydrobenzo[lmn][3,8]phenanthroline-2,7-diyl)bis(3-phenylpropanoic acid); H2B13: 2,2′-(1,3,6,8-tetraoxo-1,3,6,8-tetrahydrobenzo[lmn][3,8]phenanthroline-2,7-diyl)bis(4-methylpentanoic acid); H2B14: 1,3-benzenedicarboxylic acid; H2B15: 5-hydroxy-1,3-benzenedicarboxylic acid; H2B16: 5-amino-1,3-benzenedicarboxylic acid; H2B17: 5-sulfo-1,3-benzenedicarboxylic acid; H2B18: 5-bromo-1,3-benzenedicarboxylic acid; H2B19: 5-methyl-1,3-benzenedicarboxylic acid; H2B20: 5-tert-butyl-1,3-benzenedicarboxylic acid; H2B21: 5-nitro-1,3-benzenedicarboxylic acid; H2B22: 5-methoxy-1,3-benzenedicarboxylic acid; H2B23: 5-ethoxy-1,3-benzenedicarboxylic acid; H2B24: 5-n-propyloxy-1,3-benzenedicarboxylic acid; H2B25: 5-allyloxo-1,3-benzenedicarboxylic acid; H2B26: 5-prop-2-ynyloxy-1,3-benzenedicarboxylic acid; H2B27: 5-(2-hydroxyethoxy)-1,3-benzenedicarboxylic acid; H2B28: 5-(2-(2-hydroxyethoxy)ethoxy)-1,3-benzenedicarboxylic acid; H2B29: 5-(2-(2-(2-hydroxyethoxy)ethoxy)ethoxy)-1,3-benzenedicarboxylic acid; H2B30: 5-(2-(2-(2-methoxyethoxy)ethoxy)ethoxy)-1,3-benzenedicarboxylic acid; H2B31: 5-n-butyloxy-1,3-benzenedicarboxylic acid; H2B32: 5-n-pentyloxy-1,3-benzenedicarboxylic acid; H2B33: 5-n-octyloxy-1,3-benzenedicarboxylic acid; H2B34: 5-n-dodecyloxy-1,3-benzenedicarboxylic acid; H2B35: 5-n-tetradecyloxy-1,3-benzenedicarboxylic acid; H2B36: 5-((2-(trimethylsilyl)ethoxy)carbonyl)isophthalic acid; H3B37: 1,3,5-benzenetricarboxylic acid; H2B38: 5-(acetyloxy)isophthalic acid; H2B39: 5-(acryloyloxy)isophthalic acid; H2B40: 5-tert-butylamide-1,3-benzenedicarboxylic acid; H2B41: 5-((tert-butoxycarbonyl)amino)isophthalic acid; H2B42: 5-[[(phenylthioxomethyl)thio]methyl]-1,3-benzenedicarboxylic acid; H2B43: 5-[[[(butylthio)thioxomethyl]thio]methyl]-1,3-benzenedicarboxylic acid; H2B44: 5-(triisopropylsilyl)ethynyl-1,3-benzenedicarboxylic acid; H2B45: 3′,4′,5′-tris(dodecyloxy)-[1,1′-biphenyl]-3,5-dicarboxylic acid; H2B46: 3′,4′,5′-tris(hexadecyloxy)-[1,1′-biphenyl]-3,5-dicarboxylic acid; H2B47: 5-(p-tolyldiazenyl)-1,3-benzenedicarboxylic acid; H2B48: 5-(2,4-dimethylphenyldiazenyl)-1,3-benzenedicarboxylic acid; H2B49: 5-(5-methyl-2,4-dioxo-3,4-dihydropyrimidin-1(2H)-yl)methyl-1,3-benzenedicarboxylic acid; H2B50: 5-{2-[(phenylcarbamoyl)oxy]ethoxy}-1,3-benzenedicarboxylic acid; H2B51: 5-[2-[(2-oxo-2H-1-benzopyran-7-yl)oxy]ethoxy]-1,3-benzenedicarboxylic acid; H2B52: 5-[3-[(2-oxo-2H-1-benzopyran-7-yl)oxy]propoxy]-1,3-benzenedicarboxylic acid; H2B53: 5-[[6-[(2-oxo-2H-1-benzopyran-7-yl)oxy]hexyl]oxy]-1,3-benzenedicarboxylic acid; H2B54: 2,7-naphthalenedicarboxylic acid; H2B55: 2,7-biphenylenedicarboxylic acid; H2B56: 4,4′-(pyridine-2,6-diyl)dibenzoic acid; H2B57: biphenyl-3,4′-dicarboxylic acid; H2B58: thiophene-2,5-dicarboxylic acid; and H2B59: (1R,3S)-1,2,2-trimethylcyclopentane-1,3-dicarboxylic acid. | |
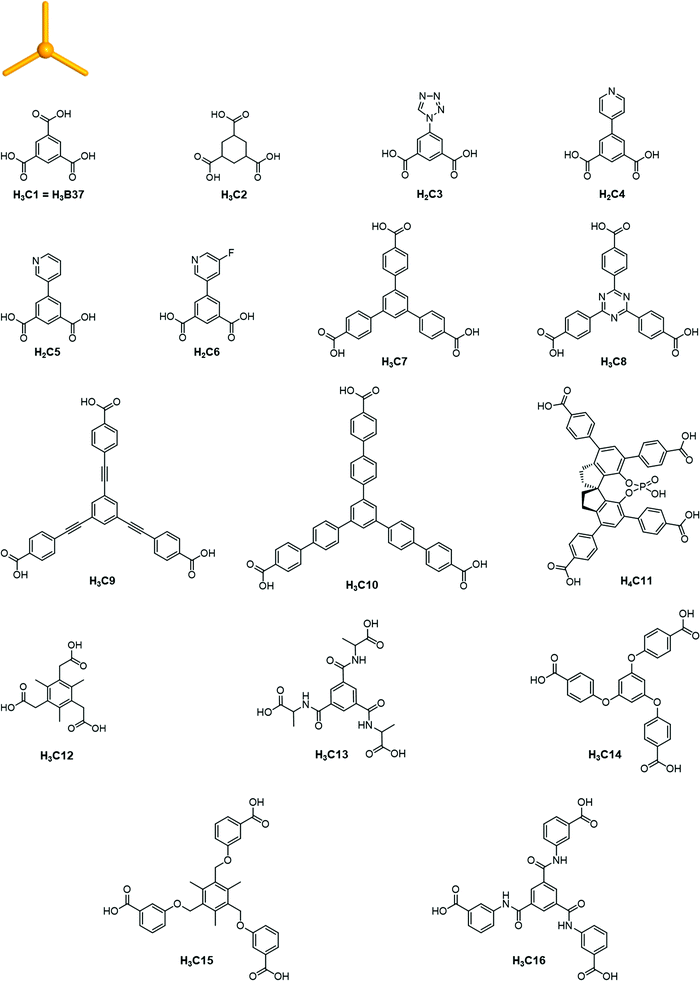 |
| Fig. 5 Tritopic organic ligands as planar 3-c nodes (used as faces) to construct MOPs. H3C1: 1,3,5-benzenetricarboxylic acid; H3C2: cyclohexane-1,3,5-tricarboxylic acid; H2C3: 5-(1H-tetrazol-5-yl)isophthalic acid; H2C4: 5-(pyridin-4-yl)isophthalic acid; H2C5: 5-(pyridine-3-yl)isophthalic acid; H2C6: 5-(5-fluoropyridin-3-yl)isophthalic acid; H3C7: 1,3,5-tris(4-carboxyphenyl)benzene; H3C8: 4,4′,4′′-(1,3,5-triazine-2,4,6-triyl)tribenzoic acid; H3C9: 4,4′,4′′-(benzene-1,3,5-triyltris(ethyne-2,1-diyl))tribenzoic acid; H3C10: 4,4′,4′′-(benzene-1,3,5-triyl-tris(benzene-4,1-diyl))tribenzoic acid; H4C11: 4,4′,6,6′-tetrakis(4-benzoic acid)-1,1′-spinolphosphonate; H3C12: 2,2′,2′′-(2,4,6-trimethylbenzene-1,3,5-triyl)triacetic acid; H3C13: 2,2′,2′′-((benzene-1,3,5-tricarbonyl)tris(azanediyl))tripropionic acid; H3C14: 4,4′,4′′-(benzene-1,3,5-triyltris(oxy))tribenzoic acid; H3C15: 3,3′,3′′-(((2,4,6-trimethylbenzene-1,3,5-triyl)tris(methylene))tris(oxy))tribenzoic acid; H3C16: 3,3′,3′′-[1,3,5-benzenetriyltris(carbonylimino)]tris-benzoic acid. | |
Table 2 Polyhedral topologies assembled from combinations of nodes and linkers
Organic linker |
SBU node |

|

|

|
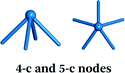
|
|
tet, cub, trp |
oct, cuo, twc, mtq-d |
ico
|
— |
|
Heterocube (cub-b) |
rdo
|
trc
|
ghm, hmg, xum |
4. Regular polyhedra
4.1. Tetrahedron (tet)
The tetrahedron is the simplest of all the convex polyhedra, composed of four vertices, six edges, and four triangular faces. In the assembly of metal–organic tetrahedra, four SBUs as 3-c nodes are linked by six organic 2-c linkers, forming tetrahedron topology, denoted by tet, the RCSR three-letter code. In this section, we survey tet-MOPs assembled from different types of SBUs.
[Fe3O(SO4)3(py)3]4A6.
The M3O SBU, forming a trigonal prism with six carboxylates, has been frequently used in various MOFs.39–41 For example, in MIL-101, a supertetrahedron with 4 M3O SBUs and organic ligands generate remarkable nanosized hierarchical pores.41 Yaghi et al. reported an isoreticular series of MOPs assembled from 3-connected Fe3O clusters and ditopic organic linkers by blocking binding sites with SO42− counter anions.15 The resulting 3-c Fe3O cluster [Fe3O(SO4)3(py3)] (py = pyridine) was capped with three bridging sulfate groups located on one side of the trigonal prism and three pyridines to offer octahedral coordination to the Fe atoms. These clusters connect with three carboxylates arranged at 60° angles and thus serve as 3-c SBUs. The resulting MOPs were assembled from four [Fe3O(SO4)3(py3)] clusters and six ditopic linkers (A1 for IRMOP-50, A5 for IRMOP-51, A6 for IRMOP-52, and A8 for IRMOP-53). The sizes of the tet-MOPs and pores increased with increasing ligand size, but the tetrahedron geometry remained unchanged (Fig. 6). Such isoreticular expansion is common in MOFs42 and can be extended to MOPs. Notably, IRMOP-51 and -53 showed permanent porosity and various gas adsorption capabilities. For example, the hydrogen uptake of IRMOP-51 was comparable with that of MOF-5, exhibiting 81% of its v/v hydrogen capability.
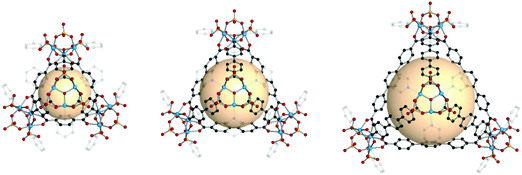 |
| Fig. 6 Structural illustration of IRMOP-50, [Fe3O(SO4)3(py3)]4(A1)6 (left), IRMOP-51, [Fe3O(SO4)3(py3)]4(A5)6 (middle), and IRMOP-53, [Fe3O(SO4)3(py3)]4(A8)6 (right).15 | |
[Cp2Zr3O(OH)3]4A6.
A notable series of emerging MOPs have been the Zr-based MOPs with high chemical and mechanical stability. Although MOFs with Zr clusters have been extensively studied, because of their structural robustness, originated from the high affinities between the oxophilic Zr cations and carboxylate groups,43 Zr-based MOPs started to appear in some recent reports.13,44–50 The first report of Zr-based MOPs by Yuan et al. presented tetrahedral MOPs, composed of trinuclear Zr clusters [Cp3Zr3O(OH)3, Cp = η5-C5H5].44 Considering a plane connecting three Zr cations, the carboxylate linkers are below the plane while the Cp rings are above the plane, providing an ideal SBU geometry as the vertices of tetrahedra. In addition to the tetrahedral cages, ligand variations resulted in several types of Zr-based MOPs, including heterocubes, and cubes with identical Zr clusters.
Tetrahedral cages can be formed with four zirconium clusters and six linear ditopic linkers ([Cp2Zr3O(OH)3]4A6). Representative examples are the ZrT series (Fig. 7).44 Using the A1 linker, a tetrahedral MOP (ZrT-1, [Cp3Zr3O(OH)3]4(A1)6) was prepared. A larger tetrahedral cage (ZrT-3, [Cp3Zr3O(OH)3]4(A5)6) was synthesized using the A5 linker. Both MOPs showed permanent porosity, as confirmed by N2 adsorption. Regarding chemical stability, mass spectra revealed the intact cages in acetonitrile/water solutions under various pH conditions (2.2–9.8).48
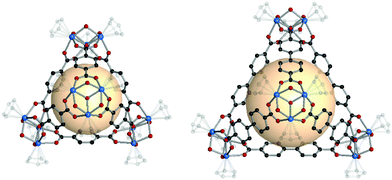 |
| Fig. 7 Structural illustration of ZrT-1, [Cp3Zr3O(OH)3]4(A1)6 (left) and ZrT-3, [Cp3Zr3O(OH)3]4(A5)6 (right).44 | |
When functionalized linkers are utilized, interesting chemical variations of tetrahedral cages are also possible. One such example is MOPs with A9 linkers, which can coordinate with metal cations such as Cu2+ and Pd2+.45 For example, Zr-bpydc ([Cp3Zr3O(OH)3]4(A9)6) with pyridyl groups on the edges of a tetrahedral cage was successfully synthesized. By adding copper and palladium salts in a solution of reactants, Zr-bpydc-CuCl2 ([Cp3Zr3O(OH)3]4(A10)6) and Zr-bpydc-PdCl2 ([Cp3Zr3O(OH)3]4(A11)6) were prepared via post-synthetic metal installation.
Another example is the tetrahedral cages with chiral organic linkers.13 The linkers are A12, A13, and A14, which are salen-based chiral metalloligands. MOPs, [Cp3Zr3O(OH)3]4 (A12 or A13)6, were prepared with enantiopure A12 and A13 (Fig. 8). Three more MOPs were synthesized by mixing two types of linkers (Mn/Cr, Mn/Fe, and Cr/Fe) in a 1
:
1 molar ratio. MOPs with single A12 and A13 were analyzed with single-crystal X-ray diffraction (SCXRD). The structures were distorted tetrahedral cages without a linker disorder. In the two MOP structures, all the linkers retained their chirality. A total of five MOPs, including three mixed-linker MOPs, exhibited similar powder X-ray diffraction (PXRD) patterns and homochirality from the circular dichroism spectra. These mixed-linker MOPs were investigated for sequential asymmetric catalysis, resulting in enhanced properties in epoxidation and ring-opening reactions.
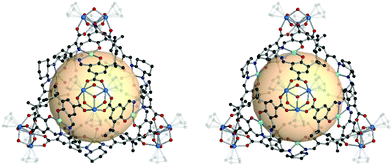 |
| Fig. 8 Structural illustration of [Cp3Zr3O(OH)3]4(A12)6 (left) and [Cp3Zr3O(OH)3]4(A13)6 (right).13 | |
The Choe group reported a tet-Zr-MOP, UMOP-1-NH2, [Cp3Zr3O(OH)3]4(A2)6, employing 2-aminoterephthalic acid (H2A2).46 Compared to ZrT-1, UMOP-1-NH2 showed similar tetrahedral cages with disordered amine groups in four sites of each phenyl ring. The cages were stable in water and methanol. UMOP-1-NH2 was further investigated as a building block for organic condensation reactions, leading to cross-linked tetrahedral cages with flexible alkyl chains. The amine-functionalized cage was also utilized in post-synthetic linker modification, confirmed by mass spectrometry.48
[V3O2(OH)2(COOH)3]4A6 and [V6O6(OCH3)9(XO4)]4A6, X = S or V.
