DOI:
10.1039/D0CS00676A
(Review Article)
Chem. Soc. Rev., 2021,
50, 667-690
Recent advances in luminescent materials for super-resolution imaging via stimulated emission depletion nanoscopy†
Received
14th August 2020
First published on 14th December 2020
Abstract
Stimulated emission depletion (STED) nanoscopy is a promising fluorescence microscopy to detect unresolvable structures at the nanoscale level and then achieve a superior imaging resolution in materials science and biological research. However, in addition to the optimization of the microscope, luminescent materials in STED nanoscopy are also of great significance to obtain imaging, visualization and even long-term tracking at an ultra-high resolution (less than 100 nm), but this is seldom summarized. Based on this consideration, recent progress on STED fluorophores for super-resolution imaging is outlined here, including inorganic fluorophores, fluorescent proteins, organic luminescent materials, aggregation-induced emission (AIE) luminogens, and fluorescent nanoparticles. Characteristics of these aforementioned STED fluorophores are also included and compared to provide a deep understanding of the relationship between the properties in luminescent materials and their performance in STED imaging. According to the results on such luminescent materials, it is anticipated that guidelines to select proper probes and even develop new materials for super-resolution imaging via STED nanoscopy will be provided here, finally promoting the development of super-resolution imaging in both materials science and biological research.
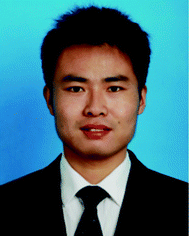
Dongfeng Dang
| Dongfeng Dang is an associated professor at school of chemistry in Xi’an Jiaotong University. He received his PhD degree in 2015 from Xiangtan University. From 2012 to 2013, he was a visiting PhD student at Chalmers University of Technology. In 2015, he was appointed as an assistant professor at Xi’an Jiaotong University and then became an associate professor in 2018. During this period, he was a postdoc and visiting scholar at The Hong Kong University of Science and Technology. His current research interest is mainly focused on functional materials for organic electronics and biomedical engineering. |
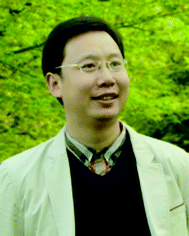
Lingjie Meng
| Lingjie Meng is a professor at school of chemistry in Xi’an Jiaotong University. He received his PhD degree from Xi’an Jiaotong University in 2005 and was a lecturer in Shanghai Jiaotong University from 2005 to 2010. Then, he became an associate professor in 2011. He was a visiting scholar at the University of Texas at Austin from 2011 to 2012. In 2013, he was appointed as a Professor in Xi’an Jiaotong University. His research interest is focused on the nanomaterials for theranostics. |
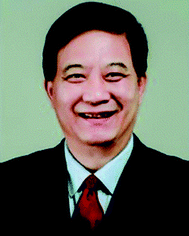
Ben Zhong Tang
| Ben Zhong Tang is the Stephen K. C. Cheong Professor of Science, Chair Professor of Chemistry, and Chair Professor of Chemical and Biological Engineering at the Hong Kong University of Science & Technology (HKUST). He received BS and PhD degrees from South China University of Technology and Kyoto University, respectively, and then conducted postdoctoral research at the University of Toronto. He joined HKUST as an assistant professor in 1994 and was promoted to a chair professor in 2008. His research interests include macromolecular chemistry, materials science, and biomedical theranostics. His lab is spearheading research on aggregation-induced emission (AIE). |
1. Introduction
Over the past few decades, optical fluorescence microscopy1–4 has become one of the most influential and powerful microscopies in life and materials science due to its advantages of high sensitivity, in situ workability, and noninvasiveness. For instance, by using a focused light beam to avoid light from the neighboring planes, confocal laser scanning microscopy (CLSM)5–8 reduces the background noise efficiently, thus significantly enhancing the performance of biological imaging. In another case, multiphoton microscopy9–12 with a long excitation wavelength provides the possibility to image at deeper penetration depths, thus achieving a clear imaging of tissues. However, although significant progress has been achieved on such optical microscopy techniques, it is hard to distinguish two objects closer than half the wavelength (λ) in a target objective according to Abbe's diffraction limit.13,14 Thus, in contrast to electron microscopy techniques, such as scanning electron microscopy (SEM), and transmission electron microscopy (TEM),15 a spatial resolution of about 200 nm can only be acquired in this case. This is a limitation for several applications. Therefore, to break the diffraction limit and narrow the resolution gap between optical and electron microscopy to image detailed structures and achieve dynamic process monitoring, super-resolution microscopy16–19 is urgently needed.
Currently, super-resolution microscopy can be categorized into two groups: (1) stochastic super-resolution microscopy and (2) non-stochastic super-resolution microscopy to reduce the size of the point-spread function (PSF).20,21 In the first case, photo-activated localization microscopy (PALM)22,23 and stochastic optical reconstruction microscopy (STORM)24–26 have recently triggered the attention of several scientists. To overcome the diffraction barrier and thus obtain precise localization, fluorophores that can undergo “off–on–off” luminescence switching are of great significance in PALM and STORM to emit randomly per area. Moreover, super-resolution optical fluctuation imaging (SOFI)27–29 exhibits a faster rate to undergo super-resolution reconstruction due to the requirements for blinking and labeling density. However, although a superior resolution can be obtained by using these techniques, thousands of images are usually needed to obtain a super-resolved image. This implies a large demand of advanced image analysis techniques. In addition, practical issues, such as the overlapping of emitters in a single emission spot or over-counting during the reconstruction process, always limit their applications in improving the imaging resolution. Interestingly, the second super-resolution category, such as structured illumination microscopy (SIM)30 and stimulated emission depletion (STED) nanoscopy,13,31–34 provides a strategy to overcome the limits of stochastic super-resolution microscopy. In the case of SIM, the targeted samples are illuminated with spatially structured excitation light,30 which can significantly enhance the imaging resolution by forming Moire fringes. This method was also well applied in biological research, such as live-cell imaging at high resolution.35 In STED nanoscopy, a donut-shaped depletion beam is employed to reduce the emission area. Only the fluorophores in the donut center can emit and be detected, thus resulting in a significant enhancement of the imaging resolution. It is noted that although there is no single super-resolution method that can produce an optimal performance, STED nanoscopy is a promising strategy to detect unresolvable structures at the nanoscale level and achieve a superior imaging resolution in biological and materials research.33,36–38
To obtain super-resolution imaging via STED nanoscopy, fluorophores as STED imaging agents, including inorganic quantum dots (QDs),39,40 up-conversion nanoparticles (UCNPs),41,42 fluorescent proteins,43,44 organic luminescent materials (OLMs),45,46 aggregation-induced emission (AIE) luminogens,47,48 and their corresponding fluorescent nanoparticles,49,50 are of great significance. The resolutions in STED nanoscopy by using various fluorophores are also listed in Table S1 (ESI†). Furthermore, a deep understanding of the photo-physical properties of these imaging agents in STED nanoscopy is not only essential to optimize the imaging performance, but also important to develop new probes to meet the STED requirements. However, despite the great roles and potential of luminescent materials in STED nanoscopy, unfortunately, only a few reviews are reported in this area. Based on this consideration, the recent progress on STED fluorophores in super-resolution imaging is summarized here. The outline here is listed as the following: (1) working mechanism of STED nanoscopy; (2) material requirements in STED nanoscopy; (3) luminescent materials for STED imaging, including inorganic luminescent materials, conventional organic dyes, aggregation-induced emission (AIE) luminogens and fluorescent nanoparticles; (4) conclusions and prospects. The goal of this review is to provide the readers with a description of the recent advances in luminescent materials in STED nanoscopy and of the rules to select proper probes when using STED nanoscopy in biological and material research. This review also wants to provide some guidelines to develop new materials for super-resolution imaging via STED nanoscopy.
2. Working mechanism of STED nanoscopy
To better understand the recent developments in luminescent materials in STED nanoscopy for super-resolution imaging, the working mechanism of STED nanoscopy should be firstly introduced. Then, its potential limitations should also be explained, thus providing the design rules for the imaging materials to well meet the STED requirements, finally leading to a superior imaging resolution.
In STED nanoscopy, in addition to the excitation laser, a doughnut-shaped depletion beam, as the second laser, plays an important role in reducing the size of PSF,51–53 thereby providing a superior imaging resolution. This means that when the fluorophore in STED nanoscopy is only excited by the excitation laser, a normal fluorescence process occurs in the fluorophore from its electronic ground state (S0) to excited singlet state (S1). Then, a fluorescence emission via an initial vibrational relaxation process follows. However, when the STED beam is “on”, the excited fluorophore (S1) can be quenched efficiently through stimulated emission depletion, thus leading to a size reduction in emission area caused by the doughnut-shaped beam, finally providing an enhanced imaging resolution (Fig. 1).18,54,55 It is worth noting that both the wavelength and intensity of the depletion beam in STED nanoscopy are important to achieve a high depletion efficiency in this super-resolution technique. Generally, the wavelength of the STED beam is located in the low-lying region relative to the maximum of its emission spectrum to avoid interferences in the signal detection. On the other hand, by using a high intensity STED beam, an excited singlet state in the fluorophores can be depleted efficiently before the fluorescence process occurs, thus leading to a significant enhancement in the resolution of STED nanoscopy (Fig. 1D).34
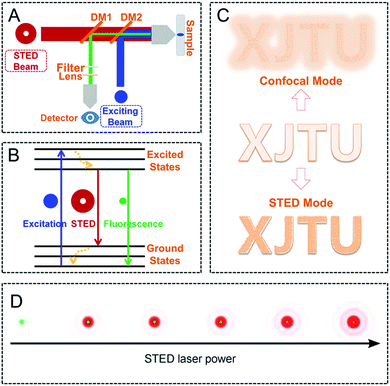 |
| Fig. 1 Schematic diagram of the setup (A) and the mechanism (B) of STED nanoscopy. (C) Schematic illustration of the performance in imaging resolution by confocal microscopy and STED nanoscopy. (D) Reduction of the lateral PSF size by using a doughnut-shaped STED beam (red) when increasing the laser power.34,55 Reproduced from ref. 55 with permission from the ACS Publications and ref. 34 with permission from John Wiley and Sons. | |
However, there is no single super-resolution method, which can produce an optimal performance in every case, including STED nanoscopy. Therefore, the potential limitations of STED nanoscopy together with the possible methods to overcome them should be listed. Instrumentation is the first issue that should be considered to obtain super-resolution imaging via STED nanoscopy. Although commercialized STED instruments have already been developed, optimizing them to reach their full potential is still important. Currently, new applications for both biological and material research, such as multi-color imaging56–58 and fluorescence lifetime imaging,59,60 are also necessary by combining STED imaging system with other spectroscopic methods. Therefore, further optimizations and simplifications of these integrated STED instruments are needed. On the other hand, some STED derivative imaging techniques should also be developed, such as triplet-state relaxation STED (T-REX STED)61 to eliminate the photo-bleaching and LocSTED62 by combining STED microscopy with single-molecule localization. Moreover, it is noted that high laser intensity to deplete the excited states of fluorophores can also easily cause photo-bleaching, leading to a poor photo-stability and finally limiting the imaging resolution, particularly in long-term monitoring and tracking. High intensity of the STED beam may also cause cell apoptosis and even cell necrosis, thus limiting its application in living cell imaging. On the other hand, the high irradiation may also prevent dynamic visualization in materials science at high resolution. From this point of view, the depletion intensity in STED nanoscopy should also be optimized and applied to match the requirements in different applications. Encouragingly, the use of suitable STED fluorophores with a high photo-stability can prevent photo-bleaching and then improve the imaging performance.63–67 Further details are provided in the following sections. However, although there are still several limitations in STED nanoscopy, by optimizing the STED laser, optical paths, scanning devices, detector systems, and also the employed luminescent materials, STED nanoscopy can be well used in the near future, such as in visualization and monitoring in biological research and materials science.
3. Material requirements in STED nanoscopy
To achieve ultra-high resolution imaging, suitable luminescent materials in STED nanoscopy are as important and necessary as the instrument itself. With the development of STED nanoscopy recently, a wide range of well-performing STED fluorophores, including QDs,68,69 UCNPs,70–72 fluorescent proteins,73–75 OLMs76,77 and their corresponding nanoparticles,55,78,79 are available. The categories and applications of these luminescent materials in STED nanoscopy are displayed in Fig. 2. Based on the short time feedback and also to easily achieve a satisfactory imaging performance, the reported fluorophores are practically preferred in new applications. However, in order to develop new fluorophores as imaging agents, a series of requirements for the luminescent materials should be determined.
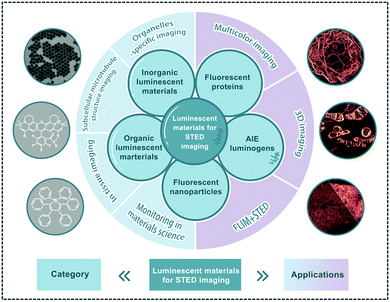 |
| Fig. 2 Brief category and application of luminescent materials for STED nanoscopy. | |
Generally, there are several typical criteria for use of the selected fluorophores in conventional optical imaging in biological research, which can be defined: (1) a high absorption cross section to undergo the excitation easily is fundamental for the fluorophores;80,81 (2) an excellent fluorescence performance, including high brightness and quantum yields, especially in the emission spectra for the applied aggregated states, is important to produce a high signal-to-noise ratio;82 (3) an excellent chemical stability to maintain its original physical and chemical properties under the different microenvironments is needed; (4) good photo-stability is also necessary to provide long-term tracking83,84 and process monitoring,85,86 such as the monitoring of cell apoptosis to evaluate tumor treatment efficiency in drug delivery; and (5) good biocompatibility and flexibility to link the biomolecular targets are needed in biological imaging in life sciences. However, in contrast to conventional optical imaging, STED nanoscopy has its own features, such as the use of a high-intensity depletion beam as the second laser.41,87 Therefore, in addition to the previous criteria, several crucial rules for the fluorescence emitters in STED nanoscopy are further required: (1) although it has been mentioned that a high photo-stability of the imaging agents is important in optical imaging, high intensity of the depletion beam in STED nanoscopy usually requires the fluorophore to exhibit a high resistance to photo-bleaching;88,89 For example, some STED fluorophores can be exposed in the depletion laser for 100 s under continuous irradiation (775 nm, 30.2 mW) without obvious photo-bleaching phenomena.88 Also, when a suitable STED power is used, imaging data can be successfully acquired over 200 frames for 10 min;88 (2) for commercial STED nanoscopy, depletion lasers with wavelengths of 592 nm (orange),90,91 660 nm (red),63,92 and 775 nm (far-red)93,94 are usually installed. The requirements on the emission spectra of the fluorophore must then match these wavelengths; (3) large Stokes' shifts (larger than 150 nm, Stokes' shift for some commonly used fluorophores were also included in Tables 1 and 2) are also anticipated in STED agents for super-resolution imaging.95,96 In terms of Stokes' shifts, anti-Stokes' shifts involved in some up-conversion nanoparticles (UCNPs) for STED nanoscopy can also be observed.41 However, it is noted that when these UCNPs were applied in STED nanoscopy, the depletion laser may also easily match with their multiple energy gaps, finally resulting in the enhancement of emission, and this may prevent depletion in STED imaging. This is also discussed in the following aspect; (4) since the employed fluorophores in STED nanoscopy may also be excited by the depletion laser from their excited state to even higher singlet states, the overlap between the excited state absorption spectrum and the stimulated emission spectrum should be prevented.97 In addition to the above-mentioned factors, the photo-blinking of some fluorophores may also play roles here due to the intersystem crossing from singlet state to triplet state, thus affecting the properties and depletion behavior of fluorophores in STED nanoscopy. However, it is noted that it is difficult to choose an ideal fluorophore to fulfil all the requirements. Therefore, the properties of the employed luminescent materials in STED nanoscopy should be fully considered. The STED fluorophores are introduced in the following sections in detail.