Polyoxometalates (POMs), high-valent early transition metal-oxide anions with structural and functional diversity, are attractive inorganic molecules because of their potential applications in catalysis, magnetism, medicine, and the field of nano/electrochemistry.51–53 POMs have been popular as SBUs for inorganic–organic hybrid materials due to their high connectivity and large-sized clusters.54,55 Among the POMs, polyoxovanadates (POVs) produced various types of coordination molecular cages when combined with organic linkers.
Navarro et al. reported a tet-MOP, constructed from trinuclear POV and ditopic pyrazolate ligands, instead of carboxylate ligands.56 Four [V3O2(OH)2(COOH)3] clusters were connected by pyrazolate with six A18 linkers (Fig. 9). This MOP exhibited permanent porosity, selective adsorption of CO2 over N2, and volatile organic compound separation properties for benzene/cyclohexane.
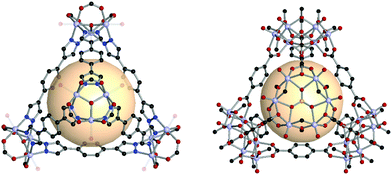 |
| Fig. 9 Structural illustration of [V3O2(OH)2(COOH)3]4(A18)6 (left)56 and VMOP-11, [V6O6(OCH3)9(SO4)]4(A1)6 (right).57 | |
The Su group has successfully demonstrated the reticular synthesis of POV-based MOPs. They showed an unprecedented alkoxo-POV as an SBU for MOPs. The alkoxo-POV, [V6O6(OCH3)9(μ6-SO4)], composed of a hexavanadium sulfur core {V6S}, nine methoxy bridging groups, and six oxo anions, offered 3-c coordinate environments for tetrahedron formation.57 The modular tetrahedral MOPs were assembled from four alkoxo-POV and six ditopic linear ligands for VMOP-11, -12, and -13 (A1 for VMOP-11, A2 for VMOP-12 and A3 for VMOP-13) (Fig. 9). Instead of a {V6S} cluster, an unprecedented {V7} alkoxo-POV was also obtained from a different metal source (VCl3 for {V7} and VOSO4·xH2O for {V6S}).58 The reactions with four [V6O6(OCH3)9(VO4)] clusters and ditopic A1 and A2 ligands afforded VMOP-16 and -17 with tetrahedral geometry. These were isostructures to VMOP-11 and -12. A recent report by Su and coworkers showed a larger VMOP composed of [V6O6(OCH3)9(μ6-SO4)] clusters and 4,4′-(ethyne-1,2-diyl)dibenzoate (A7) linkers.59
[Pd3{(NiPr)3PO}4]4A6.
The d8 metal ions are suitable components for building coordination compounds due to their highly predictable coordination environment.27,28,31 In particular, palladium(II) ions strongly prefer a square-planar coordination geometry, widely utilized to construct polyhedron-shaped coordination cages.27,28 Despite the coordination polyhedra, successfully synthesized with a single Pd(II) center, multi-nuclear Pd clusters are rather scarce in MOP chemistry. Boomishankar et al. reported a tet-MOP assembly with novel Pd3 clusters that were capped by tris(alkylimido)phosphate trianions, [(NiPr)3PO]3− (iPr = iso-propyl). The cluster formula was Pd3[(NiPr)3PO].60 Synthesis with 3-c Pd3 clusters and linear ditopic carboxylates afforded tetrahedral cage assemblies in MOP synthesis. The neutral tetrahedron-shaped [(Pd3[(NiPr)3PO])4(A19)6] was assembled by the bridging of six ditopic oxalate linkers between Pd3 clusters, serving as a 3-c SBU for edge-transitive tetrahedron geometry (Fig. 10). The porosity of this cage was verified by a CO2-sorption experiment at 195 K, and the encapsulation of polar and nonpolar solvent molecules was observed from host–guest studies in solution and the solid-state.
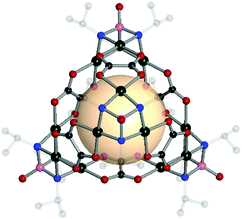 |
| Fig. 10 Structural illustration of [(Pd3[(NiPr)3PO])4(A19)6].60 | |
[(Mo2)(t-Bu-CO2)]4B6.
In a rare case, the 3-c node in tet-MOPs has different coordination bond angles instead of the typical 60° bond angle. Pamore et al. synthesized a Mo2 paddlewheel SBU-based tetrahedron.61 Mo2 units capped by tert-butyl carboxylate (t-Bu-CO2) were connected by three B1 linkers in a T-shaped bridging environment (two at 90° and one at 180°; average: 120°). The formula of the assembled tetrahedron was [(Mo2)4(t-Bu-CO2)4(B1)6]. Due to the T-shaped SBU connection, the carboxylates in the B1 linker were twisted and located out of the thiophene plane. Such distorted molecular geometry is shown in Fig. 11.
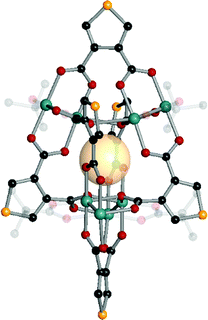 |
| Fig. 11 Structural illustration of [(Mo2)4(t-Bu-CO2)4(B1)6].61 | |
4.2. Octahedron (oct)
An octahedron is a regular convex polyhedron, composed of six vertices, twelve edges, and eight triangular faces. The dual polyhedron of an octahedron is a cube. To construct a molecularly self-assembled octahedron, six 4-c SBUs at the vertices and twelve bridging linkers are required. The RCSR code for an octahedron is oct. The ideal assembly of an oct MOP is the linkage of tetravalent SBUs with 60° coordination bond angles needed for the ditopic linkers. A previous review noted the lack of tetravalent metal SBUs with 60° coordination bond angles for oct MOPs.22 In this section, we cover various types of octahedron-shaped MOPs constructed from various SBUs with 60° or 90° coordination.
[V5O9Cl]6A12.
Due to various oxidation states and numbers of vanadium cations on POV clusters, the connectivity of POV SBUs can be modulated by the synthesis method. The tet-MOPs are commonly formed using 3-c POVs, while oct-MOPs are expected from 4-c POVs.
Nanosized octahedron-shaped MOPs were synthesized from an assembly with six 4-c polyoxo [V5O9Cl] clusters and twelve ditopic carboxylate ligands by Su et al. (A1 for VMOP-1, A2 for VMOP-2, and A3 for VMOP-3) (Fig. 12).62 Their molecular sizes are roughly 2.5 nm. As can be seen in VMOP-6, the isoreticular pore expansion is possible by using an extended organic linker, A4.63 The 4-c [V5O9Cl] SBU is good for building octahedral architectures.
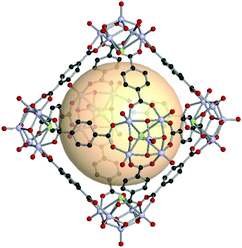 |
| Fig. 12 Structural illustration of VMOP-1, [V5O9Cl]6(A1)12.62 | |
[M4Cl(BTC4A)]6A12 and [M4O(TBSC)]6A12.
Calixarenes are macrocyclic molecules with basket shapes and have been extensively utilized as cavitands in host–guest chemistry due to their versatile conformations.64 In supramolecular chemistry, calixarenes are popular choices for making capsule-like assembled supermolecules directed by simultaneous coordination and covalent bonds.65–67 In particular, the cone formation of calix[4]arene's four hydroxyl groups at the lower rim offers potential as 4-c SBUs.
Hong et al. reported an oct-MOP constructed from p-tert-butylthiacalix[4]arene (H4BTC4A), A1 linkers, and Co(II) cations.68 The post-assembly of deprotonated BTC4A anions and four Co(II) metal cations produced the tetranuclear and 4-c SBU, with the formula [Co4(BTC4A)(μ4-Cl)]. Six [Co4(BTC4A)Cl] nodes were linked by twelve ditopic A1 linkers with 60° coordination bond angles at the SBU center (Fig. 13). The formula for the oct-MOP was [(Co4(BTC4A)(μ4-Cl))6(A1)12]. This MOP exhibited permanent porosity, possibly due to its rigid molecular construction.
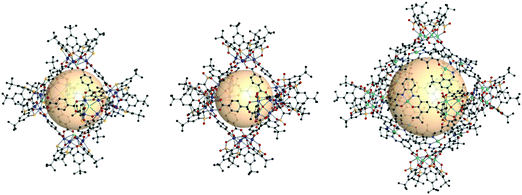 |
| Fig. 13 Structural illustration of [Co4Cl(BTC4A)]6(A1)12 (left),68 [Co4O(TBSC)]6(A1)12 (middle),69 and [Zn4O(TBSC)]6(A12)12 (right).14 | |
Similarly, Wang's group introduced oct-MOPs based on different calix[4]arenes.69 Instead of BTC4A, the calixarenes used were p-tert-butylsulfonylcalix[4]arene (H4TBSC), p-tert-pentylsulfonylcalix[4]arene (H4TPSC), and p-tert-octylsulfonylcalix[4]arene (H4TOSC) for the assembly of oct-MOPs. In the case of TBSC, Co(II) or Ni(II) cations and A1 linkers afforded the octahedral MOSC-II-tBu-M (M = Co or Ni) (Fig. 13). The coordination environment was slightly different with Hong's oct-MOP. The divalent metal cations formed coordination bonds, retaining the octahedral coordination geometry with one oxygen from the sulfonyl group, one oxygen from the hydroxyl group, two oxygens of carboxylate coordination, and one of μ4-H2O molecule, with the oct-MOP formula of [M4(TBSC)(μ4-H2O)]6(A1)12, M = Co or Ni. Interestingly, the extrinsic pores were controlled by the length of the aliphatic moieties of sulfonylcalix[4]arenes. The molecular size of the oct-MOP series increased in the following order: MOSC-II-tBu-M, MOSC-II-tPen-M, and MOSC-II-tOc-M. However, the inner pore sizes of the MOPs were practically the same. Only MOSC-II-tPen-Ni showed selective adsorption of O2 (3.46 Å; kinetic diameter) over N2 (3.64 Å) from the different guest inclusion sizes of the extrinsic pores, due to the modulated aliphatic moieties after removal of solvent molecules.
Chiral metallosalen complexes are well-known asymmetric catalysts for reactions such as the epoxidation of olefins and oxidative kinetic resolution (OKR) of racemic alcohols. Cui et al. designed and synthesized a chiral metallosalen-based oct-MOP.14 The features of the assembly were similar to the MOSC-II-tBu series. The six 4-c [Zn4O(TBSC)] SBUs were connected with twelve linear enantiopure (R,R)-A12 groups. The assembled metallosalen-based oct-MOP contained [Zn4O(TBSC)]6(A12)12, with a size of approximately 4.5 nm (Fig. 13). The efficient and enhanced enantioselectivity was verified for the OKR of secondary alcohols by supramolecular asymmetric catalysis from a metallosalen-based oct-MOP.
The octahedral coordination cages by mixing two [M4Cl(BTC4A)] and four [M4(SO4)(BTC4A)] (M = Co or Fe) were synthesized with eight A15 linkers.70 Unlike the above-mentioned calixarene-based oct-MOPs, the anion, especially SO42−, played a critical role in cage formation. Two A15 linker moieties connected four [M4(SO4)(BTC4A)] SBUs in the equatorial position. One direction of coordination was pyridyl binding, and another was carboxylate coordination to [M4(SO4)(BTC4A)]. Two [M4Cl(BTC4A)] in the axial position were bridged with one of the carboxylates in the A15 linker in the same manner as other oct-MOPs. The fascinating [6+8] condensation afforded the abnormal octahedral cages of [M4Cl(BTC4A)]2[M4(SO4)(BTC4A)]4(A15)8.
(M2)6B12.
Dimetal paddlewheel M2 (mostly Cu2) components are one of the most frequently used SBUs to construct metal–organic materials, including MOFs and MOPs.71 Square planar 4-c nodes offer a wide variety of structures, depending on the ligand geometry of the bridging carboxylates. To assemble oct-MOPs with M2 paddlewheels, ditopic organic linkers with 90° bent angles are needed. The organic linkers used for M2 based oct-MOPs are shown in Fig. 14.
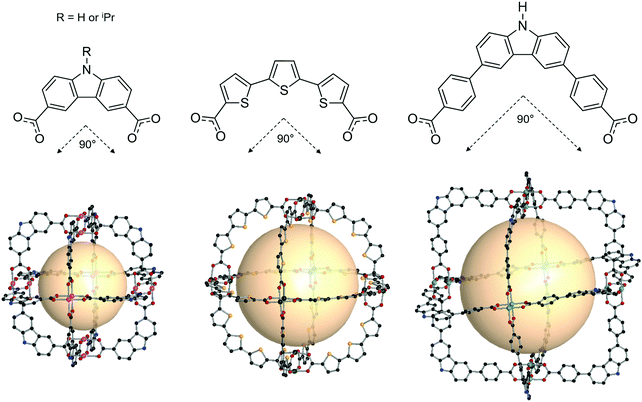 |
| Fig. 14 Chemical diagram of B4 and B5, B7, and B8 ligands, which have 90° bent angles, and an architectural illustration of the oct MOPs resulting from the combination of M2 nodes and each linker.72,73,78 | |
The first type of linker is 9-R-3,6-carbazoledicarboxylate (R = H or iPr). Zhou et al. synthesized an oct-MOP using six Cu2 and twelve 9H-3,6-carbazoledicarboxylate (B4) building blocks (Fig. 14).72 The synthesis procedure was different from the typical one-pot solvothermal synthesis. A solution of the pre-synthesized Cu2-based lantern-type cage and excess B4 linkers afforded the single-crystal oct-MOP, (Cu2)6(B4)12. The Zhou group introduced a solvothermal synthesis of quadruply-bonded Mo2-based oct-MOPs using B4 linkers.73 The Mo-Mo dimer, Mo2(CF3CO2)4, was used as a metal source to construct the rigid Mo2oct-MOP, (Mo2)6(B4)12. Dinuclear Ru2 has shown catalytic activity due to its redox-active core, which makes the building block desired for catalytic applications. Zhou et al. also reported Ru2-based oct-MOPs with B4 linkers, (Ru2)6(B4)12.74 The synthesis from two different oxidation states of Ru sources, Ru2(OAc)4Cl and Ru2(OAc)4 (OAc = acetate), produced the same oct-MOP. The Bloch group recently synthesized a similar counterpart, (Cr2)6(B4)12,75 highly stable in desolvated conditions, unlike other M2-based MOPs. Due to its remarkable stability, (Cr2)6(B4)12 showed the highest Brunauer–Emmett–Teller surface area of all the octahedral M2 MOPs. They also successfully synthesized Ni2- and Co2-based oct-MOPs using 9-isopropyl-3,6-carbazoledicaroxylate (B5).76 In the synthesis of Ni2 and Co2oct-MOPs, only B5 afforded polyhedron structures ((M2)6(B5)12, M = Ni or Co), and B4 produced a two-dimensional MOF. This result clearly demonstrated the importance of ligand functionality to control the dimensionality of the phases. In addition to carbazole-based linkers, the dibenzothiophene linker (B6) with similar bent angles was utilized for the synthesis of the Cu2oct-MOP by Duan and coworkers.77
The second type of linker is 2,2′:5′,2′′-terthiophene-5,5′′-dicarboxylate (B7). The bent angle of B7 was 90° with the cis, cis conformation. The Yaghi group synthesized an octahedral Cu2-based MOP with B7 linkers, MOP-28 (Fig. 14).78 The formula was (Cu2)6(B7)12. The pore size of MOP-28 was slightly larger than that of the (M2)6(B4)12 octahedral MOP. The permanent and high porosity of MOP-28 was verified.