Table 1 Photo-physical properties, set-up parameters and imaging performance for reported OLMs in STED nanoscopy
Compounds |
Original name |
Absorption (nm) |
Emission (nm) |
Stokes’ shift (nm) |
STED beam (nm) |
STED power (mW cm−2) |
FWHMs (nm) |
Ref. |
Estimated by the absorption and emission spectra.
|
OLM-1
|
KK114 |
637 |
660 |
23 |
760 |
— |
25.00 |
140
|
OLM-2
|
KK9046 |
632 |
654 |
22 |
760 |
— |
25.00 |
140
|
OLM-3
|
Abiberi/STAR635 |
634 |
654 |
10 |
760 |
— |
25.00 |
140
|
OLM-4
|
Abiberi/STAR635P |
635 |
655 |
20 |
760 |
— |
25.00 |
140
|
OLM-5
|
3a |
636 |
659 |
23 |
775 |
— |
— |
141
|
OLM-6
|
3b |
633 |
653 |
20 |
775 |
— |
— |
141
|
OLM-7
|
3c |
633 |
653 |
20 |
775 |
— |
44.00 |
141
|
OLM-8
|
3d |
— |
— |
— |
775 |
— |
— |
141
|
OLM-9
|
14-R |
579 |
609 |
30 |
775 |
— |
36.00 |
141
|
OLM-10
|
1c |
521 |
546 |
25 |
618 |
14 |
40.00 |
77
|
OLM-11
|
1f |
609 |
634 |
25 |
775 |
14 |
50.00 |
77
|
OLM-12
|
1d |
581 |
607 |
26 |
775 |
14 |
≤60 |
77
|
OLM-13
|
1i |
645 |
661 |
16 |
775 |
14 |
— |
77
|
OLM-14
|
H-Bphox |
— |
— |
— |
— |
— |
— |
142
|
OLM-15
|
Ph-Bphox |
415 |
528 |
113 |
— |
— |
— |
142
|
OLM-16
|
C-Bphox |
431 |
522 |
91 |
— |
— |
— |
142
|
OLM-17
|
C-Naphox |
488 |
495–590 |
7–102 |
592 |
— |
— |
142
|
OLM-18
|
PB430 |
426a |
542a |
116 |
592 |
30 |
76.00 |
67
|
OLM-19
|
MitoPB Yellow |
488 |
570–750a |
82–262 |
660 |
270 |
91.00 |
65
|
OLM-20
|
1 |
488 |
590–700 |
102–212 |
660 |
50 |
151.72 |
151
|
OLM-21
|
4 |
470 |
565–700 |
95–230 |
660 |
— |
— |
151
|
OLM-22
|
LC |
405 |
595 |
190 |
595 |
— |
40–70 |
155
|
OLM-23
|
14+ |
650 |
665 |
15 |
775 |
— |
35.00 |
158
|
OLM-24
|
5 |
405 |
500–600a |
95–195 |
660 |
30 |
80.00 |
159
|
OLM-25
|
NIM-3A |
405 |
495–574a |
90–169 |
— |
— |
— |
160
|
OLM-26
|
CM2P |
405 |
460–500 |
55–95 |
595 |
— |
50.00 |
161
|
OLM-27
|
MePyr500 |
561 |
600a |
39 |
775 |
170 |
— |
162
|
Table 2 Photophysical properties, STED set-up parameters and imaging performance for reported AIEgens (AIE-1–AIE-8)
Compounds |
Original name |
Excitation (nm) |
Emission (nm) |
Stokes’ shift (nm) |
STED beam (nm) |
STED power (mW cm−2) |
FWHM (nm) |
Ref. |
Estimated by the absorption and emission spectra.
|
AIE-1
|
Hexaphenylsilole (HPS) |
360a |
490a |
130 |
532 |
25 |
— |
47
|
AIE-2
|
TPA-T-CyP |
480 |
684 |
204 |
775 |
150 |
74.37 |
48
|
AIE-3
|
DB-TBT |
489 |
619 |
130 |
— |
— |
— |
175
|
AIE-4
|
DP-TBT |
497 |
657 |
160 |
775 |
266 |
178.00 |
175
|
AIE-5
|
TTF |
594 |
660 |
66 |
775 |
312.5 |
30.70 |
49
|
AIE-6
|
Blue-AIE-OXE |
330a |
470a |
140 |
— |
— |
— |
50
|
AIE-7
|
Green-AIE-OXE |
370a |
510a |
140 |
— |
— |
— |
50
|
AIE-8
|
Red-AIE-OXE |
405 |
640a |
235 |
660 |
100 |
95.00 |
50
|
4. Luminescent materials for STED imaging
In recent years, tremendous efforts have been devoted to the exploration of fluorophores for STED nanoscopy. This is very important to acquire a superior imaging resolution in biological research or materials science. Typically, these materials can be categorized as inorganic fluorophores,98 including QDs,68,69 carbon dots99,100 and UCNPs,70–72 and their organic counterparts. Most of these inorganic materials exhibit a much higher photo-stability than their organic counterparts. However, organic luminescent materials usually own an impressive chemical tailor-ability,101 a large Stokes' shift,95,96 and a good biocompatibility,102 which also well meet the key requirements of STED nanoscopy. This indicates that all these STED fluorophores have their unique characteristics. Therefore, the major categories of luminescent materials for super-resolution imaging via STED nanoscopy are discussed in the following sections in detail in this review.
4.1. Inorganic luminescent materials for STED imaging
In this section, the recent advances in quantum dots, carbon dots, and up-conversion nanoparticles for STED imaging are illustrated in detail.
4.1.1 Fluorescent quantum dots and carbon dots.
Generally, QDs exhibit an excellent photo-stability, a high quantum yield, and a tunable macromolecular size.103,104 Moreover, their luminescent performance can be easily tuned by using element doping or by changing their size. Therefore, QDs show great potential to be used in optical imaging, and several studies have been carried out in STED imaging using QDs as fluorophores. Stefan W. Hell et al. reported Mn2+-doped ZnSe QDs in 2008.105 The fluorescence was depleted efficiently (90%) and this resulted in a large number of fluorescent switch on–off cycles before photo-bleaching occurred. Finally, an improvement in resolution by a factor of 4.4 was obtained in reversible saturable or switchable optical fluorescence transitions (RESOLFT) microscopy when compared with that of confocal microscopy.105 These results firstly demonstrated their potential in STED and its combined microscopy. After that, luminescence depletion imaging was then reported in CdSe/14CdS nanocrystal QDs with a high depletion efficiency of 92% by using a doughnut-profiled laser.106 Finally, it's observed that compared with those obtained via confocal imaging with a full width at half maximum value (FWHM) of 450 ± 90 nm, an obviously enhanced FWHM value of 40 ± 10 nm was achieved in STED nanoscopy. This indicates the higher imaging resolution that can be obtained by using nanocrystal QDs in this case. However, it is noted that broad excitation spectra usually occur in QDs which goes deeply into their emission spectra, leading to small Stokes' shifts, which limit the choice of an appropriate depletion beam. To conquer this limitation, Stefan W. Hell et al. used a 775 nm STED laser in luminescence depletion imaging and commercially available ZnS-coated CdSe QDs and CdTe QDs (Fig. 3A) to obtain super-resolved background-free images (Fig. 3B).68 Interestingly, these QDs in their bio-functionalized form, such as Qdot705, were also used in cellular imaging: a 2.7-fold improvement in the resolution was successfully achieved, implying a significantly enhanced resolution (Fig. 3C–K).
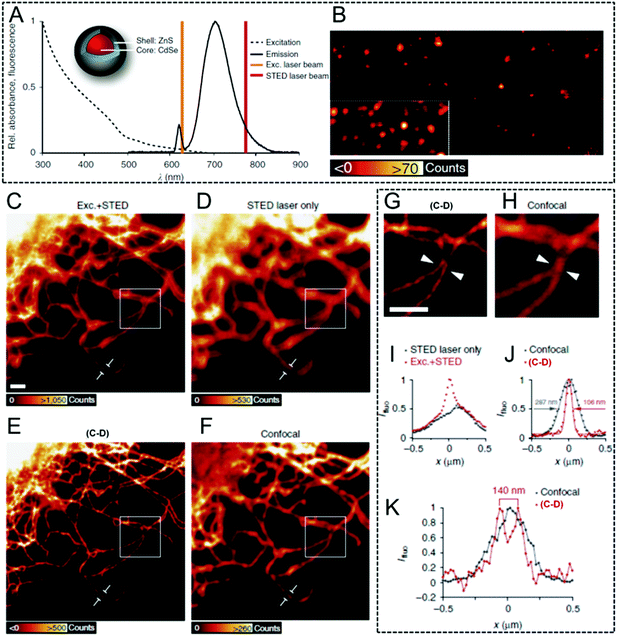 |
| Fig. 3 (A) The excitation and emission spectra of the antibody-coupled ZnS-coated CdSe (Qdot705) with an excitation laser (628 nm) and a STED laser (775 nm). (B) Qdot705 imaged via STED nanoscopy to produce a background-free final image. (C) Vimentin fibers in immunostained cells with Qdot705. The fibers were imaged with both an excitation and a STED laser beam. (D) QDs imaged only via a STED laser beam. (E) Subtraction of the image C from image D. (F) Their corresponding confocal image in the same region. (G) Enlarged image of the white square in inset E. (H) Enlarged image of the white region in inset F. (I) Intensity profile along a single vimentin fiber shown in C and D. (J) Intensity profile along a single vimentin fiber depicted at E and F. (K) Line profile of the position indicated by the arrow heads in G and H. The scale bar is 1 μm.68 Reproduced from ref. 68 with open access by Springer Nature. | |
Halide perovskite QDs (CsPbX3, X = Cl, Br, or I)107–109 have also received considerable attention due to their advantages, such as high photoluminescence brightness and photo-bleaching resistance. Interestingly, stable stimulated emissions with a low threshold can also be easily obtained in perovskites, indicating their potential in long-term STED nanoscopy applications. Qu and Song et al. firstly reported STED super-resolution imaging using CsPbBr3 QDs110 (Fig. 4A): a series of bright spots are captured in confocal microscopy, but becoming much more visible with an enhanced lateral resolution of 20.6 nm when working with STED nanoscopy. Additionally, by increasing the STED laser power, the imaged spot size decreases rapidly, finally resulting in a size of 50 nm upon a STED irradiation of 5 mW (Fig. 4B). The resolution and power of the STED depletion laser for perovskite QDs and several other commonly used fluorophores were also compared, which is also summarized in Table S2 (ESI†). It is noted that although the laser power is limited, high imaging performance was still obtained. This result demonstrates that perovskite QDs can be used as efficient probes in STED imaging with super-resolution capability upon a low power irradiation. To further confirm the super-resolution capability of CsPbBr3 QDs, anodized aluminum oxidation (AAO) masks with a porous morphology (pore diameter = 70 nm, interpore distance = 110 nm) were also imaged (Fig. 4C–J). As anticipated, after dropping the CsPbBr3 suspension into the masks, it is difficult to detect the porous surface via confocal microscopy. However, by using STED nanoscopy, discrete spots similar to SEM images with a diameter of around 65 nm and an interpore distance of 126 nm can be observed, indicating that perovskite QDs are promising candidates to be used as STED imaging agents in super-resolution imaging.
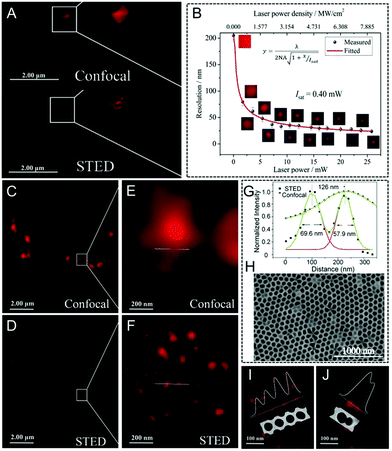 |
| Fig. 4 (A) Image of CsPbBr3 QDs in confocal mode and STED mode. (B) Resolution of the STED images by using CsPbBr3 QDs for a higher laser power. Imaging of an AAO mask filled with CsPbBr3 QDs captured by (C) confocal mode and (D) STED mode (E and F are their corresponding magnified images). (G) Fluorescence signal curves along the lines across the pores in the AAO mask. (H) SEM morphology of the AAO mask filled with CsPbBr3 QDs. (I) and (J) The morphology of the AAO mask by using CsPbBr3 QDs.110 Reproduced from ref. 110 with permission from John Wiley and Sons and Copyright Clearance Center. | |
In contrast to fluorescent QDs, carbon-based nanomaterials can also be an appealing candidate to be used as fluorophores in super-resolution imaging tools, such as STED nanoscopy.69,111 Among them, nanodiamonds with point defects exhibit good photo-luminescent properties.112 Moreover, they are extremely photo-stable and no obvious photo-bleaching occurs even under continuous laser illumination. Based on this consideration, Stefan W. Hell and co-workers obtained a 6-fold enhancement in the resolution (35 nm) by using the prepared nanodiamonds in STED nanoscopy in 2009.113 Their work provides an efficient strategy to investigate the structures in cellular organelles and even in subcellular compartments. Later, Chang et al. also utilized bovine serum albumin- (BSA) and a-lactalbumin- (a-LA) coated nanodiamonds in STED nanoscopy to monitor intracellular interactions with an ultra-high resolution.114 Carbon dots (C-dots) with biocompatible and photo-stable features, and also a size/excitation wavelength-dependent photo-luminescence emission, are also an alternative to QDs and fluorescent nanodiamonds.115,116 They can also be well used as imaging agents in long-term super-resolution imaging. In 2014, Pompa et al. prepared C-dots with an average particle size of ~5.5 nm for a STED nanoscopy measurement (Fig. 5A).111 When C-dots dispersed in a coverslip are imaged by both confocal and STED nanoscopy: a significantly enhanced lateral resolution in the STED mode is achieved with an FWHM value of only 71 nm. This is far below the resolution limit of confocal microscopy (192 nm, Fig. 5B). In addition, the cellular viability by incubating the C-dots in MCF7 cells demonstrates their high biocompatibility, even for a high concentration of 170 nM (Fig. 5C). Successively, applications with C-dot imaging in both fixed and living cells were conducted. The results show a clear visual improvement with a resolution of 30 nm in STED nanoscopy.
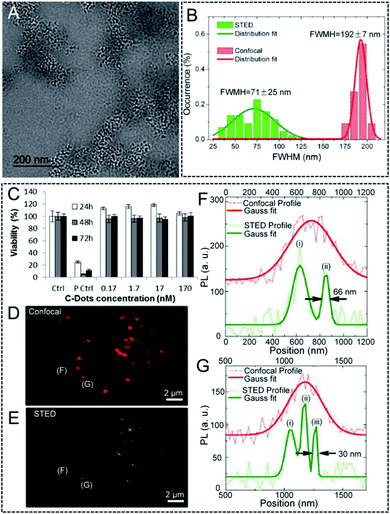 |
| Fig. 5 (A) TEM image of the C-dots. (B) Statistical analysis of the FWHM values obtained by confocal and STED nanoscopy. (C) Viability of the MCF7 cells incubated with C-dots, evaluated via the WST-8 assay (“Ctrl” corresponds to non-treated control cells; “P Ctrl” refers to cells treated with 5% DMSO). Confocal (D) and STED (E) imaging of the C-dots in fixed MCF7 cells. (F and G) Two representative line profiles extracted from the signals of C-dots in cells, together with their calculated FWHM values.111 Reproduced from ref. 111 with permission from the Royal Society of Chemistry. | |
4.1.2. Fluorescent up-conversion nanoparticles.
Up-conversion nanoparticles, which are able to convert near-infrared (NIR, 750–1000 nm) light as the excitation source into visible and even ultraviolet (UV) emission, have triggered much interest over the last few decades.117,118 Due to their unique features in the optical up-conversion, the limitations of other imaging agents, such as photo-bleaching, interference by auto-fluorescence,119,120 and high light toxicity, can be fully prevented. Moreover, these UCNPs can easily undergo bio-conjugation, thus making them biocompatible and then finally being well used in biological imaging. However, despite their excellent luminescence properties, UCNPs are not well established in STED nanoscopy. Encouragingly, Jin and his co-workers investigated the applications of Tm- (Fig. 6A) and Yb- (Fig. 6B) doped UCNPs for STED ultra-high resolution imaging in 2017.70 A doughnut-shaped 808 nm depletion beam and a 980 nm excitation beam were employed in this case. As they observed, compared with the bright spots observed in confocal microscopy, STED nanoscopy is able to detect adjacent but resolved UCNPs in both Tm- and Yb-doped UCNPs. This results in an improved resolution of 27.4 nm and 28.4 nm, respectively (Fig. 6C–F). Their corresponding FWHM values were also obtained and are shown in Fig. 6G and H. These measurements confirm that when such highly concentrated UCNPs are combined with the upconversion-STED technique, biological investigations at the nanoscale level with ultra-high resolution may be achieved. Later, He et al. developed a lanthanide-doped UCNP system (NaYF4:18% Yb3+,10% Tm3+) and then applied it successfully to two-color STED imaging.41 By employing STED nanoscopy, a resolution of approximately 66 nm was obtained (Fig. 7). The same group also conducted a surface modification by using poly(acrylic) acid and used antibodies to clearly label the subcellular structures. They achieved a lateral imaging resolution of about 82 nm, which further demonstrates the potential of UCNPs as STED agents in super-resolution cell imaging.41
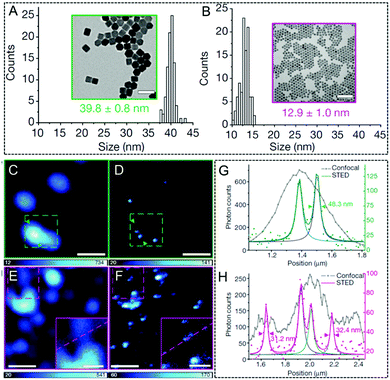 |
| Fig. 6 TEM image of 8% Tm-doped UCNPs (A). TEM image of 20% Yb-doped UCNPs (B). Confocal (C) and STED (D) microscopy images of the UCNPs with 8% Tm-doped reported in A (the scale bar is 100 nm). Confocal (E) and STED (F) microscopy image of the 20% Yb-doped UCNPs reported in B (the scale bar is 500 nm and the scale bar for inset image is 200 nm). (G) Intensity profiles of the zone between arrows across two UCNPs in C and D. (H) The intensity profiles of the square region between arrows along two UCNPs in E and F.70 Reproduced from ref. 70 with permission from the Springer Nature. | |
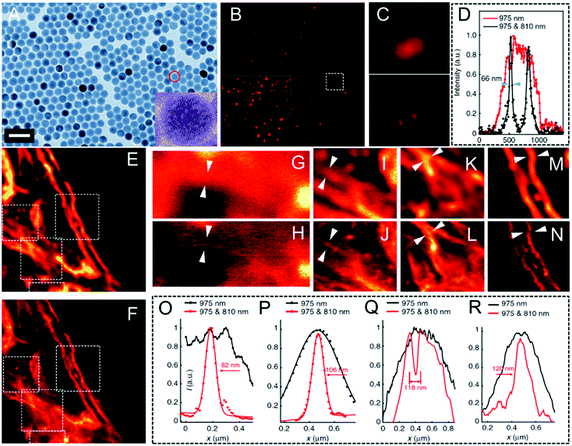 |
| Fig. 7 (A) TEM morphology of UCNPs (NaYF4:18% Yb3+,10% Tm3+; the scale bar is 50 nm). (B) Super-resolution image of the UCNPs in STED nanoscopy (the scale bar is 2.5 μm). The inset shows the image obtained by multiphoton laser scanning microscopy. (C) Magnified images of the UCNPs in the same region detected by multiphoton laser scanning microscopy and STED nanoscopy. (D) Intensity recorded over several scans for a 975 nm excitation only and a 975 nm and 810 nm co-irradiation. (E) Multiphoton imaging obtained via a 975 nm excitation of the cytoskeleton structures and of the desmin proteins in HeLa cancer cells incubated with modified UCNPs (the scale bar is 2 μm). (F) Region in the inset E but imaged in super-resolution mode. (G–N) Magnified areas selected in the inset E and F (marked by white dotted squares) and their line profile analysis (the images reported in G, I, K, and M are obtained from the white dotted squares in E; the images reported in H, J, L, and N are obtained from the white dotted squares in F). (O–R) Line profile analyses of areas suggested by the arrow in G–H, I–J, K–L, and M–N, respectively.41 Reproduced from ref. 41 with open access from Springer Nature. | |
4.1.3. Other inorganic luminescent materials.
Although many types of nanoparticles, such as QDs, C-dots, and also UCNPs, have been employed as imaging agents in STED nanoscopy, there is still room to develop new fluorophores to fulfil and further enhance their imaging resolution in STED nanoscopy.121 For example, Sivan and co-workers demonstrated the potential of hybrid nanoparticles in super-resolution imaging.122 Their system consisted of gold nanospheres (diameter = 20 nm) coated with fluorescently doped silica for STED nanoscopy. Finally, a significantly improved imaging resolution (75 nm) far beyond the diffraction limitation of CLSM was obtained with a much lower STED laser power. It's worth noting that the hybrid nanoparticles exhibit an enhanced stimulated depletion performance by using the plasmon resonance. Huidobro et al. also reported the use of gold nanorods at the nanoscale level in STED nanoscopy.123 They provided an improvement in the resolution of up to 50% in comparison to the probes without plasmonic components.
4.2. Fluorescent proteins for STED imaging
Besides the aforementioned inorganic nanoparticles, great efforts were also devoted to generating fluorescent proteins (FPs)124,125 for super-resolution imaging due to their merits of genetically encoding and expressing. Moreover, such tagging method enables the labeling of intracellular proteins with various fluorophores, thus providing a simple but powerful approach to specific labeling in living cells via STED nanoscopy. In 2010, Hell and his co-workers labeled human O6–alkylguanine–DNA alkyltransferase (hAGT) with commercially available red-emitters based on tetramethylrhodamine (TMR) to produce a fluorescent tag in living cell imaging (Fig. 8A).126 The staining procedure achieved by using organic dyes, such as TMR-Star in this case, not only maintains a perfect cell integrity, but also shows individual filaments as cellular structures with an enhanced resolution down to ∼40 nm via STED nanoscopy. However, the cellular structures tend to be blurred when confocal microscopy is performed (Fig. 8B and C). A yellow FP tag was also attached by Hell et al. to visualize the individual structural elements in the endoplasmic reticulum (ER).127 As displayed in Fig. 9A and B, fluorescence signals in the large areas can only be recorded via confocal microscopy, whereas the polygonal network in the ER of living cells with an FWHM of only 52 nm can be fully captured via STED nanoscopy. This leads to a 4-fold improvement in the imaging resolution (Fig. 9C). Moreover, the three-dimensional (3D) optical imaging at ultra-high resolution via FP tagged ER can also be achieved in this case. The dynamic monitoring of the ER movement with a 50 nm lateral resolution was also successfully conducted to reveal the structural changes in the ER network, including the forming and vanishing of the enclosures (Fig. 9D).
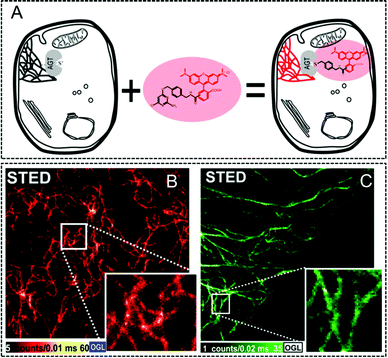 |
| Fig. 8 (A) Schematic illustration of the labeling process by using TMR-Star and hAGT in living cells. (B) Confocal and (C) STED image of vimentin labeled with TMR via hAGT in living cells (the scale bar is 1 μm).126 Reproduced from ref. 126 with permission from Elsevier. | |
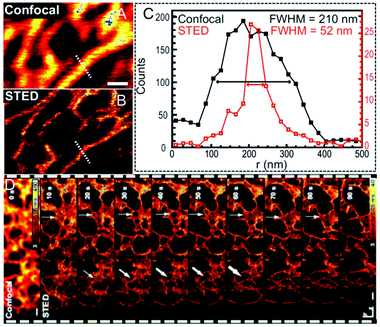 |
| Fig. 9 (A) Confocal and (B) STED image revealing the network in the ER for living cells. The scale bar represents 500 nm. (C) Line profile analyses of the A and B inset with the calculated FWHM values. (D) Dynamic monitoring of the ER movement with a subdiffraction resolution via STED nanoscopy. The first image corresponds to the confocal contrast image and the arrow heads manifest the structural changes during the collecting process. The scale bar represents 500 nm.127 Reproduced from ref. 127 with permission from the Copyright (2008) National Academy of Sciences, USA. | |
Nienhaus et al. prepared a fluorescent marker with a far-red emitting properties protein named mGarnet2.124 The molecular structure of mRuby and of the residues modified in mGarnet2 are outlined in Fig. 10A–C. In order to investigate the performance of mGarnet2 in fusion proteins, confocal microscopy was initially used to image versatile fusion structures expressed in live COS-7 cells to visualize the actin, the microtubular cytoskeletal, and the structures (Fig. 10D–F). The properties in mGarnet2 further showed its great potential as a fusion marker. Furthermore, mGarnet2 in living cells was also imaged by employing confocal microscopy and STED nanoscopy, respectively (Fig. 10G). Here, microtubules labelled with mGarnet2-RITA in living cells can be clearly observed with a significantly enhanced resolution via STED nanoscopy. According to the cross-sectional files in the microtubules (Fig. 10H), a narrower intensity file was also recorded via STED imaging with an FWHM value of 69 nm.