The third type of linker is the extended molecular structure of B4, 4,4′-(9H-carbazole-3,6-diyl)dibenzoate (B8). Zhou et al. synthesized a series of Mo2-based oct MOPs using B4, B8, and B4 with a phenyl ring added (Fig. 14).73 The molecular size of the (Mo2)6(B8)12 MOP was roughly 3.5 nm. Due to phenyl ring rotation by steric hindrance, the cage was slightly distorted compared to B4-based MOPs.
Another linker type for octahedral MOPs includes naphthalene diimide-based linkers.79,80 Mollick et al. utilized linkers with a naphthalene diimide core and terminal amino acid moieties (B9 to B12), leading to bent angles of carboxylic acid groups.79 Four Cu2-based octahedral MOPs were prepared with different amino acid moieties, alanine, valine, isoleucine, and phenylalanine, and increased chemical stability was shown. Boer et al. prepared a similar octahedral cage with a leucine-substituted naphthalene diimide linker (B13) and demonstrated enantioselective sorption and release of small analytes.80
Thus far, we have introduced examples of nearly regular octahedral M2-based MOPs that were comprised of one type of linker with 90° bent angles. Surprisingly, synthesis using two mixed linkers, one with a 60° bent angle and the other with a 120° bent angle (average 90°), can afford distorted octahedral MOPs. A post-reaction using a solution of pre-synthesized cuboctahedron-shaped Cu2 MOPs, composed of B20 and excess of 3,3′-(ethyne-1,2-diyl)-dibenzoate (B2), was used to synthesize the distorted octahedral MOP, (Cu2)6(B20)6(B2)6 (Fig. 15).72 The positions of the six B2 linkers were equatorial, and six B20 were located at the top and bottom connected by three linkers in the MOP. Similarly, Kitagawa et al. reported the distorted oct-MOP, (Cu2)6(B20)6(B3)6, using azobenzene-3,3′-dicarboxylate (B3) instead of B2.81 This anisotropic linker position was utilized to introduce a polymeric moiety to the B20 position.
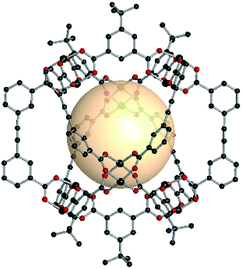 |
| Fig. 15 Structural illustration of the distorted oct MOP, (Cu2)6(B20)6(B2)6.72 | |
4.3. Cube (cub)
A cube, also known as a regular hexahedron, has eight vertices, twelve edges, and six square faces. The dual polyhedron of a cube is an octahedron. Eight 3-c SBUs and twelve linkers on the edges are needed to construct a cube-type assembly, denoted as cub in the RCSR code. The cube is a polyhedron example to perfectly fill the three-dimensional space itself and is a highly promising molecular building block to form diverse architectures. Nevertheless, known examples of cub-MOPs are still rare, compared to tet- and oct-MOPs. The formation of cub-MOPs typically competes with the tet-MOP formation, when ditopic linkers are employed. We cover three types of cub-MOPs, constructed from various SBUs and organic linkers.
[Ni6{(OCH2)3CNH2}(PW9O34)]8B12.
Yang et al. reported a rare example of the polyoxometalate-organic hybrid polyhedron.82 The polyoxometalate [Ni6(Tris)(en)3(B-α-PW9O34)] (Tris = ((OCH2)3CNH2)3−, and en = C2H4(NH2)2) SBU, denoted as Ni6PW9, was constructed by capping Keggin B-α-PW9O34 units on Tris-grafted Ni6 cores. Two carboxylate units of twelve B37 were coordinated to a Ni core in eight Ni6PW9 as an SBU. The assembled POM-based MOP was a cubic cage (Fig. 16). This is the only example of a cube-shaped and POM-based MOP.
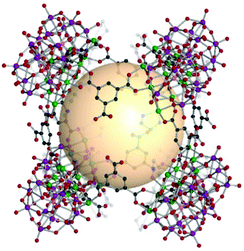 |
| Fig. 16 Structural illustration of [Ni6{(OCH2)3CNH2}(PW9O34)]8(B37)12.82 | |
[Cp2Zr3O(OH)3]8B12.
A cubic Zr-MOP (HCCF-1, [Cp3Zr3O(OH)3]8(B59)12) was formed with Zr clusters at the eight vertices and dicarboxylate linkers at the twelve edges (Fig. 17).47B59 includes a five-membered carbon ring and methyl groups, leading to a bent shape. The reported HCCF-1 crystal structure showed disordered positions of the linkers. Due to the high number of hydrogen bonds between the cubic cages (O–H⋯Cl⋯H–O), HCCF-1 retained crystallinity after desolvation and exhibited microporosity.
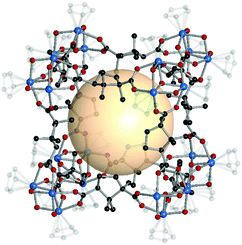 |
| Fig. 17 Structural illustration of HCCF-1, [Cp3Zr3O(OH)3]8(B59)12.47 | |
[M4Cl(BTC4A)]8A12.
Liao et al. reported a unique type of cub-MOP, CIAC-108, based on the calix[4]arene SBU, [Co4(BTC4A)(μ4-Cl)] cluster, used to build oct-MOPs (Fig. 18).83 There are two different ditopic linkers, A16 and A17. Interestingly, the former A16 linker was prepared by an in situ click reaction with 1,3-dicyanobenzene and NaN3 during one-pot synthesis. The two tetrazole moieties in A16 connect the SBUs by occupying eight of the top and bottom edges. The remaining 4 edges in the equatorial positions in the cube were linked by eight N3− linkers, and each edge was occupied by two azide linkers. The formula of CIAC-108 was [{Co4(BTC4A)(μ4-Cl)}8(A16)4(A17)8(μ2-Cl)8]. We consider this MOP with a cubic geometry with the BTC4A-based SBU as a 3-c node, although CIAC-108 can also be seen as a tetragonal prism from a different perspective.
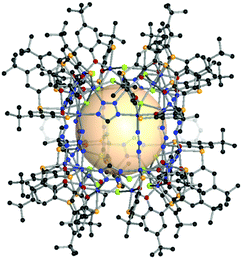 |
| Fig. 18 Structural illustration of CIAC-108, [{Co4(BTC4A)(μ4-Cl)}8(A16)4(A17)8(μ2-Cl)8].83 | |
4.4. Heterocube (cub-b)
MOPs with heterocube (binary cube) structures are comprised of two types of tritopic SBUs. In general, one of four kinds of inorganic SBUs located at the vertices is linked to four 3-c organic nodes. The cage shape resembles a face-capped tetrahedron, but topologically, we classified these MOPs as heterocubes, cub-b.
[Fe3O(SO4)3(py)3]4C4.
A cub-b-MOP, MOP-54, was assembled from four [Fe3O(SO4)3(py3)] SBUs, the same SBUs used to form tet-MOPs (IRMOP-50, -51, -52, -53), and four 1,3,5-benzene-tribenzoate nodes, C7 (Fig. 19).15 MOP-54 ([Fe3O(SO4)3(py)3]4(C7)4) exhibited permanent porosity and CO2, CH4, and benzene adsorption capacities.
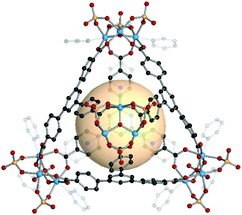 |
| Fig. 19 Structural illustration of MOP-54, [Fe3O(SO4)3(py3)]4(C7)4.15 | |
[Cp3Zr3O(OH)3]4C4.
The cub-b-Zr-MOPs include four Zr clusters and four tritopic carboxylate linkers, [Cp3Zr3O(OH)3]4C4. Two crystal structures of the heterocube cages have been reported, ZrT-2 ([Cp3Zr3O(OH)3]4(C1)4) and ZrT-4 ([Cp3Zr3O(OH)3]4(C7)4) with C7 (Fig. 20).44 Because the 3-c organic ligands observed in the single-crystal structures were relatively flat, the shape of the cages also can be considered to be tetrahedral with tritopic linkers on the faces. ZrT-2 and ZrT-4 are microporous. These MOPs exhibited stability in acetonitrile/water solutions under various pH conditions (2.2–9.8).48
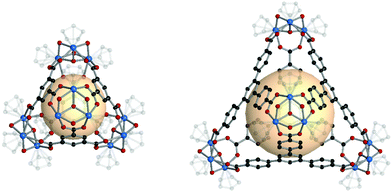 |
| Fig. 20 Structural illustration of ZrT-2, [Cp3Zr3O(OH)3]4(C1)4 (left) and ZrT-4, [Cp3Zr3O(OH)3]4(C7)4 (right).44 | |
[V6O6(OCH3)9(SO4)]4C4.
When the 3-c [V6O6(OCH3)9(SO4)] SBU was introduced to the tritopic organic ligands, C1 and C7, the POV-based MOPs, VMOP-14 ([V6O6(OCH3)9(SO4)]4(C1)4) and VMOP-15 ([V6O6(OCH3)9(SO4)]4(C7)4), could be synthesized with heterocuboidal geometries (Fig. 21).57 The molecular size of VMOP-15 was almost twice that of VMOP-14, due to the elongated tritopic linker, C7. Such isoreticular design for larger heterocubic cub-b-MOPs was utilized for selective adsorption of cationic dyes.84 VMOP-18 and VMOP-19 were crystallized and synthesized by the coordination-driven assembly of the [V6O6(OCH3)9X] cluster (X = VO4 for VMOP-18; SO4 for VMOP-19) and the tripotic 4,4′,4′′-(1,3,5-triazine-2,4,6-triyl)tribenzoate (C8) node (Fig. 21). Their packing arrangement could be described as a body-centered cubic packing type. VMOP-18 exhibited highly selective adsorption of cationic dyes over neutral and anionic dyes in aqueous solutions, demonstrating the potential uses of POV-based MOPs.
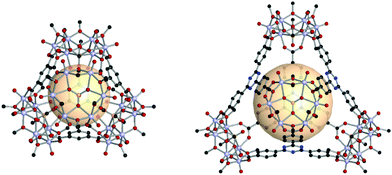 |
| Fig. 21 Structural illustration of VMOP-14, [V6O6(OCH3)9(SO4)]4(C1)4 (left)57 and VMOP-19, [V6O6(OCH3)9(SO4)]4(C8)4 (right).84 | |
[M4Cl(BTC4A)]4C4.
Liao et al. reported two heterecuboidal MOPs, [Co4Cl(BTC4A)]4(C5)4 and [Co4Cl(BTC4A)]4(C6)4, with calixarene-based SBUs.85 The BTC4A-based SBU does not have a suitable geometry for tetrahedron-shaped cage formation because of its 4-connectivity. However, the asymmetrical C5 linkers having one pyridyl and two carboxylate moieties produced heterocube MOPs. Each [Co4Cl(BTC4A)] was bridged by one pyridyl and two carboxylates from three different C5 linkers. A fluorinated C6 linker also formed the isostructural MOP. A vacant site in [Co4Cl(BTC4A)] was capped with formate.
4.5. Icosahedron (ico)
An icosahedron belonging to one of five regular solids has 20 triangular faces, 30 edges, and 12 vertices. The RCSR three-letter code is ico. Icosahedral geometry is also found in virus capsid systems.8 However, the artificial assembly of icosahedron-shaped coordination cages has been a daunting challenge due to the lack of accessible 5-c SBUs.22 Recently, pentagonal-coordinated metal clusters have become available.
[WV5O11(SO4)]12A30.
Zaworotko et al. addressed the 5-c SBU, which contains the WV5 polymetallic cluster, [WV5O11(SO4)].86 The edge-directed assembly with 30 A1 linkers yielded the elusive ico MOP, [WV5O11(SO4)]12(A1)30, for the first time (Fig. 22). They also reported the isoreticular synthesis of larger ico MOPs using A4 linkers, [WV5O11(SO4)]12(A4)30.
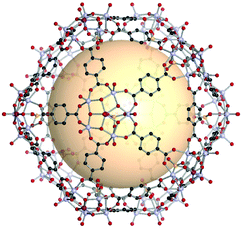 |
| Fig. 22 Structural illustration of [WV5O11SO4]12(A1)30.86 | |
5. Archimedean polyhedra
5.1. Cuboctahedron (cuo)
A cuboctahedron is a quasi-regular convex polyhedron composed of twelve vertices, twenty-four edges, and fourteen faces. More specifically, the faces consist of eight triangular faces and six square faces, each vertex is at the intersection between two triangular faces and two square faces, and each edge is at the intersection between a triangular face and a square face. The cuboctahedron is an Archimedean solid with octahedral symmetry (Oh). The RCSR letter code of a cuboctahedron is cuo with transitivity (1,1,2). The dual polyhedron of a cuboctahedron is a rhombic dodecahedron. To form a self-assembled cuboctahedron, twelve 4-c square planar SBUs at vertices and twenty-four bridging linkers between SBUs are needed. In this section, we introduce the cuboctahedral MOPs, assembled from twelve metal SBUs (M2) and twenty-four bent ditopic organic linkers (B) with 120° bond angles. Synthesized cuo-MOPs have the general formula of (M2)12B24.
(M2)12B24.
A general procedure for synthesizing cuo-MOPs is to assemble M2 SBUs and dicarboxylate linkers with 120° bond angles (Fig. 23). The nine paddlewheel M2 accessible thus far are Cu2, Mo2, Cr2, Ru2, Rh2, Ni2, Pd–Cu, Pd–Ni, and Pd–Zn. The linkers of cuo-MOPs are mainly 5-R-1,3-benzenecarboxylic acid (R = functional group placed at the 5-position of a linker, such as H, OH, and Br; abbreviated 5-R-mBDC). In addition, 2,7-naphthalenecarboxylic acid (H2B54) and 2,7-biphenylenedicarboxylic acid (H2B55) can be used as linkers for cuo-MOPs. Table 3 shows representative examples of cuo-MOPs reported.
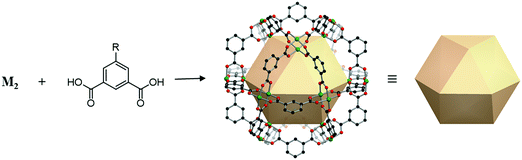 |
| Fig. 23 Schematic illustration of the general design of cuo-MOPs with M2 SBUs and 5-R-mBDC. M, green; C, black; O, red; all hydrogen atoms and solvents ligated on the metals are omitted for clarity. | |
Table 3 Examples of cuo-MOPs in the literature
Metal SBU |
Linker |
Ref. |
Metal SBU |
Linker |
Ref. |
Cu2 |
B14
|
11, 72 and 87–91
|
Cu2 |
B53
|
135
|
Cu2 |
B15
|
72, 88, 92–95, 97 and 99–108
|
Cu2 |
B54
|
109 and 136
|
Cu2 |
B16
|
88, 101 and 109–111
|
Cu2 |
B55
|
109
|
Cu2 |
B17
|
19, 72, 100, 101, 105, 108, 112–114 and 116
|
Cu2 |
B14, B15
|
72
|
Cu2 |
B18
|
109
|
Cu2 |
B20, B47
|
133
|
Cu2 |
B19
|
88
|
Cu2 |
B20, B54
|
72
|
Cu2 |
B20
|
20, 72, 99–101, 105, 108, 117 and 118
|
Cu2 |
B15, B33
|
137
|
Cu2 |
B21
|
88
|
Mo2 |
B14
|
73
|
Cu2 |
B22
|
115
|
Mo2 |
B15
|
73
|
Cu2 |
B23
|
115 and 119
|
Mo2 |
B20
|
73, 117 and 138
|
Cu2 |
B24
|
115 and 119
|
Cr2 |
B14
|
139
|
Cu2 |
B25
|
109
|
Cr2 |
B15
|
96
|
Cu2 |
B26
|
98, 120 and 121
|
Cr2 |
B20
|
96, 138 and 139
|
Cu2 |
B27
|
122
|
Cr2 |
B44
|
139
|
Cu2 |
B28
|
118 and 123
|
Ru2 |
B14
|
74
|
Cu2 |
B29
|
118
|
Ru2 |
B20
|
74 and 138
|
Cu2 |
B30
|
21
|
Rh2 |
B14
|
16 and 142
|
Cu2 |
B31
|
119
|
Rh2 |
B15
|
142
|
Cu2 |
B32
|
119
|
Rh2 |
B34
|
140 and 141
|
Cu2 |
B34
|
17, 118 and 124–127
|
Rh2 |
B35
|
141
|
Cu2 |
B40
|
128
|
Rh2 |
B36
|
143
|
Cu2 |
B42
|
129
|
Rh2 |
B37
|
143
|
Cu2 |
B43
|
129
|
Rh2 |
B38
|
142
|
Cu2 |
B44
|
130 and 131
|
Rh2 |
B39
|
142
|
Cu2 |
B45
|
132
|
Rh2 |
B40
|
143
|
Cu2 |
B46
|
132
|
Rh2 |
B41
|
143
|
Cu2 |
B48
|
133
|
Ni2 |
B15
|
76
|
Cu2 |
B49
|
134
|
Pd–Cu |
B20
|
144
|
Cu2 |
B50
|
122
|
Pd–Ni |
B20
|
144
|
Cu2 |
B51
|
135
|
Pd–Zn |
B20
|
144
|
Cu2 |
B52
|
135
|
|
|
|
(Cu2)12(B)24.
As shown in Table 3, most cuo-MOPs have been comprised of Cu2 SBUs. The Yaghi group synthesized a cuo-MOP using the Cu2 node and B14.11 The Zaworotko group also reported a hydroxylated Cu2-based cuo-MOP with B15.92 These hydroxylated cuo-MOPs have been used in applications such as a MOP precursor,72 in composites with other materials,97,106 or as supramolecular building blocks for MOFs.93,95 McManus et al. synthesized a sulfonated Cu2-based cuo-MOP with B17.112 Because this particular cuo-MOP has a negative charge due to the functional groups of linkers, it can interact with positively charged species. The resulting compounds have a 3D porous network113 and a 2D honeycomb architecture.19 Banerjee and coworkers introduced three cuo-MOPs with the linkers B22, B23, and B24.115 Because the linkers of cuo-MOPs are functionalized by alkoxy groups, the outer part of cuo-MOPs is more hydrophobic than the inner part of MOPs. When these cuo-MOPs were contacted with water, a hydrolytic conversion from MOP to MOF occurred, resulting in metal–organic nanosheets via self-exfoliation. Furukawa et al. synthesized a hydrophobic cuo-MOP with a dodecyloxy isophthalate linker, B34.124 Due to the long alkyl chains of the linker, this cuo-MOP is hydrophobic and assembled as a form of diamond packing. Hosono and Kitagawa groups introduced two kinds of routes for synthesizing MOP-core star polymers, also known as coordination star polymers (CSPs).129B42 and B43 linkers, both of them functionalized by dithiobenzoate and trithioester chain transfer groups, respectively, were used to realize several CSPs with cuo-MOPs as cores.
(Cu2)12(B1)n(B2)24−n.
The Zhou group synthesized cuo-MOPs with mixed linkers, the mixture of B14 and B15, and the mixture of B20 and B54.72 16 out of 24 B15 linkers were exchanged with B14 linkers via ligand substitution reaction in a solution of excess B14 and the precursor cuo-MOP with B15 by using the solubility of cuo-MOPs. A similar reaction, in a solution of excess B54 as a larger sized linker and the precursor cuo-MOP with B20, substituted 12 B54 linkers for 12 out of 24 B20. Park et al. synthesized a Cu2-based cuo-MOP with a mixture of B20 and B47.133 This particular cuo-MOP was introduced as a stimulus-responsive MOP due to an azobenzene moiety in B47. Lal et al. synthesized the cuo-MOP with a mixture of B15 with a hydrophilic moiety (–OH) and B33 with a hydrophobic moiety (–O(CH2)7CH3).137 These two linkers were segregated in cuo-MOP like Janus particles. Due to the segregation of hydrophilic and hydrophobic parts, micellization by clustering of cuo-MOPs was observed through transmission electron microscopy (TEM).
(Mo2)12(B)24.
An essential advantage of MOPs with the Mo2 node is that these MOPs can be studied in solution, unlike the Cu2 counterparts.73 The Zhou group synthesized a Mo2-based cuo-MOP with the B20 linker,117 together with diverse quadruply-bonded Mo2-based cuo-MOPs with B14, B15, and B20 linkers.73 Bloch et al. reported the optimized synthesis and activation of (Mo2)12(B20)24.138 This Mo2-based cuo-MOP showed permanent porosity with the highest Brunauer–Emmett–Teller (BET) surface area (1321 m2 g−1) among the reported MOPs. Compared to the synthesized iso-MOPs with Cr2 and Ru2 clusters, the BET surface area of (Mo2)12(B20)24 was higher.
(Cr2)12(B)24.
The Zhou group synthesized several Cr2-based cuo-MOPs with B14, B20, and B44 linkers.139 These Cr2-based cuo-MOPs showed substantially higher gas uptake and surface area compared with the Cu2 and Mo2-based analogues. The Bloch group also reported Cr2-based cuo-MOPs with B15 or B20 linkers,96 and the latter cuo-MOP showed a high surface area and excellent N2/O2 selectivity.
(Ru2)12(B)24.
In many Cu2cuo-MOPs, the potential reactivity at the metal sites is rather limited. The redox-active nature of dinuclear Ru2n+ species, however, is well-established with various oxidation states, ranging from tetra- to hexavalent. The Zhou group reported two Ru2-based cuo MOPs with B14 or B20 with a Ru2 redox-active core, while keeping their structural integrity.74
(Rh2)12(B)24.
The Furukawa group synthesized Rh2-based cuo-MOPs with B14.16 CO and NO molecules interacted strongly with Rh2 centers and were trapped in cuo-MOPs. The Furukawa group also synthesized a Rh2-based cuo MOP with B34.140 Kawano et al. synthesized Rh2-based cuo-MOPs with B34 or B35.141 Both functionalities placed at the 5-position of the linkers were long alkyl chains, and therefore, these MOPs exhibited good solubility in lipid bilayers through hydrophobic interactions. In general, Rh-based MOPs are robust with high thermal and chemical stability. Carné-Sánchez et al. further investigated the functionalization of Rh-based MOPs with B15. Through post-synthetic modification, hydroxyl groups of the MOP reacted with acyl anhydrides and chlorides to form MOPs with acetate ester and acrylate ester groups (B38 and B39).142 A recent work by Maspoch and coworkers showed a Rh2cuo-MOP with carboxylic acid groups at the 5-position of the linkers (B37).143 While the 1,3,5-benzenetricarboxylic acid (B37) linker often produced extended coordination networks, the protecting group (in B36) was employed and later removed for the synthesis of the MOP. Similar protection and removal were conducted to obtain the amino B16-based MOP from protected B41 linkers.
(Ni2)12(B)24.
A Ni2-based cuo-MOP can be assembled with the linker B15.76 It is well-established that the first-row transition metal cations can be easily incorporated into M2 SBUs. For example, the metal cations from Cr2+ to Zn2+ form MOFs with M2 SBUs. The effort to access cuo MOPs with a new combination of M2 SBUs possibly offers a new opportunity to control porosity, stability, and catalytic properties.
(M-Pd)12(B)24.
Sumby and Doonan groups reported cuo-MOPs, assembled from the B20 linker and heterobimetallic M–Pd, where M = Cu, Ni, or Zn.144 Interestingly, Pd(II) was predominantly localized at the inner nodes. These heterobimetallic MOPs exhibited exceptionally high H2 uptake and binding affinities for H2.
An isoreticular series of cuo-MOPs is possible, as shown in Fig. 24. As the size of the linker grows, the resulting cuo-MOPs get larger, while keeping the cuboctahedral geometry, as exemplified by MOP-23 and MOP-24 with the linkers B54 and B55, respectively.109
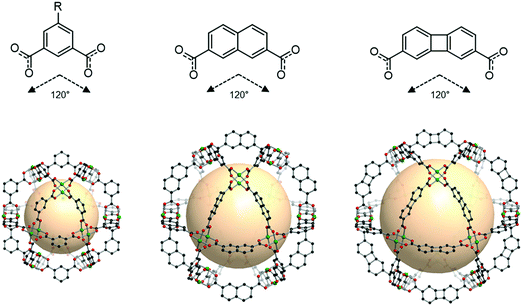 |
| Fig. 24 Chemical diagrams of 5-R-1,3-benzenedicarboxylate, B54, and B55 linkers, which have 120° bond angles. Structural illustration of cuo-MOPs resulting from the combination of M2 nodes and each linker.72,109 | |
6. Catalan polyhedra
6.1. Rhombic dodecahedron (rdo)
A rhombic dodecahedron, classified as a Catalan solid, is the dual of a cuboctahedron. Twelve rhombus faces form a rhombic dodecahedron. The transitivity is (2,1,1), and there are two types of vertices: six of the 4-c and eight of the 3-c nodes. The RCSR letter code of the rhombic dodecahedron is rdo. The combination of different 4-c and 3-c metal-cluster SBUs might be difficult in molecular polyhedron synthesis. In rdo-MOP assembly, metal-cluster SBUs are used as 4-c nodes, and the branching point of tritopic organic linkers is used as the 3-c node. Specifically, rdo-MOPs are assembled when six 4-c SBUs meet eight 3-c organic linkers.
[V4O8Cl]6C8 and [V5O9Cl]6C8.
Polyhedron-shaped molecular cages with POVs and organic ligands were reported by Zaworotko et al.145 Six shuttlecock-shaped POV clusters ([V4O8Cl] or [V5O9Cl]) as a 4-c node and eight tritopic carboxylate linkers, C1, formed small rhombic dodecahedral nanoballs. Different synthesis conditions and solvents afforded hyball-3, [V4O8Cl]6(C1)8, and hyball-4, [V5O9Cl]6(C1)8, with the same molecular octahedral geometries (Fig. 25). After exposure to the atmosphere for two weeks, the packing of hyball-3 was rearranged to hyball-3′ with additional hydrogen bonding around the [NH2Me2]+ cation. The resulting network was a doubly interpenetrated pcu net, which is another example of POV-based MOPs as supramolecular building blocks for the porous network. Hyball-4 was composed of pentanuclear [V5O9Cl] clusters, and a V5+ cation was located above four square pyramidal V4+ cations.
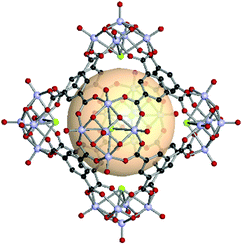 |
| Fig. 25 Structural illustration of hyball-4, [(V5O9Cl)6(C1)8].145 | |
[M4Cl(BTC4A)]6C8 and [M4O(TBSC)]6C8.
Attractive calixarene-based rhombic dodecahedral nanocages were assembled from [Co4(calix)(μ4-Cl)] (calix = BTC4A or deprotonated p-phenyl-thiacalix[4]arene (H4PTC4A)) as a 4-c node and a C1 (or C7) tritopic organic linker as a 3-c node.146 Four rdo coordination cages, CIAC-101 ([Co4(BTC4A)Cl]6(C1)8), CIAC-102 ([Co4(PTC4A)Cl]6(C1)8), CIAC-103 ([Co4(BTC4A)Cl]6(C7)8), and CIAC-104 ([Co4(PTC4A)Cl]6(C7)8), were created with different cage sizes (Fig. 26). The internal cavity sizes vary from 1.1 nm for CIAC-101 and -102 to 1.7 nm for CIAC-103 and -104. The tritopic linkers C1 and C7 capped the faces of the octahedron, and 4-c calixarene-based SBUs were located on the vertices of the octahedron. These MOPs can be assigned as rdo polyhedra with 4-c and 3-c nodes.
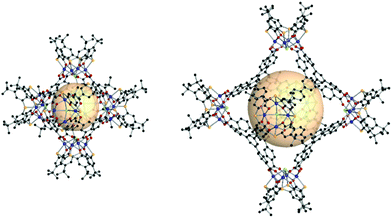 |
| Fig. 26 Structural illustration of CIAC-101 (left) and CIAC-103 (right), [Co4(BTC4A)Cl]6(C1)8 and [Co4(BTC4A)Cl]6(C7)8, respectively.146 | |
A similar calixarene-based 4-c SBU, [M4(TBSC)(μ4-H2O)], can be used for the assembly of rhombic dodecahedron-shaped MOPs with tritopic organic linkers. Wang et al. introduced a modular assembly of rdo coordination cages using various sulfonylcalix[4]arenes, metals, and organic linkers.147 MOSC-1-M (M = Ni, Co, Mg), a ([M4(TBSC)(μ4-H2O)]6(C1)8) series, was reported first. The modular aspect of the synthesis was proven to be successful as can be seen in MOSC-2-Ni ([Ni4(DTBSC)(μ4-H2O)]6(C1)8), de-p-tert-butylsulfonylcalix[4]arene (H4DTBSC), MOSC-3-Co ([M4(DTBSC)(μ4-H2O)]6(C2)8), and MOSC-4-Co ([M4(TBSC)(μ4-H2O)]6(C7)8). Liao et al. synthesized the two giant rdo coordination cages, CIAC-107 and CIAC-114, [Co4(TBSC)(OH)]6(C9)8148 and [Co4(TBSC)(OH)]6(C10)8,149 exhibiting an astonishing size of 5.0 nm and 5.4 nm, respectively. The reaction with [M4(TBSC)(μ4-H2O)] (M = Ni and Co) and the enantiopure C11 linker produced the chiral and catalytically active rdo-MOP, [M4(TBSC)(μ4-H2O)]6(C11)8.150 Three carboxylate moieties in the phosphoric acid-containing spiro ligand, C11, were bridged to the [M4(TBSC)(μ4-H2O)] SBU. Some of the dangling carboxylates, which do not make coordination bonding, formed intercage hydrogen bonding with phosphoric acid in the adjacent cage. Due to the asymmetrical linker shape, the rdo-MOP had an elongated molecular shape. The exposed phosphoric acid as the strong Brønsted acid groups acted as an efficient asymmetric catalyst.
(M2)6C8.
To form a rhombic dodecahedral MOP using an M2 paddlewheel SBU, the 3-c node component, i.e., the tritopic organic linkers, should be flexible and non-planar. The Lah group initiated the M2-based rdo-MOP assembly using the C16 3-c organic node.151 The rdo-MOP formula was (Cu2)6(C16)8 (Fig. 27). Meta-positioned carboxylates which formed coordination bonding with Cu2 were perpendicular to the central phenyl ring assigned as a 3-c node. The smallest-sized rdo-MOP was synthesized using a Cu2 SBU and C12.152 When the C12 linker had a syn–syn–syn conformation with the same carboxylate direction, the rdo-MOP could be formed. Liang et al. reported a rdo-MOP with Cu2 and the L-alanine-derived tritopic linker, C13.153 The synthesized (Cu2)6(C13)8 MOP had the same amide moiety in the organic linker as the (Cu2)6(C16)8 MOP, except for the size of the linkers. An example of nearly ideal rdo-shaped MOP (Cu2)6(C15)8 was reported.154 The molecular geometry of phenoxymethyl in the C15 linker is suitable to arrange nearly perpendicular to the central phenyl ring, preventing distortion of the rdo-MOP. A smaller tritopic linker (C14) was utilized by Huang et al. and a similar rdo MOP was reported.155
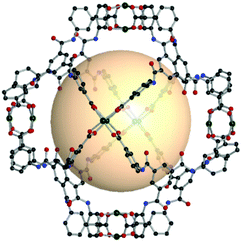 |
| Fig. 27 Structural illustration of [(Cu2)6(C16)8].151 | |
6.2. Rhombic triacontahedron (trc)
The rhombic triacontahedron is the most common thirty-faced polyhedron, containing thirty rhombus faces, sixty edges, and thirty-two vertices. The rhombic triacontahedron has the RCSR code trc. The trc-MOPs are comprised of two types of vertices, twelve 5-c SBUs and twenty 3-c organic nodes. Similar to the icosahedron, the 5-c SBU is exceptionally scarce. There is an example of an ideal trc-MOP.86 Another case is a trc-MOP with two missing organic nodes.156
[WV5O11(SO4)]12C20.