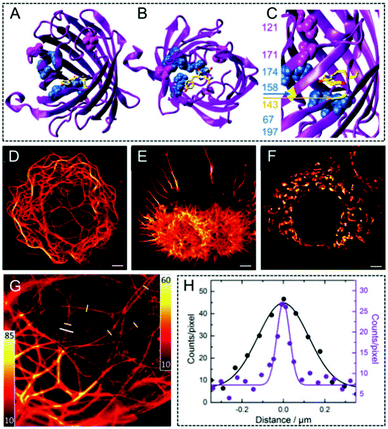 |
| Fig. 10 (A–C) Molecular structure of mRuby and of the residues modified to generate mGarnet2. Confocal microscopy images of the mGarnet2 fusion proteins expressed in living cells (“D” corresponds to mGarnet2-RITA, “E” refers to LifeAct-mGarnet2, and “F” corresponds to mito-mGarnet2). The scale bar is 5 μm. (G) Confocal and STED image of a live COS-7 cell transfected with a plasmid for the expression of mGarnet2-RITA. (H) Cross sections of a microtubule labeled by the white line in inset G detected via confocal (black) and STED (violet) imaging.124 Reproduced from ref. 124 with permission from the Royal Society of Chemistry. | |
Benefiting from the reduced absorption, the scattering, and the auto-fluorescence in the near infrared (NIR) region, high performance biological imaging, such as deep-tissue imaging can be easily achieved.73 Furthermore, the lower photo-toxicity and the potential of multi-color super-resolution imaging are two pivotal characteristics for these fluorophores in the NIR window. Therefore, based on these considerations, Jakobs and his co-workers recently developed SNIFP, an NIR fluorescent protein, which can be listed as a fusion marker and applied for live-cell imaging using confocal microscopy and STED nanoscopy.128 As shown in Fig. 11A–C, in contrast to confocal microscopy, the cellular structures in living HeLa cells expressing VIM-SNIFP or SNIFP-NUP50 can be detected with ultra-high resolution in STED nanoscopy (Fig. 11A). Moreover, the FWHM can be determined via the cross-sections: FWHM values of ∼280 nm and ∼80 nm are observed for confocal and STED nanoscopy (Fig. 11B), respectively. This demonstrates the high resolution improvement by using STED nanoscopy. Interestingly, confocal imaging (Fig. 11E) and also their consecutive STED images are also recorded (Fig. 11F–H), showing the impressively high photo-stability of SNIFP and its potential applications in dynamic monitoring and long-term tracking. In addition, due to the complementary emission spectra of mCherry26, which is excited at 587 nm, and SNIFP, red/NIR dual-color super-resolution imaging can also be successfully obtained via STED nanoscopy (Fig. 11D).
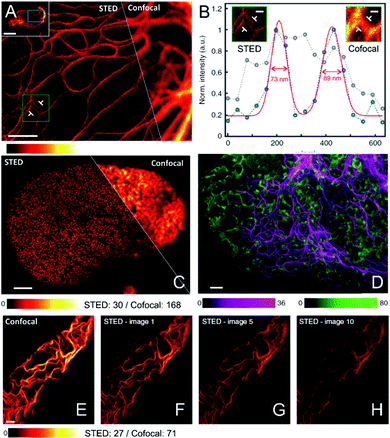 |
| Fig. 11 (A) NIR fluorescence images acquired via STED and confocal microscopy in living human cells expressing VIM-SNIFP (the scale bar is 2 μm). (B) Line profiles detected at the position indicated in A inset together with their calculated FWHM values (the scale bar is 400 nm). (C) NIR fluorescence images of nuclei in living human cells, which express SNIFP-NUP50, acquired via STED and confocal microscopy. (D) Red confocal/NIR STED dual-color imaging detected by using VIM-SNIFP (magenta) in STED mode and mCherry (green) targeted ER in confocal mode. (E) Confocal image and (F–H) consecutive STED images of a living HeLa cell expressing VIM-SNIFP (the scale bar is 2 μm).128 Reproduced from ref. 128 with open access from Springer Nature. | |
Recently, protein-based fluorescent nanoparticles73,129,130 have been reported by employing commercially available dyes during their synthesis. A highly superior photo-stability in protein-based fluorescent nanoparticles is obtained when compared to their corresponding incorporated dyes under an intense STED illumination. By employing Atto647N-transferrin nanoparticles, for example, a 4-fold improvement in the imaging resolution of these nanoparticles was achieved via STED nanoscopy. Moreover, detailed imaging in living cells was also obtained in this case due to the following merits: (1) good biocompatibility with high cell viability of 80% after incubating for 24 h; (2) excellent colloidal stability with no observed precipitation or agglomeration phenomenon for more than one year. In addition to the imaging performance with a 4-fold improvement in resolution in STED mode over confocal mode, this work also provides a strategy to enrich the toolbox of fluorescent proteins for STED super-resolution imaging.131
4.3. Organic luminescent materials for STED imaging
To meet the key requirements of STED nanoscopy, various kinds of inorganic luminescent materials, such as QDs and UCNPs, have been reported recently. However, although significant progress in developing inorganic fluorophores for STED super-resolution imaging has been achieved, these materials still show several limitations: (1) they exhibit potentially toxic features, especially when heavy metal atoms are used during the preparation process;132,133 (2) preparing nanoparticles with a uniform size remains challenging; (3) an inferior reproducibility hinders their large-scale preparation; (4) the small Stokes' shift of QDs,134,135 which usually lead to an obvious re-excitation by the STED beam, results in a poor depletion efficiency; (5) the inferior emission brightness of UCNPs, in particular for the water-soluble ones capped with hydrophilic ligands, is still a limitation for STED nanoscopy. However, it is interesting to note that alternative to inorganic fluorophores for STED imaging, organic luminescent materials (OLMs) exhibit several advantages in super-resolution imaging136 due to their large Stokes' shift, excellent chemical stability, and outstanding biocompatibility. Furthermore, their chemical structures can also be well engineered, thus leading to finely tunable luminescence properties and also the optimized imaging performance in STED nanoscopy. Based on these considerations, the recent advances in OLMs for STED imaging with ultra-high resolution are described in this section. This includes rhodamines/phosphole-based dyes, organic metal complexes and several other reported organic STED agents (Scheme 1). All their key properties, set-up parameters and imaging performance in STED nanoscopy are summarized in Table 1. It is noted that to unify the compound names in this work, we used “OLM” as the abbreviation here. However, the original names for these used compounds were also included in Table 1 for a much better understanding.
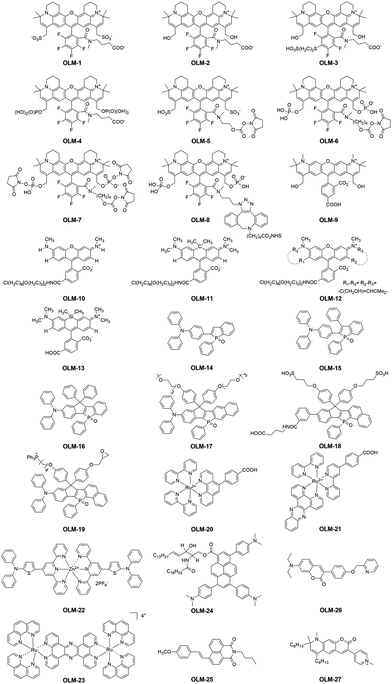 |
| Scheme 1 Chemical structures of the reported OLMs (OLM-1–OLM-27) for STED super-resolution imaging. | |
4.3.1. Rhodamine-based organic fluorophores for STED imaging.
Recently, rhodamines and their derivatives have been widely used as imaging agents in biological research.77,137–139 They show several benefits in super-resolution imaging via STED nanoscopy: (1) a high chemical stability to keep the original physical and chemical properties under the microenvironment of different chemical factors; (2) good photo-physical properties, such as a high absorption ability (extinction coefficient > 1 × 104 M−1 cm−1) and an impressive emission quantum yield (>70%);77 (3) an excellent photo-stability to provide image frames (>10) under a STED depletion laser;77 (4) an easy modulation of their chemical structures to fine-tune the fluorescence properties; (5) an efficient labeling ability to specifically bind their biological targets; (6) a good penetrability via the plasma membranes of living cells. Due to these advantages, Hell and his co-workers prepared several red fluorescent dyes of OLM-1–OLM-4 containing rigidized rhodamine building blocks.140 Their chemical structures are shown in Scheme 1. To fully compare their photo-physical properties and imaging performance in STED nanoscopy, the red fluorescence dyes Atto647N and KK114 were used as the benchmark. In contrast to KK114, OLM-1 exhibits a much longer linker between the dye core and the NHS moiety, which improves the chemical stability of NHS esters in the molecular backbone, but maintaining the excellent properties in KK114. Additionally, other substitutions in the skeleton of KK114, such as the addition of a hydroxyl group and mercaptoethyl sulfonic acid, are introduced to produce OLM-2 and OLM-3. Although minor effects on their spectral properties are observed, an enhanced cell-permeability and water solubility are obtained in OLM-2 and OLM-3, respectively. Interestingly, when phosphate groups are employed, the first phosphorylated rhodamine (OLM-4), which can be used as an alternative to the widely used sulfonated fluorophores, is obtained. The large polarity and grown number of acidic protons in OLM-4 significantly enhance its solubility in aqueous solutions. Due to their fluorescence properties similar to KK114, it is noted that all these developed new dyes are especially suitable for immunofluorescence imaging, where an outstanding spatial resolution can be obtained in STED images (Fig. 12). Moreover, in the case of OLM-4, a much higher signal-to-background ratio can be observed, leading to a clearer staining contrast. In addition, to further understand the spatial resolution achievable with these dyes, point-like fluorescent objects, probably generated by individual antibody clusters, can also be identified and their profiles display a resolution of 230 nm in the confocal channel. However, a resolution of ∼25 nm can be achieved in the STED case (Fig. 12G–I and Table 1), leading to a ∼9-fold increase in the resolution by using STED nanoscopy. This result further suggests that these dyes are good candidates to substitute KK114 in super-resolution STED imaging.
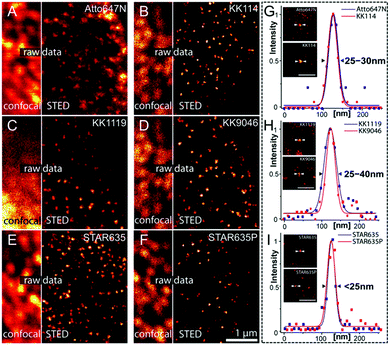 |
| Fig. 12 Confocal and STED image of the nuclei in fixed PtK2 cells by using the benchmark dyes Atto647N (A) and KK114 (B). Confocal and STED image of the nuclei in fixed PtK2 cells by using the OLM-1 (C), OLM-2 (D), OLM-3 (E), and OLM-4 (F) red dyes. (G–I) The line profiles with the calculated FWHM for KK1119, KK9046, STAR635, and STAR635P for STED imaging are also provided (the scale bar is 500 nm).140 Reproduced from ref. 140 with open access from Springer Nature. | |
Hell et al. developed a series of rhodamines (OLM-5–OLM-9, Scheme 1)141 in 2014 with a strong red fluorescence signal and two polar residues, as for example the hydroxyls, primary phosphates or sulfonic acid groups. High emission brightness and photo-stability were observed, facilitating the application of these fluorophores in STED imaging, especially in two-color STED biological imaging with high signal-to-noise ratio. These dyes were initially tested in single-color STED nanoscopy, where the nuclear pore complex and the tubulin cytoskeleton of mammalian cells were labelled with OLM-5–OLM-8 and spectrally similar contrast probes, Atto647N, KK114, and Abberior Star 635P. As depicted in Fig. 13, compared with the fluorescence image obtained by confocal microscopy, clearer images are obtained with OLM-5–OLM-8via STED with a high staining specificity, low background, and ultra-high resolution. Then, based on the absorption and emission spectra of OLM-7 and OLM-9 (Fig. 13E and Table 1), two-color STED imaging by a single wavelength (775 nm) with low spectral crosstalk can be obtained (Fig. 13F, G and Table 1). It is noted that OLM-7 and OLM-9 exhibited high brightness with PLQY values of up to ∼90%, which also displayed impressive values when conjugating with antibodies. Therefore, although the detection windows in this case are narrow, OLM-7 and OLM-9 still provide bright images in both channels. This fosters the use of two-color STED imaging in several applications by labeling these dyes, such as in the visualization of nuclear pore complexes (NPCs) and Golgi apparatus in human fibroblast cells. In the first case, the protein giantin can target clusters in the membrane of the Golgi apparatus (Fig. 13F). The matrix protein GM130 can enter the interior of the giantin marked structures (Fig. 13G), indicating that both dyes provide high-resolution two-color STED imaging with an excellent signal-to-noise ratio and 40 nm resolution. Recently, bright and photo-stable rhodamines and carbopyronines (OLM-10–OLM-13, Scheme 1) with absorption in the range of 500–630 nm have also been reported by the same research group (Table 1).77 Besides the superb fluorescence performance, their specific labeling ability of the cytoskeletal filaments by the HaloTag technology was also observed, thus providing the single- and two-color images of these live cells via STED nanoscopy with a resolution of 40–60 nm (Table 1).
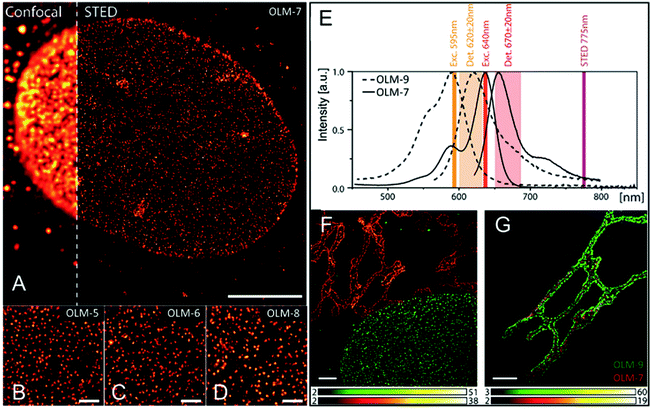 |
| Fig. 13 Single-color STED imaging of the central subunits of the nuclear pore complex in fixed HeLa cells by labeling with the OLM-7 (A, scale bar = 5 μm), OLM-5 (B), OLM-6 (C), and OLM-8 (D) dyes. The scale bar for B–D is 1 μm. (E) Spectral rationale of the setup used and overlap with the spectra of the OLM-9 and OLM-7 dyes. (F) Two-color STED cellular nanoscopy of giantin (OLM-7) and NPC (OLM-9). (G) Two-color STED cellular nanoscopy of giantin (OLM-9) and GM130 (OLM-7). The scale bar for F and G is 2 μm.141 Reproduced from ref. 141 with permission Copyright Wiley-VCH GmbH. | |
4.3.2. Phosphole-based organic fluorophores for STED imaging.
As previously mentioned, an intensive STED beam with a high power usually generates rapid photo-bleaching of the fluorophores when it is used in STED nanoscopy. This phenomenon is even more pronounced for organic fluorophores, probably preventing their applications in biological imaging and tracking with an ultra-high resolution. Therefore, organic fluorophores with high photo-bleaching/photo-blinking resistance are urgently needed. To further enrich the toolbox of highly photo-stable organic STED agents, Yamaguchi and his co-workers developed a core scaffold of (N,N-diphenylamino)phenyl-substituted benzophosphole p-oxide (OLM14–OLM17).142 Here, the electron donors and the electron acceptors were employed simultaneously, leading to excellent fluorescence properties, such as excellent photo-luminescence quantum yields and large Stokes' shift. To take advantage of these features and provide such scaffolds with a high photo-stability, OLM-17 with emission in the range of 500–750 nm was synthesized.142 This compound displays an impressively high luminescence quantum yield of up to 71% and a large Stokes' shift of 5900 cm−1 in a MeOH solution. Studies on its excited-state dynamics indicate that OLM-17 not only exhibits a small knr value due to its structural rigidity to suppress the non-radiative decay, but also a high kr value due to its enlarged π conjugation, which accelerates the radiative decay of its excited states. The phosphoryl moiety and the carbon bridge, which are located close to the central double bond in OLM-17, may also prevent a series of degradation processes, such as the reaction with the O2 singlet or the hydroxyl radical via photoexcitation, probably producing a photo-stable characteristic. To confirm this hypothesis, the photostability of OLM-17 was investigated and, as shown in Fig. 14, no significant photo-bleaching can be observed even after successive exposure to the depletion laser irradiation (405 nm) for 2 hours (Fig. 14A). The commercial probes Alexa Fluor 488 (Alexa 488) and Atto 488 for STED imaging were introduced in the system as reference compounds. After a 2 h irradiation, only 26.2% of Alexa 488 and 96.7% of Atto 488 were present in the system, but the fluorescence intensity of OLM-17 persisted (Fig. 14B). Even when incubating the HeLa cells, OLM-17 still remained intact (Fig. 14C). To further test this concept, a series of fluorescence images acquired by STED nanoscopy were recorded to produce ER-specific images. The fluorescence signals show that OLM-17 remains virtually unchanged (Fig. 14D), whereas the signal of the cells labelled with Alexa 488 is almost completely quenched (Fig. 14E). In order to improve the water-solubility and the specific binding ability to a series of biological targets, OLM-18 with a similar molecular skeleton to the one of OLM-17 was then developed by Yamaguchi et al. in 2017 (Scheme 1).67 The solubility of OLM-18 in water is significantly improved and a robust emission with a high emission efficiency of 67% is obtained in aqueous media due to the lower interactions between the electron-donating and electron-withdrawing units. In addition, the functional group of OLM-18 easily fosters the bioconjugation with antibodies for immunolabeling in biological applications. Benefiting from its impressive luminescence performance and excellent resistance to photobleaching, OLM-18 can be finally used in STED super-resolution imaging, 3-D reconstruction via STED nanoscopy, and multicolor STED imaging.
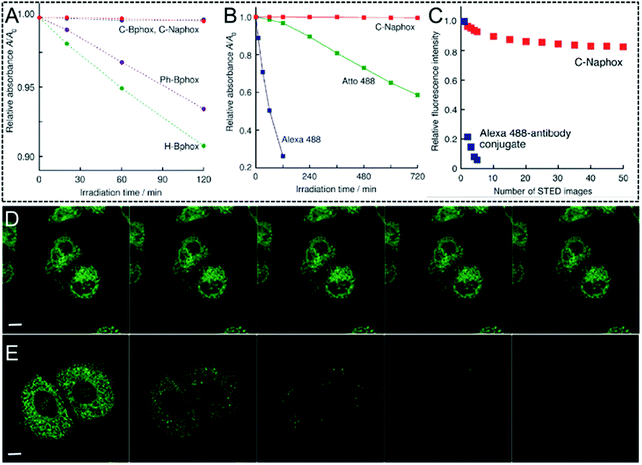 |
| Fig. 14 (A) Relative absorbance values of OLM-15 (purple circle), OLM-14 (green circle), OLM-16 (blue circle), and OLM-17 (red circle) as a function of the irradiation time. The experiment was conducted with a 405 nm pulsed laser in MeCN solutions. (B) Relative absorbance values of the DMSO/HEPES buffer solutions (pH 7.3, 7 : 3 v/v) with OLM-17 (red square), Alexa 488 (blue square), and Atto 488 (green square) as a function of the irradiation time. The experiment was performed by using a Xe lamp with a power of 300 W. (C) Plots of the normalized intracellular fluorescence intensity as a function of the number of recorded STED images. The STED images of the fixed HeLa cells were obtained by using the OLM-17 (D) and Alexa 488 (E) antibody conjugates during successive scanning. The scale bar for D and E is 10 μm.142 Reproduced from ref. 142 with permission from John Wiley and Sons. | |
Since mitochondria play great roles in cellular events,143–146 such as apoptosis, Yamaguchi and his co-workers also developed a super-photostable fluorophore, OLM-19.65 In this case, the lipophilic cationic triphenylphosphonium building block and the highly reactive epoxide units were drawn into the naphthophosphole skeleton to acquire mitochondria-specific imaging. Initially, both OLM-19 and MitoTracker Deep Red FM were incubated in the living cells and a high co-localization was obtained for both dyes together with a large Pearson's correlation coefficient, demonstrating that OLM-19 can efficiently stain the mitochondria. Successively, mitochondria-specific imaging via both confocal and STED nanoscopy was conducted: the fine structure of the mitochondrial cristae cannot be recorded via conventional confocal microscopy but is obtained via live-STED imaging with a narrowed FWHM of 60 nm (Fig. 15A and B). This implies that a higher imaging resolution can be obtained with OLM-19 in this case. Interestingly, two-color imaging pictures were also obtained by using OLM-19 and tetramethylrhodamine (TMR) in STED mode. By fully comparing the fluorescent signals of TMR and OLM-19, it can be found that the TMR signals always surround those of OLM-19. This implies that fluorescence by OLM-19 appears inside the TMR-labeled outer mitochondrial membranes. Moreover, due to the high photo-stability and impressive imaging performance in single and two-color STED super-resolution imaging of OLM-19, the mitochondrial dynamics by time-lapse STED imaging was finally achieved to well visualize the rapid inter-cristae merge in single mitochondrion and inter-mitochondrial fusions (Fig. 15E and F). In addition, the long-term tracking of the mitochondrial morphological changes over 90 s was also successfully recorded via live-cell STED imaging (Fig. 15G). This provides an efficient method to obtain detailed information about the dynamic of ultra-structures in mitochondria in several biological processes.
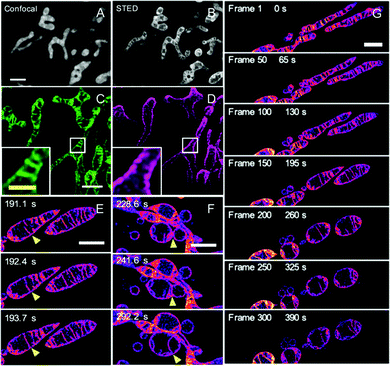 |
| Fig. 15 Confocal (A) and STED (B) imaging of the mitochondria of living HeLa cells stained with OLM-19 (λex = 488 nm; λSTED = 660 nm; scale bar= 2 μm). Two-color STED image of the (C) mitochondrial inner membrane labeled with OLM-19 (λex = 470 nm; green) and the (D) mitochondrial outer membrane labeled with TOMM20-TMR (λex = 540 nm; magenta). (E–G) Time-lapse STED imaging of the mitochondrial dynamics in OLM-19-labeled cells (Scale bar = 2 μm).65 Reproduced from ref. 65 with permission from the Copyright (2019) National Academy of Sciences, USA. | |
4.3.3. Organic metal complexes in STED imaging.
Recently, organic metal complexes147–150 have also been widely applied as imaging agents in cellular applications due to their distinctive coordination geometry. This results in intense charge transfer bands and finely tunable optical properties, such as Stokes' shift, long-living emissive states, and photo-stability. These features in organic metal complexes make them a good alternative to inorganic fluorophores for super-resolution imaging in STED nanoscopy. In 2016, Keyes and his co-workers reported two red emitting Ru(II) polypyridyl complexes of OLM-20 and OLM-21 (Scheme 1).151 Both compounds emit well with a high quantum yield due to the triplet metal to ligand charge transfer state (MLCT).152–154 By using OLM-20 and OLM-21, their corresponding ruthenium polypyridyl complex peptide conjugates, Ru-ER and Ru-NLS respectively, were prepared, to be used in organelle-specific STED imaging in living cells. In the case of Ru-ER incubated HeLa cells, an obviously enhanced resolution can be obtained via STED nanoscopy and the tubular structure of the smooth ER can be visualized. However, this cannot be resolved by confocal microscopy. Moreover, Ru-NLS can be used to target the nuclei in HeLa cells, which can also be imaged via STED nanoscopy, finally producing a facile identification of the cell phase by recording the different stages of mitosis with an ultra-high resolution. In another case, a Zn(II) complex (OLM-22) was then developed by Tian et al.155 In OLM-22, thiophene units to extend the electronic delocalization and terpyridine to target mitochondria were attached to its molecular backbone. This results in a strong metal–ligand charge transfer and an enhancement of its optical properties. Interestingly, only fibril patterns in the mitochondria structure were observed via conventional confocal micrography. However, when OLM-22 stained cells were detected by STED nanoscopy, high signal-to-noise ratio (>10, estimated by the fluorescence intensity in targets and backgrounds) revealing the folded crista structures was successfully obtained. This shows the great possibility of using organic metal complexes, such as OLM-22, in super-resolution imaging to obtain detailed information in cellular research. Although several studies have been reported on the use of organic metal complexes for super-resolution imaging, only a few probes with multifunctional imaging modalities, such as in STED nanoscopy and structured illumination microscopy (SIM),18,156,157 were developed. Thomas et al. prepared a di-nuclear Ru(II) complex of OLM-23 with a strong emission in the near-infrared window and a high cell membrane permeability to perform cellular imaging at high resolution.158 It is interesting to note that the subcellular localization of OLM-23 is concentration dependent. The complex is mainly localized in the mitochondria at low concentration, but it migrates to the nuclear DNA at concentrations higher than ∼20 μM. Furthermore, OLM-23 also exhibits excellent photo-stability and a large Stokes' shift, well meeting the requirements in both STED and SIM microscopy. Its use leads to high resolution imaging in two colors, 2C-SIM, STED, and 3D-STED for both mitochondria and nuclear chromatin.
4.3.4. Other reported organic fluorophores for STED imaging.
In this section, other previously reported organic fluorophores, such as those containing pyrene, naphthalimide, and coumarin building blocks, are discussed. Their chemical structures (OLM-24–OLM-27) are shown in Scheme 1. Among them, a mega-Stokes OLM-24 with a pyrene unit that was utilized for locating lipid droplets (LDs) in living cells by STED nanoscopy was reported by Keyes et al.159Via passive diffusion, OLM-24 can be found close to lipid droplets and appeared as bright spots in TNF-α treated cells. This result was demonstrated by co-incubating it with the commercial lipid droplet dye of Nile Red (Fig. 16A and B). Moreover, this dye also exhibited excellent emission properties with a photo-luminescence spectrum in the 450–700 nm range (Table 1). Due to its PL emission and large Stokes' shift, the performance of OLM-24 as a STED nanoscopy probe was also examined by using a 660 nm STED beam (Table 1). Fig. 16(D–H) display the confocal and STED images of OLM-24 in living HeLa cells. One can observe that in confocal microscopy an individual and bright LD with a size of 0.864 μm can be detected, whereas via STED nanoscopy, this object is then distinguished into obvious LDs with sizes of 0.339, 0.385, and 0.319 μm, respectively. The recorded plot profile further indicates the significantly enhanced imaging resolution of the LDs via STED (Fig. 16I). The photo-stability of OLM-24 was also investigated and 70% of the fluorescence signal can be retained after continuous irradiation for 20 minutes. However, in the case of Nile Red, only 40% of the fluorescence intensity is retained, indicating that the OLM-24 probe is also suitable for the long-term tracking and monitoring of LDs in living cells via STED nanoscopy. Furthermore, similar studies by Yang and Zhou et al. also show well-resolved LDs in cells by using naphthalimide-based OLM-25160 and coumarin-based OLM-26.161 Additionally, several other fluorophores, such as OLM-27,162 can also be applied in super-resolution organelle-specific imaging and dynamic monitoring to detect the interactions between different organelles in biological processes. These studies further demonstrate that organic fluorophores with a high biocompatibility can be used as promising candidates for STED imaging agents in super-resolution imaging in biological research.
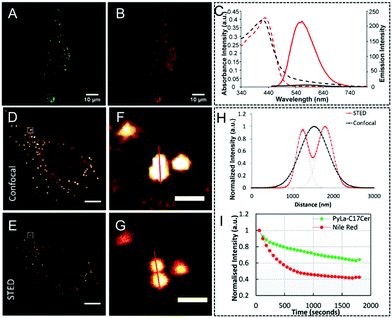 |
| Fig. 16 (A and B) Co-localization of OLM-24 and Nile Red in HeLa cells treated with TNF-α (OLM-24 in green, Nile Red in red). (C) Absorption (dashed line) and fluorescence emission (solid line) spectra of OLM-24 in dichloromethane (red) and CH3CN (black). Confocal (D) and STED (E) image of lipid droplets by using OLM-24 in living HeLa cells (F and G are their corresponding magnified images in D and E). (H) Corresponding plot profiles of the lipid droplets via confocal imaging and STED imaging. (I) Photo-stability of OLM-24 and Nile Red over 20 minutes of continuous STED imaging in living HeLa cells.159 Reproduced from ref. 159 with permission from the Royal Society of Chemistry. | |
4.4. AIE luminogens for STED imaging
For the majority of the aforementioned conventional OLMs, bright luminescent features can be observed in well-dissolved solution states. However, a decrease or even quenching of the emission usually occur in their aggregated states, such as in solids and formed nanoparticles. However, it should be mentioned that most reported organic fluorophores are hydrophobic and when they are used in aqueous media, aggregation and precipitation can easily occur, leading to decreased emission. This may limit their real-world applications in biological research. To achieve ultra-high resolution in STED imaging, the “Aggregation-Caused Quenching (ACQ)” phenomenon163,164 should be prevented to obtain high intensity during the measurements. It is noteworthy that a new concept of “Aggregation-Induced Emission (AIE)” was first proposed by Tang et al. in 2001.165–168 In this case, a completely different phenomenon is observed: inferior emission signals are recorded in solution, but significantly enhanced luminescence properties can be achieved in aggregated states (Fig. 17A and B).168Fig. 17C displays the main mechanism of “Restriction of Intramolecular Motion (RIM)” in AIEgens. This includes the “Restriction of Intramolecular Rotation (RIR)” and the “Restriction of Intramolecular Vibration (RIV)”.169,170 According to the AIE concept and RIM mechanism, tremendous studies were carried out to develop OLMs with AIE characteristics (AIE luminogens, AIEgens) in biological research.171–174 In addition to their high brightness in aggregated states, AIEgens also usually exhibit a large Stokes' shift, a super photostability, and also an excellent biocompatibility. These are the basic requirements for super-resolution imaging by using STED nanoscopy. In this section, several AIEgens applied in STED super-resolution imaging are discussed and their chemical structures are shown in Scheme 2. The photo-physical properties, STED set-up parameters and imaging performance for these AIEgens are listed in Table 2. “AIE” as the abbreviation name together with their original names for these used agents are also included in Table 2.