Ideal trc-MOP assemblies were formed by a combination of twelve 5-c [WV5O11(SO4)] SBUs and twenty tritopic linkers as the 3-c nodes, C1 and C8.86 The formula of assembled trc-MOPs was [WV5O11(SO4)]12(C1)20 and [WV5O11(SO4)]12(C8)20. The molecular size of [WV5O11(SO4)]12(C8)20 was about 4.3 nm (Fig. 28). Thirty-two node components are the largest number in MOP assemblies.
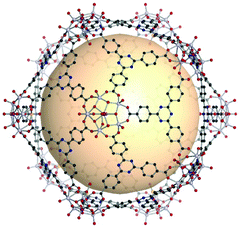 |
| Fig. 28 Structural illustration of [WV5O11(SO4)]12(C8)20.86 | |
[M4Cl(BTC4A)]12C18.
Zheng et al. reported a trc-MOP with two missing 3-c nodes formed from the asymmetric tritopic linker, C3.156 The SBU used, [M4Cl(BTC4A)], typically acts as a 4-c node, but with the asymmetric C3 linker, six of the [M4Cl(BTC4A)] SBUs act as 5-c nodes (Fig. 29). Interestingly, two of the cofacial 3-c nodes were missing in [M4Cl(BTC4A)]12(C3)18. Six of SBUs were 5-c nodes, and the other six SBUs near the defect sites became 4-c nodes.
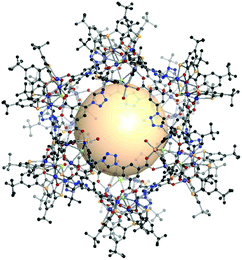 |
| Fig. 29 Structural illustration of [M4Cl(BTC4A)]12(C3)18.156 | |
7. Semi-regular polyhedra
7.1. Triangular prism (trp)
A triangular prism is composed of two triangles on the top and bottom faces and three rectangles on the side with D3h symmetry. The right prismatic polyhedra are semi-regular solids of (1,2,2) transitivity. The RCSR code is trp. There is only one example of a trp-MOP structure in the literature.
[Ni4O(TBSC)]6(B)6(B2)3.
Chen et al. reported the trp-MOP, [Ni4O(TBSC)]6(B58)12 (Fig. 30).157,158 The cluster [Ni4O(TBSC)] is generally known as a 4-c SBU. However, in the trp case, it became a 3-c node. The edge description was different, unlike other edge-transitive MOPs because doubly bridging B58 linkers corresponded to the three edges on the sides of the rectangle. The bent geometry of the thiophene of the B58 linker formed a triangular prismatic cage. Four carboxylates of the linkers formed coordination bonds with [Ni4O(TBSC)], but two of them on the side were considered to be one edge of the trp-MOP.
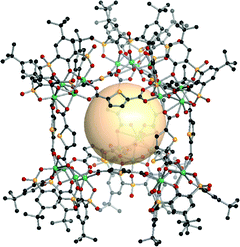 |
| Fig. 30 Structural illustration of [Ni4O(TBSC)]6(B58)12.158 | |
8. Other miscellaneous polyhedra
8.1. Anticuboctahedron (twc)
A anticuboctahedron is similar to the cuboctahedron but distinctly different. Imagine separating a cuboctahedron into two upper and lower parts (Fig. 31). Rotation of the lower part by 180°, followed by joining two parts, creates an anticuboctahedron. While a cuboctahedron belongs to an Archimedean solid, an anticuboctahedron is a Johnson solid, often referred as a triangular orthobicupola, J27. The RCSR code for an anticuboctahedron is twc with transitivity (2,4,3), named after a twinned cuboctahedron.
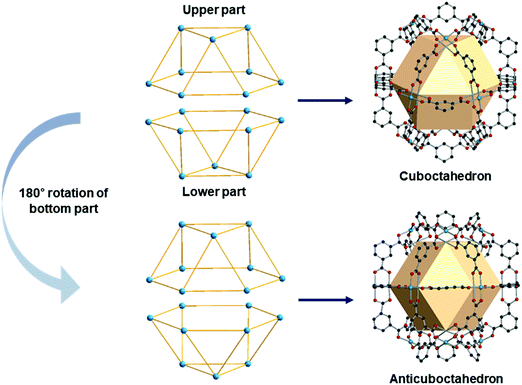 |
| Fig. 31 Schematic representation of the differences between a cuboctahedron and an anticuboctahedron. | |
[M2]12B24.
The twc MOP can be considered as a structural isomer of the cuo-MOP. The twc-MOP was synthesized from paddlewheel Cu2 units and B22 linkers.112 Apparently, the square face connectivity was different from that of the typical cuo-MOP. Another twc M2 MOP was synthesized from a Mo2 unit and the B14 linker.117 Zhou et al. also synthesized an extended twc-MOP cage using a larger bent-type linker (B54), (Mo2)12(B54)24.73 A Rh2-based anticuboctahedral MOP was reported by Kitagawa et al.16 The (Rh2)12(B14)24twc-MOP possessed a higher gas (N2 and CO) adsorption capacity compared to that of Cu2-based cuo-MOPs.
8.2. Hendecahedron (mtq-d)
A hendecahedron is a polyhedron with 11 faces, and numerous topologically different isomers are possible. In the molecular assembly, an odd number of faces and vertices is significantly rare. Surprisingly, Zhou et al. successfully demonstrated the assembly of metal–organic hendecahedra with an odd number of faces and vertices with mtq-d topology with transitivity (2,2,3).159 The dual of mtq topology is mtq-d.160
[M2]9(B′)6(B′′)12.
The construction of mtq-d MOPs was made by mixing two types of bent angle linkers, 90° and 120°, together with 4-c Cu2 SBUs.159 The linkers used were B4 (90°) and B56 (120°) and B57 (120°). The mtq-d-MOPs, (Cu2)9(B4)6(B56)12 and (Cu2)9(B4)6(B57)12, are comprised of six B4 linkers and twelve B56 or B57 linkers (Fig. 32). The twelve 120° linkers, B56 or B57, form polygons on the sides.
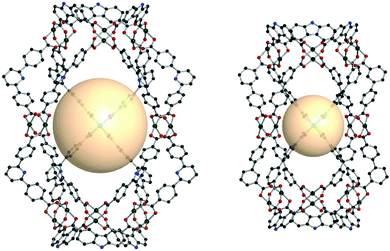 |
| Fig. 32 Structural illustration of (Cu2)9(B4)6(B56)12 (left) and (Cu2)9(B4)6(B57)12 (right).159 | |
8.3. Metal–organic Johnson deltahedra
A deltahedron is a polyhedron with equilateral triangular faces. There are eight convex deltahedra. The other five deltahedra belong to Jonson solids and they are called Johnson deltahedra, including triangular bipyramid (tbp), pentagonal bipyramid (ppi), snub disphenoid (bds), triaugmented triangular prism (tct), and gyroelongated square bipyramid (csa). Deltahedral geometries are well known in the main group and coordination chemistry, such as closo-carboranes and inorganic coordination complexes. Three types of geometries (bds, tct, csa) were assembled with new metal–organic Johnson deltahedra as their face-capped topologies (ghm, hmg, xum, respectively) by using the combination of 4-c and 5-c SBUs.161,162
8.3.1. Face-capped snub disphenoid (ghm).
The snub disphenoid (bds), one of the Johnson solids (J84), has 12 triangular faces and 8 vertices. It is a common geometry in coordination compounds, also known as “bisdisphenoid”. Wang et al. synthesized a face-capped snub disphenoid (ghm) type MOP, VMOP-22.161
[V5O9(OCH3)]4[MoV5O11(SO4)]4C12. VMOP-22 are composed of four 4-c SBUs ([V5O9(OCH3)]), four 5-c SBUs ([MoV5O11(SO4)]) and twelve C1 linkers. The overall formula of VMOP-22 is {[V5O9(OCH3)]4[MoV5O11(SO4)]4}(C1)12 (Fig. 33).
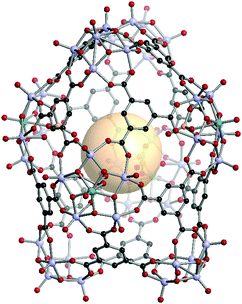 |
| Fig. 33 Structural illustration of VMOP-22, {[V5O9(OCH3)]4[MoV5O11(SO4)]4}(C1)12.161 | |
8.3.2. Face-capped triaugmented triangular prism (hmg).
The triaugmented triangular prism (tct) is one of the Johnson solids known as J51 and has fourteen triangular faces and nine vertices. It is a well-known coordination geometry as the “tricapped trigonal prismatic molecular geometry”, a common feature in nona-coordinating complexes. A MOP with face-capped triaugmented triangular prism (hmg) topology, VMOP-23, was synthesized by Wang et al.161
[V5O9Cl]3[MoV5O11(SO4)]6C14. Three 4-c [V5O9Cl] and six 5-c [MoV5O11(SO4)] clusters are located on the nine vertices of the tct polyhedron. Fourteen 3-c C1 linkers connected these SBUs to assemble hmg MOP, VMOP-23 (Fig. 34). The overall formula of VMOP-23 is {[V5O9Cl]3[MoV5O11(SO4)]6}(C1)14.
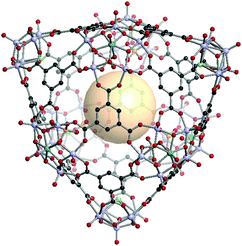 |
| Fig. 34 Structural illustration of VMOP-23, {[V5O9Cl]3[MoV5O11(SO4)]6}(C1)14.161 | |
8.3.3. Face-capped gyroelongated square bipyramid (xum).
A hexadecahedron with 16 faces has numerous topologically different isomers. Xu et al. and Hang et al. reported face-capped gyroelongated square bipyramidal MOPs.161,162 The arrangement of 10 SBUs in the vertices indicated the gyroelongated square bipyramid geometry, known as the Johnson-type solid J17 with csa topology, according to the RCSR code. However, the linker used to cap the 16 triangular faces was the tritopic C1 or C4, as a 3-c node. The topological code was xum from the RCSR. However, we could not find the exact name for this polyhedron.
[V5O8(CN)2]2[V6O11(SO4)]8C16. Two [V5O8(CN)2] SUBs as a 4-c node and eight [V6O11(SO4)] SBUs as a 5-c node are assembled with sixteen face-capping 3-c C1 organic linkers to form VMOP-24 with xum topology.161 The overall formula of VMOP-24 is {[V5O8(CN)2]2[V6O11(SO4)]8}(C1)16 (Fig. 35).
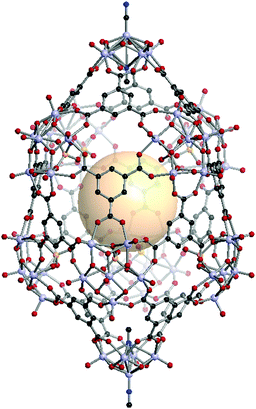 |
| Fig. 35 Structural illustration of VMOP-24, {[V5O9(CN)2]2[V6O11(SO4)]8}(C1)16.161 | |
{[M4(BTC4A)]10Cl6X4}C16 (X = CO3, HCOO, MeNCOO, or HC4). In this structure, there are three kinds of nodes.162 Two of them are [Ni4(BTC4A)] SBUs, utilized as 4-c or 5-c nodes, and the remaining one is C4, the 3-c asymmetric organic linker. The 4-c [Ni4Cl(BTC4A)] SBUs are located on top and bottom of the polyhedron, and four carboxylates of C4 are coordinated to the Ni4 cluster. The other eight [Ni4(BTC4A)] SBUs are nodes, but four of them have different coordination environments of the Ni4 cluster, compared to the chloride-coordinated [Ni4Cl(BTC4A)]. The different types of anions, denoted as X, are coordinated in a disordered fashion to the Ni4 cluster instead of Cl. The overall formula for xum MOP is {[M4(BTC4A)]10Cl6X4}(C4)16 (Fig. 36).
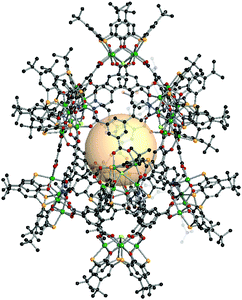 |
| Fig. 36 Structural illustration of {[M4(BTC4A)]10Cl6X4}(C4)16.162 | |
9. Notable polyhedra without metal-cluster SBUs
9.1. Coordination-driven self-assembly for Goldberg polyhedra
Some coordination cages built from Pd cations and pyridyl linkers are worthy of mention in this review, bridging the gap between MOPs and coordination cages. Perhaps MOF chemists can gain significant insights from these extraordinary cages made by Fujita and others. The cage with rdo topology, [Pd6(C24H21N3)8]12+, was prepared with six Pd atoms and eight tridentate ligands (C24H21N3 = 1,3,5-tris(4-pyridylmethyl)benzene) (Fig. 37).163 The Pd atoms are located at the vertices of the octahedron. Each Pd cations form a square planar geometry with four coordinated pyridyl groups of the ligands. Yaghi and coworkers reported Pd-based rdo cages, MOP-100 and MOP-101, composed of tetrakis(1-imidazolyl)borate and tetrakis(4-methyl-1-imidazolyl)borate linkers, respectively.164 Two cuo cages by Fujita and coworkers, [Pd12(C14OH10N2)24]24+ and [Pt12(C14OH10N2)24]24+, were synthesized with ditopic pyridyl ligands (C14OH10N2 = 2,5-bis(4-pyridyl)furan) (Fig. 37).165,166 By utilizing functionalized ligands, the inner or outer space of the cuo cage was tuned with various organic moieties.167 One of the interesting approaches showed the attachment of the other pyridyl ligand moieties in the precursor ligands, resulting in sphere-in-sphere complexes.168 When the ligand (C14SH10N2 = 2,5-bis(4-pyridyl)thiophene) with a larger bent angle was used, a larger coordination cage, [Pd24(C14SH10N2)48]48+ with rco (rhombicuboctahedron) geometry, was formed (Fig. 37).169 The next extension of the MnL2n series, M30L60 with the ido geometry, was prepared with a longer thiophene-cored bipyridyl linker, resulting in a giant cage with a size up to 8 nm (Fig. 37).170
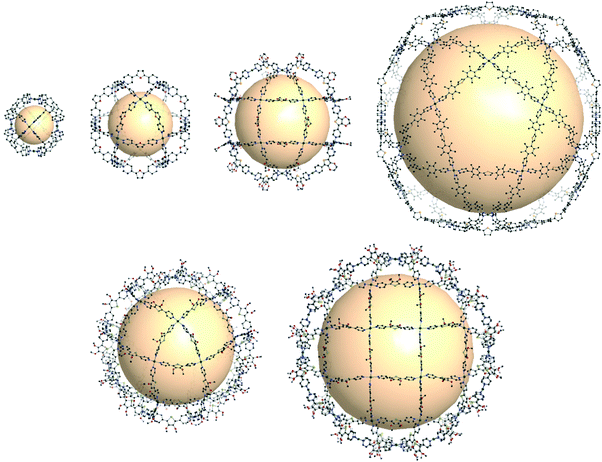 |
| Fig. 37 Structural illustration of coordination cages composed of Pd ions and bipyridyl linkers. Examples of rdo-, cuo-, rco-, and ido-type cages (first row). Cages with the formulas M30L60 and M48L96 corresponding to Goldberg polyhedra (second row).163–171 | |
Out of the regular and semi-regular polyhedra, Goldberg polyhedra composed of triangles and squares were synthesized, with the formulas M30L60 and M48L96, which had not been previously reported at the molecular level.171 To form M30L60 and M48L96 structures, a selenophene-cored bipyridyl linker with a slightly larger bend angle than that of the thiophene-cored linker was used, forming a rhombicuboctahedral cage (Fig. 37). With the same selenophene-cored linker, M48L96 was prepared. Self-assembly of giant polyhedra, from large numbers of small components, might be the state-of-the-art in supramolecular chemistry,170–172 which reminds us of the polyhedral protein complexes.173–175
9.2. Coordination cages with organic SBUs
Recently, intriguing calixarene assemblies were emerged with calix[n]arenes, acting as n-c nodes. On the other hand, these calix[n]arenes could be defined as a core with n number of 3-c nodes (center of the phenyl ring; two for adjacent phenyl, and one for the coordination center), unlike metal-cluster based SBUs which we have covered in this review.38
Some examples show coordination cages, including macrocyclic compounds such as pyrogallolarene and calixarene. The Atwood group reported an octahedral cage (Cu24(Pg)6, Pg = C-propan-3-ol pyrogallol[4]arene) (Fig. 38).176 In the structure, Pg SBUs were located at the vertices of the octahedron, and three copper ions formed coordination bonds with the linkers on each face of the octahedron. Considering the copper ions as building units with three connectivity, the cage can be interpreted as a rdo-type cage. Strictly speaking, the topology of the Cu24(Pg)6 cage is a rdo-derived tro (i.e. truncated octahedron), when Pg is considered to be a square with four 3-c nodes. With various metal ions, similar cages were synthesized, showing control over the coordination geometry of the metal ions.176–181
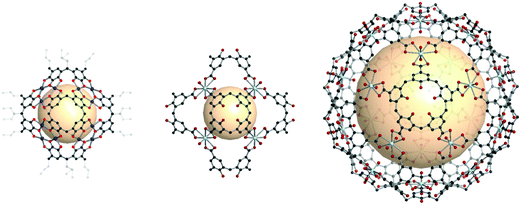 |
| Fig. 38 Structural illustration of coordination cages, including macrocyclic compounds. A rdo-derived tro-type cage synthesized with pyrogallol[4]arene linkers (left). Cages composed of calixarene linkers and uranyl ions showing rdo-derived tro and trc-derived deg topologies (middle and right).176,182 | |
Other macrocyclic compounds, calix[4]arene tetracarboxylate (CTC) and calix[5]arene pentacarboxylate (CPC), were employed to construct coordination cages with uranyl (UO22+) ions.182 In the case of CTC, a cage with rdo-derived tro topology, [(UO2)8(CTC)6]8−, was synthesized with six CTCs on the vertices and eight 3-c uranyl (UO2) nodes (Fig. 38). When CPC linkers were used, a combination of the linkers with five connectivity and uranyl nodes with three connectivity resulted in a cage with a trc-derived deg topology, [(UO2)20(CPC)12]20− (Fig. 38). What is striking here is the five-fold symmetry of CPC, making it possible to build a trc with 12 pentagon faces. Introducing the five-fold symmetry nodeis an important contribution because it can lead to exotic polyhedra (e.g., ico, ido) with five-fold symmetry.