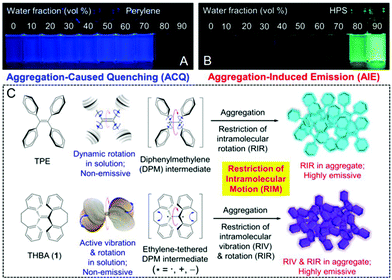 |
| Fig. 17 Fluorescence photographs of perylene (A) and HPS (B) in THF/water mixtures with different water fractions. (C) Schematic illustration of the RIM mechanism in the developed AIEgens.168 Reproduced from ref. 168 with permission from the ACS Publications. | |
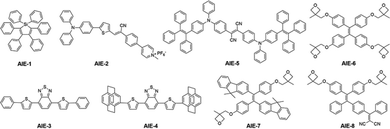 |
| Scheme 2 Chemical structures of AIEgens (AIE-1–AIE-8) for STED super-resolution imaging. | |
In 2015, Qian, Tang and He et al. investigated the potential of AIEgens in STED nanoscopy.47 In this case, the hexaphenylsilole (HPS) molecule was employed as AIE-1 and the commonly used organic fluorophore Coumarin 102 was included as the reference. As displayed in Fig. 18, similar absorption and emission spectra were observed for AIE-1 and Coumarin 102. Due to their similar emission spectra, a similar STED beam was used to investigate their photo-bleaching resistance and emission depletion efficiency in STED nanoscopy. As observed, although the concentration of both dyes is identical and the STED set-up parameters are similar, a severe photo-bleaching occurs in Coumarin 102. However, reversible emission signals can be observed in AIE-1 for a lower photo-bleaching effect. Coumarin 102 exhibits strong π–π stacking interactions due to its planar structure, and thus a non-radiative decay could occur quickly after the excitation and generate thermal energy. At this point, the de-structured Coumarin 102 generates fluorescence bleaching. However, this process can be well prevented by AIE-1 due to its propeller-like nonplanar structure. This pioneering work demonstrates that for AIEgens, such as AIE-1, a high resistance to photo-bleaching and high emission depletion in the STED process can be obtained. This paves the ways for their use in high resolution imaging using STED nanoscopy.
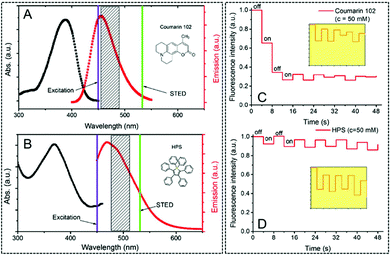 |
| Fig. 18 Absorption (black curve) and emission spectra (red curve) of Coumarin 102 (A) and AIE-1 (B) (purple line: excitation laser; green line: STED laser). Photo-bleaching comparison between Coumarin 102 (C) and AIE-1 (D) excited with a 25 mW STED beam ([c] = 50 mM).47 Reprinted with permission from ref. 47 published in The Optical Society. | |
A red and NIR-fluorescent molecule of AIE-2 was also prepared by Qian and his co-workers in 2018.48AIE-2 exhibits weak emission signals in a dimethyl sulfoxide (DMSO) solution. However, upon the gradual addition of poor solvent to form nanoaggregates, an intensive fluorescence emission can be recorded (Fig. 19A), leading to a high αAIE index of 42 (Fig. 19B). According to the absorption (479 nm) and emission peaks (684 nm), a Stokes' shift of ∼200 nm was recorded for AIE-2 (Table 2). This large value avoids the re-excitation process during the STED beam excitation. By incubating AIE-2 and a mitochondrion-targeting tracker (MitoTracker Deep Red FM), AIE-2 can also be used as a specific mitochondrion-specific probe (Fig. 19C and D). The super-resolution imaging of AIE-2-based mitochondria by using a 480 nm laser as an excitation source and a 775 nm depletion laser as the STED beam can then be conducted (Table 2). Fig. 19E and F display the acquired images in AIE-2 stained cells in confocal mode and STED mode, respectively. Circular and filiform morphologies can be well-distinguished in the STED case, implying that a much higher resolution (∼92.5 nm) can be obtained than in the case of confocal microscopy (∼248.2 nm). By taking advantage of these features and of the well-resolved images of AIE-2 in single cells, the normal mitochondrial activities, such as fission and fusion, and even smaller changes in the mitochondrial morphology can be well recorded with a high resolution of 74.37 nm (Fig. 19G–N and Table 2).
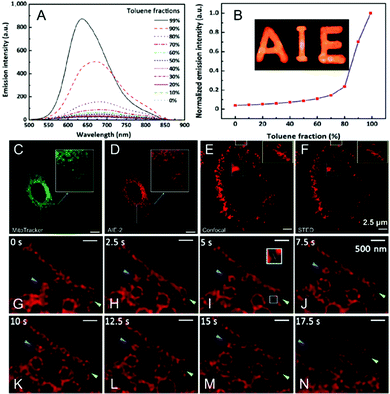 |
| Fig. 19 (A) Emission spectra and (B) plot of the peak intensity for AIE-2 in DMSO/toluene mixtures with various toluene fractions (the inset shows a fluorescence image of AIE-2 in the solid state under 365 nm UV light). Confocal microscopy images of HeLa cells stained with (C) MitoTracker (50 nM) and (D) AIE-2 (5 μM). Confocal microscopy image (E) and STED nanoscopy image (F) of AIE-2-stained mitochondria in a living cell. (G–N) Mitochondrial motion (blue arrowheads) and morphological changes (green arrowheads) captured by STED nanoscopy with a time interval of 2.5 s.48 Reproduced from ref. 48 with permission from Springer Nature. | |
In addition to the application in biological imaging, our group reported an electron-donor- and acceptor-based AIE-4 for the visualization of self-assembling helical fibers with a high-resolution.175 As outlined in Scheme 2, the molecular skeleton of AIE-4 mainly contains two parts: one is the planar thiophene benzothiadiazole core, which produces intermolecular interactions to drive the self-assembly process of the helical fibers; the other is the paracyclophane terminus with a large steric hindrance to control the π–π stacking in aggregated states. According to the molecular design in AIE-4, an “aggregation-fusion-transition” process in a THF/water mixture can be performed to undergo a self-assembling morphology, which passes from vesicles to coalesced vesicles, and finally converts to helical fibers (Fig. 20A–C). The cryo-TEM images in Fig. 20D and E further confirm the self-assembling process of the helixes in AIE-4. Furthermore, AIE-4 exhibits a deep-red emission covering the 500–800 nm range, indicating that a commercial 775 nm depletion beam can be used in STED nanoscopy (Table 2). Encouragingly, a high PLQY value approaching 25%, a Stokes’ shift of 160 nm, and also a superior photo-stability over long-term irradiation in the aggregate state can also be observed in AIE-4.175 These are all the key requirements for STED nanoscopy. Therefore, the formed vesicles and helical fibers were then imaged via STED nanoscopy. In the first vesicles case, an indistinct membrane with small-sized nanoholes (180 nm) was captured via confocal microscopy by using AIE-4.175 However, the fluorescence boundary of the vesicle membrane can be clearly observed via STED nanoscopy: large-sized holes of 430 nm (Fig. 20H–J) can be detected. Similar to the vesicles, an inferior imaging quality was also recorded via confocal microscopy. However, a significantly enhanced resolution was achieved in the self-assembling process of helical fibers (dash lines) via STED nanoscopy at ultra-high resolution. The fiber shows a width of 184 nm and an FWHM of only 178 nm (Fig. 20K–M and Table 2). The 3D STED imaging at an ultra-high resolution and dynamic monitoring of the assembly procedure were also obtained, suggesting that the AIEgens can be good candidates for real-time, in situ visualization and tracking of their assembled nanostructures by using STED nanoscopy.
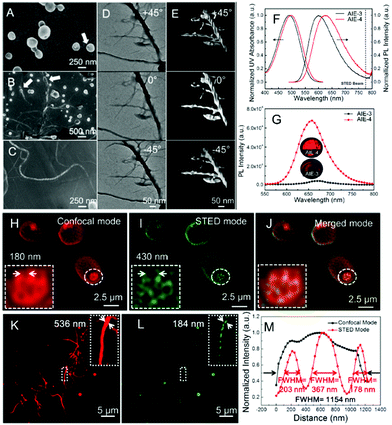 |
| Fig. 20 (A–C) Time-dependent SEM images (A: 0 h, arrow: nanoholes; B: 12 h, arrow: coalesced vesicles; C: 24 h) showing the self-assembly process of AIE-4 in a THF/water mixture. (D) Cryo-TEM images of the self-assembling process of helical fibers caused by AIE-4 (top view: 0°; and side view: −45° and +45°) and (E) their corresponding 3D reconstruction (top view: 0°; and side view: −45° and +45°). (F) Normalized UV absorption and PL spectra of AIE-3 and AIE-4 in a THF solution. (G) PL spectra of AIE-3 and AIE-4 in solids. The inset shows the images of their fluorescence powder. Self-assembling process of the vesicles of AIE-4 captured by (H) confocal microscopy, (I) STED nanoscopy, and (J) their corresponding merged images. Fluorescence images of the AIE-4 helical fibers captured by (K) confocal microscopy, (L) STED nanoscopy under optimized conditions, and (M) their corresponding fluorescence intensity profiles with calculated FWHM along the fibers.175 Reproduced from ref. 175 with permission from ACS Publications. | |
In addition to these organic fluorophores with AIE features, AIE nanoparticles have also been recently developed and applied in STED super-resolution imaging.49,50 For example, by encapsulating them with colloidal mesoporous silica, AIE-5-based fluorescent NPs were produced by Qian and his co-workers (Fig. 21A).49 Then, upon the photoexcitation of AIE-5@SiO2-NPs (594 nm), a red/NIR emission in the 500–900 nm range was obtained (Fig. 21B and Table 2) with a Stokes' shift of 150 nm. Then, by using a 775 nm STED beam (Table 2), a high depletion efficiency and a high photo-stability were observed (Fig. 21C, D and Table 2). This provides a lateral resolution of ∼30 nm for the NPs via STED nanoscopy (Fig. 21E and F). This value is on the verge of their real particle size (24.3 nm). More importantly, super-resolution imaging in HeLa cells by incubating AIE-5@SiO2-NPs was also performed (Fig. 21G and H). It can be observed that in confocal microscopy, only the fluorescence spots with a large size can be imaged and it is difficult to distinguish the closely spaced NPs. However, as anticipated, clearer fluorescence spots can be easily achieved via STED nanoscopy by using AIE-5-contained NPs, resulting in an ultra-high lateral resolution of ∼30 nm (Table 2). Furthermore, such developed AIE nanoparticles offer an efficient method to perform long-term imaging and monitoring in living cells. Wu and Sun et al. reported AIEgen-based nanodots for subcellular imaging via STED nanoscopy.50 As shown in Scheme 2, oxetane-substituted AIE-6–AIE-8 were chosen for their tunable blue, green, and red emission, respectively. Moreover, due to their promising AIE features, an intense emission with the PLQYs of up to 69%, 63%, and 57%, was also observed in AIE-6, AIE-7, and AIE-8, respectively (Fig. 22A). Upon modified nanoprecipitation and photo-crosslinking, ultra-stable AIE dots were prepared for streptavidin bio-conjugation (Fig. 22B). Finally, such AIE dots with small size, a good stability, and surface functionalization were applied in super-resolution imaging. Fig. 22C and D illustrate the morphology of the subcellular microtubule structures by using red-emitted AIE dots (AIE-8) in both confocal mode and STED mode. Obviously, a higher imaging performance can be obtained via STED nanoscopy with a significantly enhanced FWHM of ∼95 nm (Table 2), whereas in the case of confocal microscopy, the FWHM only measures 200 nm.
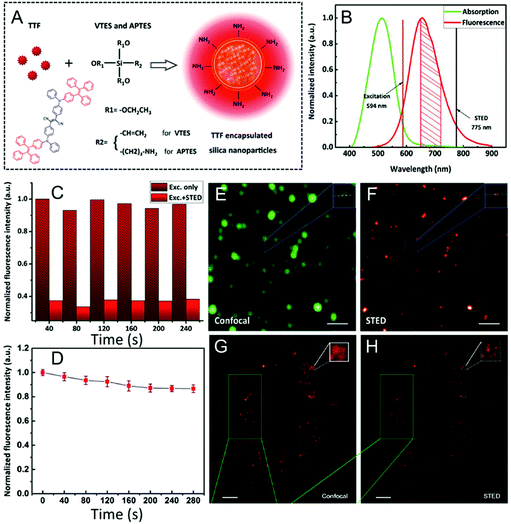 |
| Fig. 21 (A) Synthesis illustration of AIE-5@SiO2 NPs. (B) Absorption and fluorescence spectra of AIE-5@SiO2 NPs. (C) Normalized fluorescence intensities of AIE-5@SiO2 NPs irradiated by a 594 nm excitation laser with and without a STED laser (775 nm, 250 mW). (D) Normalized fluorescence intensity of AIE-5@SiO2 NPs at various time points during 280 s-successive scanning by an excitation beam (594 nm) and STED beam (775 nm). Fluorescence images of AIE-5@SiO2 NPs by confocal (E) and STED nanoscopy (F) by a 594 nm excitation laser (2.5 mW) and a 775 nm STED laser (312.5 mW). The scale bar in E and F is 1 μm. Confocal (G) and STED nanoscopy (H) imaging of AIE-5@SiO2 NP stained HeLa cells. The scale bar in G and H is 3 μm.49 Reproduced from ref. 49 with permission from the John Wiley and Sons. | |
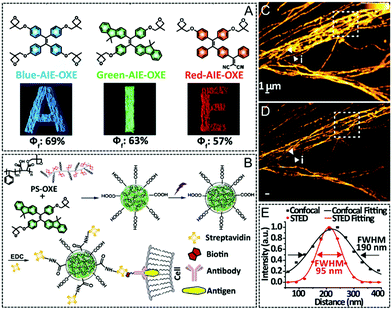 |
| Fig. 22 (A) Chemical structures of AIE-6, AIE-7, and AIE-8 and their corresponding fluorescence images in solid states by 365 nm UV light. (B) Schematic illustration to prepare small AIE dots via photo-crosslinking. Confocal (C) and STED (D) images of the microtubule structures labeled via the AIE-8 nanodots. (E) Line profile of the position indicated by the arrow heads (i) in C and D. The scale bar measures 1 μm.50 Reproduced from ref. 50 with permission from the John Wiley and Sons. | |
4.5. Fluorescent nanoparticles for STED imaging
In addition to the tremendous efforts in developing photo-stable fluorophores, fluorescent nanoparticles have also been applied in STED super-resolution imaging recently.117,176 This greatly enriched the toolbox of STED agents for super-resolution biological imaging. Among them, inorganic nanoparticles, such as QDs and UCNPs, were discussed in the inorganic fluorophores section, whereas the use of AIE nanoparticles or AIE dots was described in the AIE section. This section only focuses on fluorescent NPs used with conventional organic dyes and polymeric fluorophores. Fang et al. developed highly emissive polymeric dots (Pdots) by using a semiconducting polymer based on PDFDP in 2018.66 Successively, bio-conjugation was achieved to produce biotin-labeled Pdots. The chemical structure of PDFDP and a schematic illustration to prepare them via an optimized nanoprecipitation process are displayed in Fig. 23A. Both Pdots and biotin-Pdots exhibit a monodispersed spherical morphology with a size of ∼40 nm (Fig. 23B). Moreover, a superior photo-stability can also be observed, indicating their potential in STED cellular imaging. A series of typical confocal and STED images are displayed in Fig. 23C and D: fluorescence spots with a smaller size can be recorded via STED nanoscopy (10 MW cm−2) when compared to confocal microscopy, leading to a higher improvement in the spatial resolution (71 nm). Moreover, biotin-Pdots were incubated in cancerous HeLa cells and imaged via STED nanoscopy. As displayed, the high signal-to-noise ratio and ultra-resolution images of Pdot-stained HeLa cells show that it is possible to distinguish closely spaced particles in a STED case with a 77 nm resolution. In contrast to the emission-quenched bioti-Atto565 after 1 h irradiation, the fluorescence intensity of Pdots only decreases by 30% after 2 h of continuous imaging, demonstrating its potential in long-term STED cellular imaging. In another case,79 by using a nonplanar compound (DAPF) to prevent the intermolecular π–π stacking and also the formation of excimer. Fu and his co-workers prepared silica-coated core–shell nanoparticles (CSONPs) with highly emissive features in solids, a large Stokes' shift measuring up to 150 nm, and also a superior photo-stability. Interestingly, an impressive depletion efficiency of up to 99% via the use of a STED beam at low saturation intensity (0.18 MW cm−2) was also recorded in this case. Finally, an ultra-high imaging resolution of ∼60 nm can be achieved via STED nanoscopy with a low depletion power of 0.89 MW cm−2. More importantly, due to these advantages of the CSONPs, super-resolution imaging for living HeLa cells was also conducted. The findings show a high resolution of 63 nm and a long-term photo-stability over 600 s.
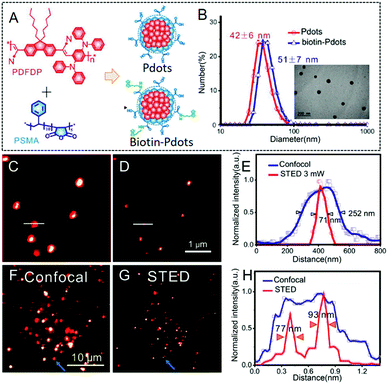 |
| Fig. 23 (A) Schematic illustration of the preparation procedure of the PDFDP-based Pdots and biotin-Pdots. (B) DLS measurement and TEM image of the Pdots. Confocal (C) and STED (D) image of the PDFDP-based Pdots. (E) Intensity profiles with calculated FWHMs along the white lines in figure C and D. HeLa cell imaging via biotin-Pdots by using confocal (F) and STED (G) microscopy. (H) Intensity profiles with calculated FWHMs along the white lines in figure F and G.66 Reproduced from ref. 66 with permission from the John Wiley and Sons. | |
Recently, our group also developed fluorescent organic nanoparticles for STED super-resolution imaging by using the organic fluorophore of DBTBT-4C8.55 It is noted that via an alkyl chain engineering process in DBTBT-4C8, a high brightness with PLQY of 25% in the aggregated state was obtained. Moreover, nanoparticles with a uniform and well-defined spherical morphology were produced (Fig. 24A). By using the deep-red emission to match the commercial 775 nm beam (Fig. 24B), high resistance to photo-bleaching (Fig. 24C), excellent biocompatibility and STED super-resolution imaging can be easily obtained for HeLa cells. In this case, only the bright spots were imaged via confocal microscopy (Fig. 24D and E), but detailed fluorescence signals can be obtained by using STED nanoscopy, finally leading to a significant improvement in the FWHM of 100 nm and 170 nm (Fig. 24F). Furthermore, 3D STED imaging was also successfully carried out here by using the fluorescent NPs by a layer-by-layer record and reconstruction process (Fig. 24G–J). The corresponding estimated volumes reported in Fig. 24K and L further confirm the improved imaging resolution via STED nanoscopy. This result further demonstrates that the incorporation of fluorescence nanoparticles with STED nanoscopy can open new avenues for future super-resolution imaging.
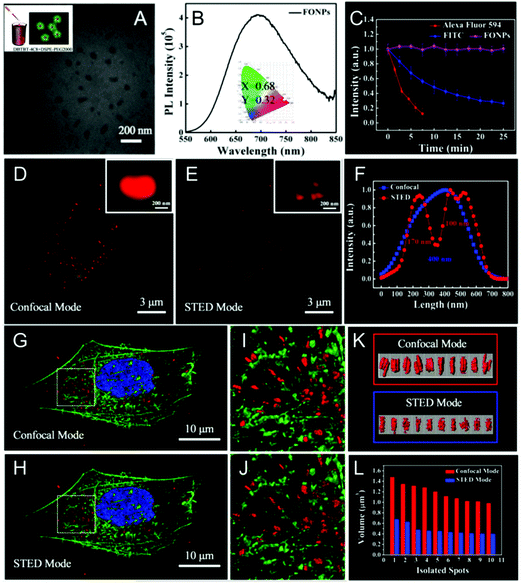 |
| Fig. 24 (A) Schematic illustration and TEM image of the fluorescence nanoparticles based on DBTBT-4C8 via the nano-precipitation method. (B) PL spectra of the DBTBT-4C8-based nanoparticles. The inset shows the corresponding chromaticity coordinates. (C) Attenuation of the fluorescence for Alexa Fluor 594, FITC, and DBTBT-4C8 nanoparticle-containing HeLa cells under a STED laser irradiation of 600 nm. Images of the DBTBT-4C8-based nanoparticles in HeLa cells acquired via confocal (D) and STED nanoscopy (E). (F) Their corresponding cross section files with FWHM in the magnified image in D and E. 3D reconstructed images of the DAPI (blue), Phalloidin (green), and DBTBT-4C8 nanoparticle (red) stained HeLa cells via confocal (G) and STED nanoscopy (H) together with their corresponding magnified images (I and J). 3D reconstructed fluorescence nanoparticles in HeLa cells obtained by confocal and STED nanoscopy (K) together with their corresponding estimated volumes (L).55 Reproduced from ref. 55 with permission from ACS Publications. | |
5. Conclusions and prospects
In summary, the recent progress on fluorophores in STED nanoscopy, including inorganic fluorophores, fluorescent proteins, organic luminescence materials, AIE luminogens, and fluorescence nanoparticles, are here discussed, due to their important role in super-resolution imaging. According to the results reported in this review, the following statements can be drawn: (1) in addition to their superior photo-stability, ultra-high resolution can be easily obtained for inorganic fluorophores in STED nanoscopy; (2) organic fluorophores usually exhibit a good chemical tailorability to finely tune their luminescence properties, leading to an optimized imaging performance in STED nanoscopy. Moreover, an excellent biocompatibility can be easily obtained in this case. Among them, rhodamine-based fluorophores, which are able to efficiently label biological targets, are widely reported for super-resolution imaging and dynamic monitoring in biological research. (3) Other promising STED fluorophores are AIE luminogens, due to their high brightness in aggregates, superior photo-stability, and large Stokes' shifts. These advantages are the key requirements for STED imaging at ultra-high resolution. However, it is noted that although significant achievements have been obtained in the development of luminescent materials for super-resolution imaging via STED nanoscopy, the following aspects in this field should be taken under consideration for future research: (1) despite the high resolution achieved by using inorganic fluorophores, new methods to prepare nanoparticles at a large scale with high quality and reproducibility should be developed to meet the requirements of STED nanoscopy; (2) only a few fluorophores are commercially available and unfortunately, some of these dyes suffer from several drawbacks, such as high cost, poor photo-stability, and limited Stokes' shift; (3) suitable organic fluorophores and fluorescent nanoparticles for super-resolution biological imaging are still limited, despite the success of rhodamine-based fluorophores. Moreover, STED imaging is currently not only limited to conventional imaging; more and more requirements exist to perform different multi-functional STED imaging, such as multi-color STED imaging, time-lapse 3D STED imaging, deep STED imaging, and STED imaging combined with other techniques (T-REX STED, LocSTED and RESCue-STED to reduce the state transition cycles). Therefore, more efforts should be devoted to developing new fluorophores for multi-functional STED imaging in biological research; and (4) although AIEgens are promising candidates to be used in STED super-resolution imaging, the majority of the reported AIEgens are hydrophobic. This limits their bio-conjugation ability in targeting a specific objective in biomedical research. From this aspect, water-soluble AIEgens with different functional groups, which can efficiently target biological complexes, show great potential to be employed in super-resolution biological imaging. Considering that luminescence materials suitable for STED imaging are not often investigated, it's believed that their further exploration in the future may provide a deeper understanding of the relation among luminescent materials and their STED imaging performance. More detailed guidelines could also be anticipated in selecting and even developing new fluorophores for STED super-resolution imaging, finally providing a tool to “see the forest tree by tree” in both materials science and biological research.
Conflicts of interest
There are no conflicts to declare.
Acknowledgements
The authors are thankful for the financial support from the National Natural Science Foundation of China (21975197, 21674085 and 51603165), The Young Talent Fund of University Association for Science and Technology in Shaanxi, China (20180601), the Natural Science Basic Research Plan in Shaanxi Province of China (2019JM-040), the Key Laboratory Construction Program of Xi'an Municipal Bureau of Science and Technology (201805056ZD7CG40), and the Fundamental Fund of Xi'an Jiao Tong University (xzy022020015). This work was also supported by Innovation Capability Support Program of Shaanxi (No. 2018PT-28 and 2019PT-05).
Notes and references
- M. J. Booth, Light: Sci. Appl., 2014, 3, e165 CrossRef.
- S. W. Hell, Abstr. Pap., Jt. Conf. – Chem. Inst. Can. Am. Chem. Soc., 2009, 238, 20 Search PubMed -PHYS.
- J. Ryan, A. R. Gerhold, V. Boudreau, L. Smith and P. S. Maddox, Light Microscopy: Methods and Protocols, 2017, 1563, 1, DOI:10.1007/978-1-4939-6810-7_1.
- F. Chen, P. W. Tillberg and E. S. Boyden, Science, 2015, 347, 543 CrossRef CAS.
- K. W. Dunn, M. M. Kamocka and J. H. McDonald, Am. J. Physiol.: Cell Physiol., 2011, 300, C723 CrossRef CAS.
- S. W. Paddock, Biotechniques, 1999, 27, 992 CrossRef CAS.
- A. Fine, W. B. Amos, R. M. Durbin and P. A. McNaughton, Trends Neurosci., 1988, 11, 346 CrossRef CAS.
- H. Shuman, J. M. Murray and C. Dilullo, Biotechniques, 1989, 7, 154–163 CAS.
- P. T. C. So, C. Y. Dong, B. R. Masters and K. M. Berland, Annu. Rev. Biomed. Eng., 2000, 02, 399 CrossRef CAS.
- E. E. Hoover and J. A. Squier, Nat. Photonics, 2013, 7, 93 CrossRef CAS.
- W. J. Yang and R. Yuste, Nat. Methods, 2017, 14, 349 CrossRef CAS.
- K. Konig, J. Microsc., 2000, 200, 83 CrossRef CAS.
- S. W. Hell and J. Wichmann, Opt. Lett., 1994, 19, 780 CrossRef CAS.
- S. W. Hell, Science, 2007, 316, 1153 CrossRef CAS.
- A. H. Zewail, Science, 2010, 328, 187 CrossRef CAS.
- Y. M. Sigal, R. Zhou and X. Zhuang, Science, 2018, 361, 880 CrossRef CAS.
- C. Errico, J. Pierre, S. Pezet, Y. Desailly, Z. Lenkei, O. Couture and M. Tanter, Nature, 2015, 527, 499 CrossRef CAS.
- L. Schermelleh, A. Ferrand, T. Huser, C. Eggeling, M. Sauer, O. Biehlmaier and G. P. C. Drummen, Nat. Cell Biol., 2019, 21, 72 CrossRef CAS.
- E. A. Ash and G. Nicholls, Nature, 1972, 237, 510 CrossRef CAS.
- H. Deschout, F. C. Zanacchi, M. Mlodzianoski, A. Diaspro, J. Bewersdorf, S. T. Hess and K. Braeckmans, Nat. Methods, 2014, 11, 253 CrossRef CAS.