Cotton et al. reported a tet cage assembled from four 3-c organic SBUs with 120° coordination bond angles and six inorganic linkers as edges (Fig. 39).183 Topologically, organic and inorganic building blocks are used as nodes and linkers, respectively, unlike typical MOPs assembled from inorganic nodes and organic linkers. The organic SBU, C1, was linked with 2-c Mo2 paddle wheel linkers, and adjacent carboxylate units were bridged. The 2-c Mo2 linker had a 90° linkage angle. Two non-bridging coordination sites on the Mo2 paddle wheel were capped by two N,N′-di-p-anisylfromamidinate (DAniF) anions. An isostructural tet cage can be made with the Rh2 node, in addition to the Mo2 dimer.184 The combination of M2 inorganic linkers and other well-designed organic SBUs might afford new polyhedral structures.185 After 20 years, Bloch et al. synthesized tet and cub cages based on 2-c Cu2 paddle wheel linkers and C1 as the organic SBU (Fig. 40).186 Cu paddle wheels in tet and cub cages were capped by two bipyridines (2,2′-bipyridine (bipy) for cub and 4,4′-dimethoxy bipy (OMe-bipy) for the tet cage). Indeed, there are more chances to make unique polyhedral architectures using a variation of the combination of SBU and linkers.
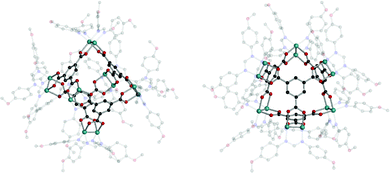 |
| Fig. 39 Structural illustrations of (C1)4[Mo2(DAniF)2]6.183 | |
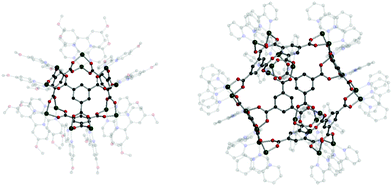 |
| Fig. 40 Structural illustration of (C1)4[Cu2(OMe-bipy)2]6 (left) and (C1)8[Cu2(bipy)2]12 (right).186 | |
10. Concluding remarks and future perspectives
After the first appearance of Cu-based MOP in 2001, the reticular design of MOPs, assisted by topological guidance, has been popular as exemplified by the MOPs with 14 topologies (including nine edge-transitive ones) highlighted in this review. In addition to such symmetrically pleasing topologies, rather limited applications are suggested for MOPs, unlike MOFs. The fact that MOPs are significantly under-explored probably stems from chemical stability and permanent porosity. Creating highly porous MOPs has been a challenge for MOP community. MOPs with over 1000 m2 g−1 of BET surface area are quite recent works, including a BET surface area of 1321 m2 g−1, the highest record for MOPs.138 Establishing porous MOPs demands elaborated understanding of both intrinsic (confined cavities) and extrinsic (interstitial voids) pores. Several recent works showed the higher BET surface area obtained from the control of the amorphization in different metal species.47,138,139 To create highly porous MOPs, a careful selection of chemically stable SBUs such as Zr-based nodes and robust organic linkers is mandatory, controlling intrinsic cavity stability and inter-cage interactions simultaneously. The use of oxophilic cations such as Zr and Cr cations often yields water-stable MOPs with permanent porosity. The discovery of water-stable SBUs with diverse coordination geometry is critical to promote the variety of MOPs.
A unique advantage of MOPs as porous adsorbents is not only the robustness of the desired SBU but also the adjustment of arrangement between the cages for the connection of extrinsic pores. Considering that most of the MOP crystals are held together by weak intermolecular interactions, the control of the packing of MOPs is still challenging to modulate their porous nature and chemical environment in exterior pores. Yuan et al. reported the enhancement of stability and porosity using the control of hydrogen bonding connectivity of Zr-based MOPs.187 Bloch et al. synthesized an ionic salt from the mixing of negatively charged cuo-MOP and positively charged Zr-based tet-MOP.188 The novel strategies to regulate interactions such as hydrogen bonding and ionic interactions will expand the number of porous MOP family.
Cage-shaped molecules are attractive because of their prominent properties, especially molecular recognition and encapsulation using the inner cavities. The inner pore in MOPs has great potential for new applications. MOPs can be considered as intermediate porous materials between MOFs and porous organic cages (POCs).189,190 Solution-processability of POCs and MOPs as the porous membrane was nicely demonstrated in the literature.118,191,192 From the diversity of SBUs and organic linkers, modular design is the unique strength of MOPs. These new MOPs allow us to explore an essential advantage of MOPs, solution processability, a feature highly desired for industrial applications.
MOFs are well established and widely used in highly active research fields encompassing broad areas of fundamental and materials sciences, while the research areas of MOPs are less explored compared to MOF counterparts. Recently, interesting examples of discrete cages of MOP have been reported for special applications, which are difficult to be accessed by MOFs.46,101,140,192–196 Further efforts for the discovery of new types of MOP are necessary to enrich the specific applications for MOP chemistry. The characteristics, functions, and potential challenges for MOFs, MOPs, and POCs are compared in Table 4.
Table 4 Selected characteristics and functions of MOFs, MOPs, and POCs
Characteristics |
MOFs |
MOPs |
POCs |
Porosity |
Microporous to mesoporous; up to ∼8000 m2 g−1 |
Non-porous or microporous; up to ∼1300 m2 g−1 |
Typically microporous; up to ∼3000 m2 g−1 |
Applications |
Highly applicable to areas such as catalysis, gas separation, bio/energy-related applications, to name a few |
Similar, but rather limited applications, compared to MOFs |
Particularly, important in molecular separation |
Diversity |
SBU and linker modulation has been well developed; multivariate MOFs; high structural diversity |
Only 14 types of polyhedra; limited choice of SBUs and linkers |
Cage type and chemical bonding could be modulated; limited types of cage shapes |
Chemical stability |
Poor to good; number of water-stable MOFs is rapidly growing |
Generally unstable in water; highly stable MOPs are exceptionally rare |
Generally unstable; stability could be enhanced by modification of chemical bonding |
Processability |
Insoluble in solvents |
Soluble in specific solvents for some MOPs; applicable to membrane/composites |
Soluble in specific solvents; advantageous for membrane applications |
Unique properties |
Control of structural and chemical properties; isoreticular approach; can be ultraporous |
Solution processability; isoreticular approach; can take advantages from the SBU and organic linker |
Solution processability; exhibiting polymorphism; possible to co-crystallize |
Potential challenges |
Commercialization; reproducible and large-scale applications |
Establishment of active research area; structural diversity; chemical stability and high permanent porosity |
Establishment of active research area; chemical stability |
The last point is the expansion of topological blueprints for MOPs, similar to coordination cages and MOFs. Only fourteen types of polyhedra were discovered in MOP assembly. This number is significantly lower than that of numerous topologies in over 80
000 MOF crystal structures. Several recent trends in MOPs could be identified in the review. One trend is the use of 5-c metal nodes, enabling us to build a new type of MOP like icosahedron. Inspired by numerous pyridyl-based cage compounds reported by Fujita et al., new linkers with the bent angle slightly higher than 120° could also be tested much more frequently in MOP synthesis. The most popular MOPs, cuo-MOPs, have 120° linkers capable of accommodating functional groups at 5-position without hindering the formation of MOPs. If one recalls that 120° is not the ideal angle for constructing a cuboctahedron, the angle variation in the choice of linkers for MOPs could also be interesting, which might narrow the gap between protein assembly and MOP assembly. We strongly believe that there is ample room for MOP chemists to create MOPs with giant cavities through extreme self-assembly.170–175
Conflicts of interest
There are no conflicts of interest to declare.
Acknowledgements
This work was supported by the National Research Foundation of Korea (NRF-2016R1A5A1009405 and 2020R1A2C3008226), Korea Environment Industry & Technology Institute (KEITI) through Public Technology Program based on Environmental Policy Program, funded by Korea Ministry of Environment (MOE) (2018000210002). We would like to thank Si Hyun Kim for his help with the visualization of polyhedra.
References
- S. Alvarez, Dalton Trans., 2005, 2209–2233 RSC
.
- B. Artmann, Math. Intell., 1993, 15, 52–53 CrossRef
.
- National Research Institute of Cultural Heritage, Sul, Korean Alcoholic Beverages, 2013, pp. 9–10.
-
F. M. D. Cornford, Plato's Cosmology: The Timaeus of Plato, Routledge, 2014 Search PubMed
.
- P. Barker and B. R. Goldstein, Osiris, 2001, 16, 88–113 CrossRef
.
- J.-P. Luminet, J. R. Weeks, A. Riazuelo, R. Lehoucq and J.-P. Uzan, Nature, 2003, 425, 593–595 CrossRef CAS
.
- H. W. Kroto, J. R. Heat, S. C. O’Brien, R. F. Curl and R. E. Smally, Nature, 1985, 318, 162–163 CrossRef CAS
.
- D. L. D. Casper and A. Klug, Cold Spring Harbor Symp. Quant. Biol., 1962, 27, 1–24 CrossRef
.
- D. Shechtman, I. Blech, D. Gratias and J. W. Cahn, Phys. Rev. Lett., 1984, 53, 1951–1953 CrossRef CAS
.
- Y. Sun and Y. Xia, Science, 2002, 298, 2176–2179 CrossRef CAS
.
- M. Eddaoudi, J. Kim, J. B. Wachter, H. K. Chae, M. O’Keeffe and O. M. Yaghi, J. Am. Chem. Soc., 2001, 123, 4368–4369 CrossRef CAS
.
- M. J. Kalmutzki, N. Hanikel and O. M. Yaghi, Sci. Adv., 2018, 4, eaat9180 CrossRef CAS
.
- J. Jiao, C. Tan, Z. Li, Y. Liu, X. Han and Y. Cui, J. Am. Chem. Soc., 2018, 140, 2251–2259 CrossRef CAS
.
- C. Tan, J. Jiao, Z. Li, Y. Liu, X. Han and Y. Cui, Angew. Chem., Int. Ed., 2018, 57, 2085–2090 CrossRef CAS
.
- A. C. Sudik, A. R. Millward, N. W. Ockwig, A. P. Côté, J. Kim and O. M. Yaghi, J. Am. Chem. Soc., 2005, 127, 7110–7118 CrossRef CAS
.
- S. Furukawa, N. Horike, M. Kondo, Y. Hijikata, A. Carné-Sánchez, P. Larpent, N. Louvain, S. Diring, H. Sato, R. Matsuda, R. Kawano and S. Kitagawa, Inorg. Chem., 2016, 55, 10843–10846 CrossRef CAS
.
- M. Jung, H. Kim, K. Baek and K. Kim, Angew. Chem., Int. Ed., 2008, 47, 5755–5757 CrossRef CAS
.
- A. Mallick, B. Garai, D. D. Díaz and R. Banerjee, Angew. Chem., Int. Ed., 2013, 52, 13755–13759 CrossRef CAS
.
- Y. Li, D. Zhang, F. Gai, X. Zhu, Y. Guo, T. Ma, Y. Liu and Q. Huo, Chem. Commun., 2012, 48, 7946–7948 RSC
.
- C. Zhao, N. Wang, L. Wang, H. Huang, R. Zhang, F. Yang, Y. Xie, S. Ji and J.-R. Li, Chem. Commun., 2014, 50, 13921–13923 RSC
.
- Y. N. Yun, M. Sohail, J.-H. Moon, T. W. Kim, K. M. Park, D. H. Chun, Y. C. Park, C.-H. Cho and H. Kim, Chem. – Asian J., 2018, 13, 631–635 CrossRef CAS
.
- D. J. Tranchemontagne, Z. Ni, M. O’Keeffe and O. M. Yaghi, Angew. Chem., Int. Ed., 2008, 47, 5136–5147 CrossRef CAS
.
- N. Hosono and S. Kitagawa, Acc. Chem. Res., 2018, 51, 2437–2446 CrossRef CAS
.
- J. J. Perry IV, J. A. Perman and M. J. Zaworotko, Chem. Soc. Rev., 2009, 38, 1400–1417 RSC
.
- V. Guillerm, D. Kim, J. F. Eubank, R. Luebke, X. Liu, K. Adil, M. S. Lah and M. Eddaoudi, Chem. Soc. Rev., 2014, 43, 6141–6172 RSC
.
- D. Kim, X. Liu and M. S. Lah, Inorg. Chem. Front., 2015, 2, 336–360 RSC
.
- M. Fujita, M. Tominaga, A. Hori and B. Therrien, Acc. Chem. Res., 2005, 38, 371–380 CrossRef
.
- K. Harris, D. Fujita and M. Fujita, Chem. Commun., 2013, 49, 6703–6712 RSC
.
- M. Yoshizawa, J. K. Klosterman and M. Fujita, Angew. Chem., Int. Ed., 2009, 48, 3418–3438 CrossRef CAS
.
- S. R. Seidel and P. J. Stang, Acc. Chem. Res., 2002, 35, 972–983 CrossRef CAS
.
- R. Chakrabarty, P. S. Mukherjee and P. J. Stang, Chem. Rev., 2011, 111, 6810–6918 CrossRef CAS
.
- D. L. Caulder and K. N. Raymond, Acc. Chem. Res., 1999, 32, 975–982 CrossRef CAS
.
- N. Ahmad, A. H. Chughtai, H. A. Younus and F. Verpoort, Coord. Chem. Rev., 2014, 280, 1–27 CrossRef CAS
.
- N. Ahmad, H. A. Younus, A. H. Chunghtai and F. Verpoort, Chem. Soc. Rev., 2015, 44, 9–25 RSC
.
- H. Vardhan, M. Yusubov and F. Verpoort, Coord. Chem. Rev., 2016, 306, 171–194 CrossRef CAS
.
- M. O’Keeffe, M. A. Peskov, S. T. Ramsden and O. M. Yaghi, Acc. Chem. Res., 2008, 41, 1782–1789 CrossRef
.
- Informally, the dual of a polyhedron is the polyhedron obtained by inserting new vertices in the old faces and joining them by new edges to vertices in contiguous faces22 (MOP review by Yaghi and O’Keeffe).
- C. Bonneau, M. O’Keeffe, D. M. Proserpio, V. A. Blatov, S. R. Batten, S. A. Bourne, M. S. Lah, J. Eon, S. T. Hyde, S. B. Wiggin and L. Öhrström, Cryst. Growth Des., 2018, 18, 3411–3418 CrossRef CAS
.
- C. Serre, F. Millange, S. Surblé and G. Férey, Angew. Chem., Int. Ed., 2004, 43, 6286–6289 CrossRef CAS
.
- G. Férey, C. Serre, C. Mellot-Draznieks, F. Millange, S. Surblé, J. Dutour and I. Margiolaki, Angew. Chem., Int. Ed., 2004, 43, 6296–6301 CrossRef
.
- G. Férey, C. Mellot-Draznieks, C. Serre, F. Millange, J. Dutour, S. Surblé and I. Margiolaki, Science, 2005, 309, 2040–2042 CrossRef
.
- O. M. Yaghi, M. O’Keeffe, N. W. Ockwig, H. K. Chae, M. Eddaoudi and J. Kim, Nature, 2003, 423, 705–714 CrossRef CAS
.
- J. H. Cavka, S. Jakobsen, U. Olsbye, N. Guillou, C. Lamberti, S. Bordiga and K. P. Lillerud, J. Am. Chem. Soc., 2008, 130, 13850–13851 CrossRef
.
- G. Liu, Z. Ju, D. Yuan and M. Hong, Inorg. Chem., 2013, 52, 13815–13817 CrossRef CAS
.
- G. Liu, M. Zeller, K. Su, J. Pang, Z. Ju, D. Yuan and M. Hong, Chem. – Eur. J., 2016, 22, 17345–17350 CrossRef CAS
.
- D. Nam, J. Huh, J. Lee, J. H. Kwak, H. Y. Jeong, K. Choi and W. Choe, Chem. Sci., 2017, 8, 7765–7771 RSC
.
- Z. Ju, G. Liu, Y. S. Chen, D. Yuan and B. Chen, Chem. – Eur. J., 2017, 23, 4774–4777 CrossRef CAS
.
- G. Liu, Y. Di Yuan, J. Wang, Y. Cheng, S. B. Peh, Y. Wang, Y. Qian, J. Dong, D. Yuan and D. Zhao, J. Am. Chem. Soc., 2018, 140, 6231–6234 CrossRef CAS
.
- S. Lee, J. H. Lee, J. C. Kim, S. Lee, S. K. Kwak and W. Choe, ACS Appl. Mater. Interfaces, 2018, 10, 8685–8691 CrossRef CAS
.
- W.-H. Xing, H.-Y. Li, X.-Y. Dong and S.-Q. Zang, J. Mater. Chem. A, 2018, 6, 7724–7730 RSC
.
- M. T. Pope and A. Müller, Angew. Chem., Int. Ed. Engl., 1991, 30, 34–48 CrossRef
.
- E. Coronado and C. J. Gómez-García, Chem. Rev., 1998, 98, 273–296 CrossRef CAS
.
- D.-L. Long, E. Burkholder and L. Cronin, Chem. Soc. Rev., 2007, 36, 105–121 RSC
.
- A. Dolbecq, E. Dumas, C. R. Mayer and P. Mialane, Chem. Rev., 2010, 110, 6009–6048 CrossRef CAS
.
- D. Du, J. Qin, S. Li, Z. Su and Y. Lan, Chem. Soc. Rev., 2014, 43, 4615–4632 RSC
.
- A. W. Augustynaiak, M. Fandzloch, M. Domingo, I. Łakomska and J. A. R. Navarro, Chem. Commun., 2015, 51, 14724–14727 RSC
.
- Y. Zhang, X. Wang, S. Li, Y. Gong, B. Song, K. Shao and Z. Su, Chem. Commun., 2016, 52, 9632–9635 RSC
.
- Y. Zhang, S. Li, X. Wang, Y. Gong, K. Shao and Z. Su, Dalton Trans., 2016, 45, 14898–14901 RSC
.
- Y. Gong, Y. Zhang, C. Qin, C. Sun, X. Wang and Z. Su, Angew. Chem., Int. Ed., 2019, 58, 780–784 CrossRef CAS
.
- A. K. Gupta, A. Yadav, A. K. Srivastava, K. R. Ramya, H. Paithankar, S. Nandi, J. Chugh and R. Boomishankar, Inorg. Chem., 2015, 54, 3196–3202 CrossRef CAS
.
- M. J. Byrnes, M. H. Chisholm and N. J. Patmore, Inorg. Chem., 2005, 44, 9347–9352 CrossRef CAS
.
- Y. Zhang, X. Wang, E. Zhou, X. Wu, B. Song, K. Shao and Z. Su, Dalton Trans., 2016, 45, 3698–3701 RSC
.
- Y. Zhang, X. Wang, S. Li, B. Song, K. Shao and Z. Su, Inorg. Chem., 2016, 55, 8770–8775 CrossRef CAS
.
-
J. Vicens and V. Böhmer, Calixarenes: A Versatile Class of Macrocyclic Compounds, Springer, 2012 Search PubMed
.
- R. M. McKinlay, G. W. V. Cave and J. L. Atwood, Proc. Natl. Acad. Sci. U. S. A., 2005, 102, 5944–5948 CrossRef CAS
.
- P. Jing, S. J. Dalgarno and J. L. Atwood, Coord. Chem. Rev., 2010, 254, 1760–1768 CrossRef
.
- P. P. Cholewa and S. J. Dalgarno, CrystEngComm, 2014, 16, 3655–3666 RSC
.
- K. Xiong, F. Jiang, Y. Gai, D. Yuan, L. Chen, M. Wu, K. Su and M. Hong, Chem. Sci., 2012, 3, 2321–2325 RSC
.
- F. Dai, U. Sambasivam, A. J. Hammerstrom and Z. Wang, J. Am. Chem. Soc., 2014, 136, 7480–7491 CrossRef CAS
.
- H. Tan, S. Du, Y. Bi and W. Liao, Inorg. Chem., 2014, 53, 7083–7085 CrossRef CAS
.
- M. Köberl, M. Cokoja, W. A. Herrmann and F. E. Kühn, Dalton Trans., 2011, 40, 6834–6859 RSC
.
- J. R. Li and H. C. Zhou, Nat. Chem., 2010, 2, 893–898 CrossRef CAS
.
- J. R. Li, A. A. Yakovenko, W. Lu, D. J. Timmons, W. Zhuang, D. Yuan and H. C. Zhou, J. Am. Chem. Soc., 2010, 132, 17599–17610 CrossRef CAS
.
- M. D. Young, Q. Zhang and H. C. Zhou, Inorg. Chim. Acta, 2015, 424, 216–220 CrossRef CAS
.
- C. A. Rowland, G. R. Lorzing, E. J. Gosselin, B. A. Trump, G. P. A. Yap, C. M. Brown and E. D. Bloch, J. Am. Chem. Soc., 2018, 140, 11153–11157 CrossRef CAS
.
- E. J. Gosselin, C. A. Rowland, K. P. Balto, G. P. A. Yap and E. D. Bloch, Inorg. Chem., 2018, 57, 11847–11850 CrossRef
.
- S. Ma, Y. Niu, X. Zhao and Z. Duan, Inorg. Chem. Commun., 2016, 70, 10–13 CrossRef CAS
.
- Z. Ni, A. Yassar, T. Antoun and O. M. Yaghi, J. Am. Chem. Soc., 2005, 127, 12752–12753 CrossRef CAS
.
- S. Mollick, S. Mukherjee, D. Kim, Z. Qiao, A. V. Desai, R. Saha, Y. D. More, J. Jiang, M. S. Lah and S. K. Ghosh, Angew. Chem., Int. Ed., 2019, 58, 1041–1045 CrossRef CAS
.
- S. A. Boer, K. F. White, B. Slater, A. J. Emerson, G. P. Knowles, W. A. Donald, A. W. Thornton, B. P. Ladewig, T. D. M. Bell, M. R. Hill, A. L. Chaffee, B. F. Abrahams and D. R. Turner, Chem. – Eur. J., 2019, 25, 8489–8493 CrossRef CAS
.
- N. Hosono, K. Omoto and S. Kitagawa, Chem. Commun., 2017, 53, 8180–8183 RSC
.
- S. T. Zhang, J. Zhang, X. X. Li, W. H. Fang and G. Y. Yang, J. Am. Chem. Soc., 2010, 132, 15102–15103 CrossRef
.
- Y. Bi, S. Wang, M. Liu, S. Du and W. Liao, Chem. Commun., 2013, 49, 6785–6787 RSC
.
- Y. Gong, W. Chen, L. Zhao, K. Shao, X. Wang and Z. Su, Dalton Trans., 2018, 47, 12979–12983 RSC
.
- X. Hang, S. Wang, X. Zhu, H. Han and W. Liao, CrystEngComm, 2016, 18, 4938–4943 RSC
.
- Y. Zhang, H. Gan, C. Qin, X. Wang, Z. Su and M. J. Zaworotko, J. Am. Chem. Soc., 2018, 140, 17365–17368 CrossRef CAS
.
- B. Moulton, J. Lu, A. Mondal and M. J. Zaworotko, Chem. Commun., 2001, 863–864 RSC
.
- T. H. Chen, L. Wang, J. V. Trueblood, V. H. Grassian and S. M. Cohen, J. Am. Chem. Soc., 2016, 138, 9646–9654 CrossRef CAS
.
- J. Yang, M. Lutz, A. Grzech, F. M. Mulder and T. J. Dingemans, CrystEngComm, 2014, 16, 5121–5127 RSC
.
- E. Amayuelas, A. Fidalgo-Marijuan, G. Barandika, B. Bazán, M. K. Urtiaga and M. I. Arriortua, CrystEngComm, 2015, 17, 3297–3304 RSC
.
- E. Amayuelas, A. Fidalgo-Marijuán, B. Bazán, M. K. Urtiaga, G. Barandika and M. I. Arriortua, CrystEngComm, 2016, 18, 1709–1712 RSC
.
- H. Abourahma, A. W. Coleman, B. Moulton, B. Rather, P. Shahgaldian and M. J. Zaworotko, Chem. Commun., 2001, 2380–2381 RSC
.
- J. Lee, J. Kwak and W. Choe, Nat. Commun., 2017, 8, 14070 CrossRef CAS
.
- R. W. Larsen, G. J. McManus, J. J. Perry IV, E. Rivera-Otero and M. J. Zaworotko, Inorg. Chem., 2007, 46, 5904–5910 CrossRef CAS
.
- H. Kim, M. Oh, D. Kim, J. Park, J. Seong, S. K. Kwak and M. S. Lah, Chem. Commun., 2015, 51, 3678–3681 RSC
.
- G. R. Lorzing, B. A. Trump, C. M. Brown and E. D. Bloch, Chem. Mater., 2017, 29, 8583–8587 CrossRef CAS
.
- R. Kumar and C. N. R. Rao, Dalton Trans., 2017, 46, 7998–8003 RSC
.
- A. Mallick, B. Garai, D. D. Díaz and R. Banerjee, Angew. Chem., Int. Ed., 2013, 52, 13755–13759 CrossRef CAS
.
- H. Huang, J.-R. Li, K. Wang, T. Han, M. Tong, L. Li, Y. Xie, Q. Yang, D. Liu and C. Zhong, Nat. Commun., 2015, 6, 8847 CrossRef CAS
.
- Y.-H. Kang, N. Yan, Z.-Y. Gao, P. Tan, Y. Jiang, X.-Q. Liu and L.-B. Sun, J. Mater. Chem. A, 2017, 5, 5278–5282 RSC
.
- Y. H. Kang, X. D. Liu, N. Yan, Y. Jiang, X. Q. Liu, L. B. Sun and J. R. Li, J. Am. Chem. Soc., 2016, 138, 6099–6102 CrossRef CAS
.
- R. W. Larsen, J. Am. Chem. Soc., 2008, 130, 11246–11247 CrossRef CAS
.
- C. M. Vetromile, A. Lozano, S. Feola and R. W. Larsen, Inorg. Chim. Acta, 2011, 378, 36–41 CrossRef CAS
.
- K. Mohomed, H. Abourahma, M. J. Zaworotko and J. P. Harmon, Chem. Commun., 2005, 3277–3279 RSC
.
- L.-B. Sun, J.-R. Li, W.-G. Lu, Z.-Y. Gu, Z.-P. Luo and H.-C. Zhou, J. Am. Chem. Soc., 2012, 134, 15923–15928 CrossRef CAS
.
- K. Mohomed, T. G. Gerasimov, H. Abourahma, M. J. Zaworotko and J. P. Harmon, Mater. Sci. Eng., A, 2005, 409, 227–233 CrossRef
.
- Z. Niu, S. Fang, X. Liu, J.-G. Ma, S. Ma and P. Cheng, J. Am. Chem. Soc., 2015, 137, 14873–14876 CrossRef CAS
.
- C. Zhao, N. Wang, L. Wang, S. Wang, H. Fan, F. Yang, S. Ji, J. R. Li and J. Yu, AIChE J., 2016, 62, 3706–3716 CrossRef CAS
.
- H. Furukawa, J. Kim, N. W. Ockwig, M. O’Keeffe and O. M. Yaghi, J. Am. Chem. Soc., 2008, 130, 11650–11661 CrossRef CAS
.
- H.-N. Wang, X. Meng, G.-S. Yang, X.-L. Wang, K. Z. Shao, Z.-M. Su and C.-G. Wang, Chem. Commun., 2011, 47, 7128–7130 RSC
.
- X. Liu, X. Wang, A. V. Bavykina, L. Chu, M. Shan, A. Sabetghadam, H. Miro, F. Kapteijn and J. Gascon, ACS Appl. Mater. Interfaces, 2018, 10, 21381–21389 CrossRef CAS
.
- G. J. McManus, Z. Wang and M. J. Zaworotko, Cryst. Growth Des., 2004, 4, 11–13 CrossRef CAS
.
- W. Wei, W. Li, X. Wang and J. He, Cryst. Growth Des., 2013, 13, 3843–3846 CrossRef CAS
.
- J. Ma, Y. Ying, Q. Yang, Y. Ban, H. Huang, X. Guo, Y. Xiao, D. Liu, Y. Li, W. Yang and C. Zhong, Chem. Commun., 2015, 51, 4249–4251 RSC
.
- B. Garai, A. Mallick, A. Das, R. Mukherjee and R. Banerjee, Chem. – Eur. J., 2017, 23, 7361–7366 CrossRef CAS
.
- C. R. P. Fulong, J. Liu, V. J. Pastore, H. Lin and T. R. Cook, Dalton Trans., 2018, 47, 7905–7915 RSC
.
- Y. Ke, D. J. Collins and H. C. Zhou, Inorg. Chem., 2005, 44, 4154–4156 CrossRef CAS
.
- M. Kitchin, J. Teo, K. Konstas, C. H. Lau, C. J. Sumby, A. W. Thornton, C. J. Doonan and M. R. Hill, J. Mater. Chem. A, 2015, 3, 15241–15247 RSC
.
- O. Barreda, G. Bannwart, G. P. A. Yap and E. D. Bloch, ACS Appl. Mater. Interfaces, 2018, 10, 11420–11424 CrossRef CAS
.
- D. Zhao, S. Tan, D. Yuan, W. Lu, Y. H. Rezenom, H. Jiang, L. Q. Wang and H. C. Zhou, Adv. Mater., 2011, 23, 90–93 CrossRef CAS
.
- N. Ahmad, H. A. Younus, A. H. Chughtai, K. V. Hecke, M. Danish, Z. Gaoke and F. Verpoort, Sci. Rep., 2017, 7, 832 CrossRef
.
- M. Tonigold, J. Hitzbleck, S. Bahnmüller, G. Langstein and D. Volkmer, Dalton Trans., 2009, 1363–1371 RSC
.
- M. Tonigold and D. Volkmer, Inorg. Chim. Acta, 2010, 363, 4220–4229 CrossRef CAS
.
- H. Furukawa, J. Kim, K. E. Plass and O. M. Yaghi, J. Am. Chem. Soc., 2006, 128, 8398–8399 CrossRef CAS
.
- E. V. Perez, K. J. Balkus, J. P. Ferraris and I. H. Musselman, J. Membr. Sci., 2014, 463, 82–93 CrossRef CAS
.
- J. J. Perry IV, V. C. Kravtsov, M. J. Zaworotko and R. W. Larsen, Cryst. Growth Des., 2011, 11, 3183–3189 CrossRef
.
- M.-S. Kim, J. Perry IV, T. C. M. Julien, E. Marangon, C. Manouat, J. F. Eubank and J. P. Harmon, J. Mater. Chem. A, 2015, 3, 13215–13225 RSC
.
- O. Barreda, G. A. Taggart, C. A. Rowland, G. P. A. Yap and E. D. Bloch, Chem. Mater., 2018, 30, 3975–3978 CrossRef CAS
.
- N. Hosono, M. Gochomori, R. Matsuda, H. Sato and S. Kitagawa, J. Am. Chem. Soc., 2016, 138, 6525–6531 CrossRef CAS
.
- D. Zhao, D. Q. Yuan, R. Krishna, J. M. van Baten and H. C. Zhou, Chem. Commun., 2010, 46, 7352–7354 RSC
.
- T.-F. Liu, Y.-P. Chen, A. A. Yakovenko and H.-C. Zhou, J. Am. Chem. Soc., 2012, 134, 17358–17361 CrossRef CAS
.
- K. Omoto, N. Hosono, M. Gochomori and S. Kitagawa, Chem. Commun., 2018, 54, 7290–7293 RSC
.
- J. Park, L. B. Sun, Y. P. Chen, Z. Perry and H. C. Zhou, Angew. Chem., Int. Ed., 2014, 53, 5842–5846 CrossRef CAS
.
- M. Zhang, W. Lu, J. R. Li, M. Bosch, Y. P. Chen, T. F. Liu, Y. Liu and H. C. Zhou, Inorg. Chem. Front., 2014, 1, 159–162 RSC
.
- J. Bae, K. Baek, D. Yuan, W. Kim, K. Kim, H. C. Zhou and J. Park, Chem. Commun., 2017, 53, 9250–9253 RSC
.
- H. Jung, D. Moon and H. Chun, Bull. Korean Chem. Soc., 2011, 32, 2489–2492 CrossRef CAS
.
- G. Lal, S. J. Lee, D. M. Spasyuk and G. K. H. Shimizu, Chem. Commun., 2018, 54, 1722–1725 RSC
.
- G. R. Lorzing, E. J. Gosselin, B. A. Trump, A. H. P. York, A. Sturluson, C. A. Rowland, G. P. A. Yap, C. M. Brown, C. M. Simon and E. D. Bloch, J. Am. Chem. Soc., 2019, 141, 12128–12138 CrossRef CAS
.
- J. Park, Z. Perry, Y. P. Chen, J. Bae and H. C. Zhou, ACS Appl. Mater. Interfaces, 2017, 9, 28064–28068 CrossRef CAS
.
- A. Carné-Sánchez, G. A. Craig, P. Larpent, T. Hirose, M. Higuchi, S. Kitagawa, K. Matsuda, K. Urayama and S. Furukawa, Nat. Commun., 2018, 9, 2506 CrossRef
.
- R. Kawano, N. Horike, Y. Hijikata, M. Kondo, A. Carné-Sánchez, P. Larpent, S. Ikemura, T. Osaki, K. Kamiya, S. Kitagawa, S. Takeuchi and S. Furukawa, Chem, 2017, 2, 393–403 CAS
.
- A. Carné-Sánchez, J. Albalad, T. Grancha, I. Imaz, J. Juanhuix, P. Larpent, S. Furukawa and D. Maspoch, J. Am. Chem. Soc., 2019, 141, 4094–4102 CrossRef
.
- J. Albalad, A. Carné-Sánchez, T. Grancha, L. Hernández-López and D. Maspoch, Chem. Commun., 2019, 55, 12785–12788 RSC
.
- J. M. Teo, C. J. Coghlan, J. D. Evans, E. Tsivion, M. Head-Gordon, C. J. Sumby and C. J. Doonan, Chem. Commun., 2016, 52, 276–279 RSC
.
- Z. Zhang, L. Wojtas and M. J. Zaworotko, Chem. Sci., 2014, 5, 927–931 RSC
.
- M. Liu, W. Liao, C. Hu, S. Du and H. Zhang, Angew. Chem., Int. Ed., 2012, 51, 1585–1588 CrossRef CAS
.
- F.-R. Dai and Z. Wang, J. Am. Chem. Soc., 2012, 134, 8002–8005 CrossRef CAS
.
- S. Du, C. Hu, J.-C. Xiao, H. Tan and W. Liao, Chem. Commun., 2012, 48, 9177–9179 RSC
.
- S. Du, T.-Q. Yu, W. Liao and C. Hu, Dalton Trans., 2015, 44, 14394–14402 RSC
.
- W. Gong, D. Chu, H. Jiang, X. Chen, Y. Cui and Y. Liu, Nat. Commun., 2019, 10, 600 CrossRef CAS
.
- M. J. Prakash, Y. Zou, S. Hong, M. Park, M. N. Bui, G. H. Seong and M. S. Lah, Inorg. Chem., 2009, 48, 1281–1283 CrossRef CAS
.
- M. Paul, N. N. Adarsh and P. Dastidar, Cryst. Growth Des., 2014, 14, 1331–1337 CrossRef CAS
.
- Z. Chen, X. Liu, A. Wu, Y. Liang, X. Wang and F. Liang, RSC Adv., 2016, 6, 9911–9915 RSC
.
- Y.-C. He, J. Yang, W.-Q. Kan and J.-F. Ma, CrystEngComm, 2013, 15, 848–851 RSC
.
- J. Huang, L. Liu, Y. Yang, Y. Li, L. Wang, S. Xiang, Z. Yao and Z. Zhang, Chem. Commun., 2019, 108, 107540 CAS
.
- D. Geng, X. Han, Y. Bi, Y. Qin, Q. Li, L. Huang, K. Zhou, L. Song and Z. Zheng, Chem. Sci., 2018, 9, 8535–8541 RSC
.
- It is difficult to accept that the trp MOP is designed from building units since only one type of organic linker was used for the preparation of a polyhedron of transitivity (1,2,2).
- S. Wang, X. Gao, X. Hang, X. Zhu, H. Han, W. Liao and W. Chen, J. Am. Chem. Soc., 2016, 138, 16236–16239 CrossRef CAS
.
- J.-R. Li and H.-C. Zhou, Angew. Chem., Int. Ed., 2009, 48, 8465–8468 CrossRef CAS
.
- O. Delgado-Friendrichs and M. O’Keeffe, Acta Crystallogr., Sect. A: Found. Adv., 2017, 73, 227–230 CrossRef
.
- N. Xu, H. Gan, C. Qin, X. Wang and Z. Su, Angew. Chem., Int. Ed., 2019, 58, 4649–4653 CrossRef CAS
.
- X. Hang, B. Liu, X. Zhu, S. Wang, H. Han, W. Liao, Y. Liu and C. Hu, J. Am. Chem. Soc., 2016, 138, 2969–2972 CrossRef CAS
.
- D. K. Chand, K. Biradha, M. Fujita, S. Sakamoto and K. Yamaguchi, Chem. Commun., 2002, 2486–2487 RSC
.
- Z. Lu, C. B. Knobler, H. Furukawa, B. Wang, G. Liu and O. M. Yaghi, J. Am. Chem. Soc., 2009, 131, 12532–12533 CrossRef CAS
.
- M. Tominaga, K. Suzuki, M. Kawano, T. Kusukawa, T. Ozeki, S. Sakamoto, K. Yamaguchi and M. Fujita, Angew. Chem., Int. Ed., 2004, 43, 5621–5625 CrossRef CAS
.
- D. Fujita, A. Takahashi, S. Sato and M. Fujita, J. Am. Chem. Soc., 2011, 133, 13317–13319 CrossRef CAS
.
- K. Harris, D. Fujita and M. Fujita, Chem. Commun., 2013, 49, 6703–6712 RSC
.
- Q. F. Sun, T. Murase, S. Sato and M. Fujita, Angew. Chem., Int. Ed., 2011, 50, 10318–10321 CrossRef CAS
.
- Q.-F. Sun, J. Iwasa, D. Ogawa, Y. Ishido, S. Sato, T. Ozeki, Y. Sei, K. Yamaguchi and M. Fujita, Science, 2010, 328, 1144–1147 CrossRef CAS
.
- D. Fujita, Y. Ueda, S. Sato, H. Yokoyama, N. Mizuno, T. Kumasaka and M. Fujita, Chem, 2016, 1, 91–101 CAS
.
- D. Fujita, Y. Ueda, S. Sato, N. Mizuno, T. Kumasaka and M. Fujita, Nature, 2016, 540, 563–566 CrossRef CAS
.
- P. Li, N. A. Vermeulen, C. D. Malliakas, D. A. Gómez-Gualdrón, A. J. Howarth, B. L. Mehdi, A. Dohnalkova, N. D. Browning, M. O’Keeffe and O. K. Farha, Science, 2017, 356, 624–627 CrossRef CAS
.
- P.-S. Huang, S. E. Boyken and D. Baker, Nature, 2016, 537, 320–327 CrossRef CAS
.
- Y. Hsia, J. B. Bale, S. Gonen, D. Shi, W. Sheffler, K. K. Fong, U. Nattermann, C. Xu, P.-S. Huang, R. Ravichandran, S. Yi, T. N. Davis, T. Gonen, N. P. King and D. Baker, Nature, 2016, 535, 136–139 CrossRef CAS
.
- J. B. Bale, S. Gonen, Y. Liu, W. Sheffler, D. Ellis, C. Thomas, D. Cascio, T. O. Yeates, T. Gonen, N. P. King and D. Baker, Science, 2016, 353, 389–394 CrossRef CAS
.
- R. M. McKinlay, G. W. V. Cave and J. L. Atwood, Proc. Natl. Acad. Sci. U. S. A., 2005, 102, 5944–5948 CrossRef CAS
.
- H. Kumari, A. V. Mossine, S. R. Kline, C. L. Dennis, D. A. Fowler, S. J. Teat, C. L. Barnes, C. A. Deakyne and J. L. Atwood, Angew. Chem., Int. Ed., 2012, 51, 1452–1454 CrossRef CAS
.
- A. S. Rathnayake, K. A. Feaster, J. White, C. L. Barnes, S. J. Teat and J. L. Atwood, Cryst. Growth Des., 2016, 16, 3562–3564 CrossRef CAS
.
- C. Zhang, R. S. Patil, C. Liu, C. L. Barnes and J. L. Atwood, J. Am. Chem. Soc., 2017, 139, 2920–2923 CrossRef CAS
.
- C. Zhang, R. S. Patil, T. Li, C. L. Barnes and J. L. Atwood, Chem. Commun., 2017, 53, 4312–4314 RSC
.
- K. Su, M. Wu, D. Yuan and M. Hong, Nat. Commun., 2018, 9, 4941 CrossRef
.
- S. Pasquale, S. Sattin, E. C. Escudero-Adán, M. Martínez-Belmonte and J. De Mendoza, Nat. Commun., 2012, 3, 785 CrossRef
.
- F. A. Cotton, L. M. Daniels, C. Lin and C. A. Murillo, Chem. Commun., 1999, 841–842 RSC
.
- F. A. Cotton, C. Lin and C. A. Murillo, Inorg. Chem., 2001, 40, 6413–6417 CrossRef CAS
.
- F. A. Cotton, P. Lei, C. Lin, C. A. Murillo, X. Wang, S.-Y. Yu and Z.-X. Zhang, J. Am. Chem. Soc., 2004, 126, 1518–1525 CrossRef CAS
.
- G. R. Lorzing, E. J. Gosselin, B. S. Lindner, R. Bhattacharjee, G. P. A. Yap, S. Caratzoulas and E. D. Bloch, Chem. Commun., 2019, 55, 9527–9530 RSC
.
- M. Zhou, G. Liu, Z. Ju, K. Su, S. Du, Y. Tan and D. Yuan, Cryst. Growth Des., 2020, 20, 4127–4134 CrossRef CAS
.
- E. J. Gosselin, G. E. Decker, A. M. Antonio, G. R. Lorzing, G. P. A. Yap and E. D. Bloch, J. Am. Chem. Soc., 2020, 142, 9594–9598 CrossRef
.
- T. Tozawa, J. T. A. Jones, S. I. Swamy, S. Jiang, D. J. Adams, S. Shakespeare, R. Clowes, D. Bradshaw, T. Hasell, S. Y. Chong, C. Tang, S. Thompson, J. Parker, A. Trewin, J. Bacsa, A. M. Z. Slawin, A. Steiner and A. I. Cooper, Nat. Mater., 2009, 8, 973–978 CrossRef CAS
.
- T. Hasell and A. I. Cooper, Nat. Rev. Mater., 2016, 1, 1–14 Search PubMed
.
- Q. Song, S. Jiang, T. Hasell, M. Liu, S. Sun, A. K. Cheetham, E. Sivaniah and A. I. Cooper, Adv. Mater., 2016, 28, 2629–2637 CrossRef CAS
.
- J. Liu, W. Duan, J. Song, X. Guo, Z. Wang, X. Shi, J. Liang, J. Wang, P. Cheng, Y. Chen, M. J. Zaworotko and Z. Zhang, J. Am. Chem. Soc., 2019, 141, 12064–12070 CrossRef CAS
.
- J. Lee, D.-W. Lim, S. Dekura, H. Kitagawa and W. Choe, ACS Appl. Mater. Interfaces, 2019, 11, 12639–12646 CrossRef CAS
.
- Y. Fang, J. Li, T. Togo, F. Jin, Z. Xiao, L. Liu, H. Drake, X. Lian and H.-C. Zhou, Chem, 2018, 4, 555–563 CAS
.
- T. Grancha, A. Carné-Sánchez, L. Hernández-López, J. Albalad, I. Imaz, J. Juanhuix and D. Maspoch, J. Am. Chem. Soc., 2019, 141, 18349–18355 CrossRef CAS
.
- S. Mollick, S. Fajal, S. Saurabh, D. Mahato and S. K. Ghosh, ACS Cent. Sci., 2020, 6, 1534–1541 CrossRef CAS
.
|
This journal is © The Royal Society of Chemistry 2021 |
Click here to see how this site uses Cookies. View our privacy policy here.