- H. Shen, L. J. Tauzin, R. Baiyasi, W. X. Wang, N. Moringo, B. Shuang and C. F. Landes, Chem. Rev., 2017, 117, 7331 CrossRef CAS.
- E. Betzig, G. H. Patterson, R. Sougrat, O. W. Lindwasser, S. Olenych, J. S. Bonifacino, M. W. Davidson, J. L. Schwartz and H. F. Hess, Science, 2006, 313, 1642 CrossRef CAS.
- C. Errico, J. Pierre, S. Pezet, Y. Desailly, Z. Lenkei, O. Couture and M. Tanter, Nature, 2015, 527, 499 CrossRef CAS.
- S. A. Jones, S. H. Shim, J. He and X. W. Zhuang, Nat. Methods, 2011, 8, 499 CrossRef CAS.
- B. Huang, W. Wang, M. Bates and X. Zhuang, Science, 2008, 319, 810 CrossRef CAS.
- M. J. Rust, M. Bates and X. W. Zhuang, Nat. Methods, 2006, 3, 793 CrossRef CAS.
- T. Dertingera, R. Colyera, G. Iyera, S. Weissa and J. Enderlein, Proc. Natl. Acad. Sci. U. S. A., 2009, 106, 22287 CrossRef.
- Z. P. Zeng, X. Z. Chen, H. N. Wang, N. Huang, C. Y. Shan, H. Zhang, J. L. Teng and P. Xi, Sci. Rep., 2015, 5, 8359 CrossRef CAS.
- S. Geissbuehler, A. Sharipov, A. Godinat, N. L. Bocchio, P. A. Sandoz, A. Huss, N. A. Jensen, S. Jakobs, J. Enderlein, F. G. van der Goot, E. A. Dubikovskaya, T. Lasser and M. Leutenegger, Nat. Commun., 2014, 5, 5830 CrossRef.
- B. Huang, H. Babcock and X. Zhuang, Cell, 2010, 143, 1047 CrossRef CAS.
- T. A. Klar and S. W. Hell, Opt. Lett., 1999, 24, 954 CrossRef CAS.
- H. Blom and J. Widengren, Chem. Rev., 2017, 117, 7377 CrossRef CAS.
- G. Vicidomini, P. Bianchini and A. Diaspro, Nat. Methods, 2018, 15, 173 CrossRef CAS.
- T. Muller, C. Schumann and A. Kraegeloh, ChemPhysChem, 2012, 13, 1986 CrossRef.
- L. Schermelleh, A. Ferrand, T. Huser, C. Eggeling, M. Sauer, O. Biehlmaier and G. P. C. Drummen, Nat. Cell Biol., 2019, 21, 72 CrossRef CAS.
- B. Neupane, F. S. Ligler and G. Wang, J. Biomed. Opt., 2014, 19, 080901 CrossRef.
- J. Angibaud, P. Mascalchi, C. Poujol and U. V. Nägerl, J. Phys. D: Appl. Phys., 2020, 53, 184001 CrossRef CAS.
- L. Wang, W. Yan, R. Li, X. Weng, J. Zhang, Z. Yang, L. Liu, T. Ye and J. Qu, Nanophotonics, 2018, 7, 1971 Search PubMed.
- S. Ye, J. Guo, J. Song and J. Qu, Appl. Phys. Lett., 2020, 116, 041101 CrossRef CAS.
- X. W. Hua, Y. W. Bao, J. Zeng and F. G. Wu, ACS Appl. Mater. Interfaces, 2019, 11, 32647 CrossRef CAS.
- Q. Zhan, H. Liu, B. Wang, Q. Wu, R. Pu, C. Zhou, B. Huang, X. Peng, H. Ågren and S. He, Nat. Commun., 2017, 8, 1058 CrossRef.
- R. Wu, Q. Zhan, H. Liu, X. Wen, B. Wang and S. He, Opt. Express, 2015, 23, 32401 CrossRef CAS.
- D. Nair, E. Hosy, J. D. Petersen, A. Constals, G. Giannone, D. Choquet and J. B. Sibarita, J. Neurosci., 2013, 33, 13204 CrossRef CAS.
- G. Dumbovića, X. Sanjuan, M. Perucho and S. V. Forcales, Methods DOI:10.1016/j.ymeth.2020.04.009.
- Z. Yang, A. Sharma, J. Qi, X. Peng, D. Y. Lee, R. Hu, D. Lin, J. Qu and J. S. Kim, Chem. Soc. Rev., 2016, 45, 4651 RSC.
- Z. Liu and C. Wu, Chin. Opt., 2018, 11, 344 CrossRef.
- J. Yu, X. Sun, F. Cai, Z. Zhu, A. Qin, J. Qian, B. Tang and S. He, Opt. Lett., 2015, 40, 2313 CrossRef CAS.
- D. Y. Li, X. Ni, X. Y. Zhang, L. W. Liu, J. L. Qu, D. Ding and J. Qian, Nano Res., 2018, 11, 6023 CrossRef CAS.
- D. Y. Li, W. Qin, B. Xu, J. Qian and B. Z. Tang, Adv. Mater., 2017, 29, 1703643 CrossRef.
- X. F. Fang, X. Z. Chen, R. Q. Li, Z. H. Liu, H. B. Chen, Z. Z. Sun, B. Ju, Y. F. Liu, S. X. A. Zhang, D. Ding, Y. J. Sun and C. F. Wu, Small, 2017, 13, 1702128 CrossRef.
- G. Vicidomini, A. Schonle, H. S. Ta, K. Y. Han, G. Moneron, C. Eggeling and S. W. Hell, PLoS One, 2013, 8, e54421 CrossRef CAS.
- P. Bianchini, C. Peres, M. Oneto, S. Galiani, G. Vicidomini and A. Diaspro, Cell Tissue Res., 2015, 360, 143 CrossRef CAS.
- L. W. Wang, Y. Chen, X. Peng, J. Zhang, J. L. Wang, L. W. Liu, Z. G. Yang, W. Yan and J. L. Qu, Nanophotonics, 2020, 9, 831 CAS.
- Y. M. Sigal, R. B. Zhou and X. W. Zhuang, Science, 2018, 361, 880 CrossRef CAS.
- Y. Z. Xu, H. K. Zhang, N. Zhang, X. C. Wang, D. F. Dang, X. N. Jing, D. Xi, Y. Hao, B. Z. Tang and L. J. Meng, ACS Appl. Mater. Interfaces, 2020, 12, 6814 CrossRef CAS.
- I. Vojnovic, J. Winkelmeier and U. Endesfelder, Biochem. Soc. Trans., 2019, 47, 1041 CrossRef CAS.
- J. Wang, J. Zhang, L. Wang, X. Gao, Y. Shao, L. Liu, Z. Yang, W. Yan and J. Qu, J. Biophotonics, 2020, 13, e202000057, DOI:10.1002/jbio.202000057.
- C. Spahn, J. B. Grimm, L. D. Lavis, M. Lampe and M. Heilemann, Nano Lett., 2019, 19, 500 CrossRef CAS.
- W. Becker, J. Microsc., 2012, 247, 119 CrossRef CAS.
- M. D. Lesoine, S. Bose, J. W. Petrich and E. A. Smith, J. Phys. Chem. B, 2012, 116, 7821 CrossRef CAS.
- C. Li, S. Liu, W. Wang, W. Liu, C. Kuang and X. Liu, J. Microsc., 2018, 271, 4 CrossRef CAS.
- S. Puthukodan, E. Murtezi, J. Jacak and T. A. Klar, Nanophotonics, 2020, 9, 783 CAS.
- Q. C. Yao, L. J. Li, X. S. Huang, H. D. Li, Y. Y. Fang, J. Xia, J. L. Fan, L. Y. Chen, J. Y. Wang and X. J. Peng, Anal. Chem., 2019, 91, 15777 CrossRef CAS.
- R. Wirth, P. Gao, G. U. Nienhaus, M. Sunbul and A. Jaschke, J. Am. Chem. Soc., 2019, 141, 7562 CrossRef CAS.
- C. G. Wang, M. Taki, Y. Sato, Y. Tamura, H. Yaginuma, Y. Okada and S. Yamaguchi, Proc. Natl. Acad. Sci. U. S. A., 2019, 116, 15817 CrossRef CAS.
- Y. Y. Wu, H. F. Ruan, R. Zhao, Z. Z. Dong, W. H. Li, X. J. Tang, J. H. Yuan and X. H. Fang, Adv. Opt. Mater., 2018, 6, 1800333 CrossRef.
- C. G. Wang, M. Taki, Y. Sato, A. Fukazawa, T. Higashiyama and S. Yamaguchi, J. Am. Chem. Soc., 2017, 139, 10374 CrossRef CAS.
- J. Hanne, H. J. Falk, F. Gorlitz, P. Hoyer, J. Engelhardt, S. J. Sahl and S. W. Hell, Nat. Commun., 2015, 6, 7127 CrossRef CAS.
- H. Li, S. Ye, J. Guo, H. Wang, W. Yan, J. Song and J. Qu, Nano Res., 2019, 12, 3075 CrossRef CAS.
- Y. Liu, Y. Lu, X. Yang, X. Zheng, S. Wen, F. Wang, X. Vidal, J. Zhao, D. Liu, Z. Zhou, C. Ma, J. Zhou, J. A. Piper, P. Xi and D. Jin, Nature, 2017, 543, 229 CrossRef CAS.
- B. B. Neupane and G. Wang, Eng. Res. Express, 2020, 2, 015035 CrossRef.
- S. Krause, M. Baldtzer Liisberg, S. Lahtinen, T. Soukka and T. Vosch, ACS Appl. Nano Mater., 2019, 2, 5817 CrossRef CAS.
- K. S. Morozova, K. D. Piatkevich, T. J. Gould, J. Zhang, J. Bewersdorf and V. V. Verkhusha, Biophys. J., 2010, 99, L13 CrossRef CAS.
- J. T. Wessels, K. Yamauchi, R. M. Hoffman and F. S. Wouters, Cytometry, Part A, 2010, 77A, 667 CrossRef CAS.
- W. Wegner, P. Ilgen, C. Gregor, J. V. Dort, A. C. Mott, H. Steffens and K. I. Willig, Sci. Rep., 2017, 7, 11781 CrossRef.
- G. Lukinavičius, G. Y. Mitronova, S. Schnorrenberg, A. N. Butkevich, H. Barthel, V. N. Belov and S. W. Hell, Chem. Sci., 2018, 9, 3324 RSC.
- A. N. Butkevich, G. Yu Mitronova, S. C. Sidenstein, J. L. Klocke, D. Kamin, D. N. H. Meineke, E. D’Este, P. T. Kraemer, J. G. Danzl, V. N. Belov and S. W. Hell, Angew. Chem., Int. Ed., 2016, 55, 3290 CrossRef CAS.
- L. Liang, W. Yan, X. Qin, X. Peng, H. Feng, Y. Wang, Z. Zhu, L. Liu, Y. Han, Q. Xu, J. Qu and X. Liu, Angew. Chem., Int. Ed., 2020, 132, 756 CrossRef.
- Z. Man, Z. Lv, Z. Xu, H. Cui, Q. Liao, L. Zheng, X. Jin, Q. He and H. Fu, Nanoscale, 2019, 11, 12990 RSC.
- M. Collot, T. K. Fam, P. Ashokkumar, O. Faklaris, T. Galli, L. Danglot and A. S. Klymchenko, J. Am. Chem. Soc., 2018, 140, 5401 CrossRef CAS.
- Y. Xu, C. Li, R. Xu, N. Zhang, Z. Wang, X. Jing, Z. Yang, D. Dang, P. Zhang and L. Meng, Chem. Sci., 2020, 11, 8157 RSC.
- G. T. Dempsey, J. C. Vaughan, K. H. Chen, M. Bates and X. Zhuang, Nat. Methods, 2011, 8, 1027 CrossRef CAS.
- W. W. W. Hsiao, Y. Y. Hui, P. C. Tsai and H. C. Chang, Acc. Chem. Res., 2016, 49, 400 CrossRef CAS.
- M. F. Kircher, S. S. Gambhir and J. Grimm, Nat. Rev. Clin. Oncol., 2011, 8, 677 CrossRef CAS.
- M. Z. Zhang, W. Du, X. H. Tian, R. L. Zhang, M. Zhao, H. P. Zhou, Y. Q. Ding, L. Li, J. Y. Wu and Y. P. Tian, J. Mater. Chem. B, 2018, 6, 4417 RSC.
- S. Schuebbe, C. Cavelius, C. Schumann, M. Koch and A. Kraegeloh, Adv. Eng. Mater., 2010, 12, 417 CrossRef CAS.
- R. Sharma, M. Singh and R. Sharma, Spectrochim. Acta, Part A, 2020, 231, 117715 CrossRef CAS.
- X. Yang, Z. Yang, Z. Wu, Y. He, C. Shan, P. Chai, C. Ma, M. Tian, J. Teng, D. Jin, W. Yan, P. Das, J. Qu and P. Xi, Nat. Commun., 2020, 11, 3699 CrossRef CAS.
- G. Lukinavicius, L. Reymond, E. D’Este, A. Masharina, F. Göttfert, H. Ta, A. Güther, M. Fournier, S. Rizzo, H. Waldmann, C. Blaukopf, C. Sommer, D. W. Gerlich, H. D. Arndt, S. W. Hell and K. Johnsson, Nat. Methods, 2014, 11, 731 CrossRef CAS.
- Y. Wang, C. Kuang, Z. Gu, Y. Xu, S. Li, X. Hao and X. Liu, Opt. Eng., 2013, 52, 093107 CrossRef.
- B. Neupane, F. Chen, W. Sun, D. T. Chiu and G. F. Wang, Rev. Sci. Instrum., 2013, 84, 043701 CrossRef.
- K. Kolmakov, V. N. Belov, J. Bierwagen, C. Ringemann, V. Muller, C. Eggeling and S. W. Hell, Chem. – Eur. J., 2010, 16, 158 CrossRef CAS.
- M. Weber, T. A. Khan, L. J. Patalag, M. Bossi, M. Leutenegger, V. N. Belov and S. W. Hell, Chem. – Eur. J., 2020, 6 DOI:10.1002/chem.202004645.
- F. Gottfert, C. A. Wurm, V. Mueller, S. Berning, V. C. Cordes, A. Honigmann and S. W. Hell, Biophys. J., 2013, 105, L01 CrossRef.
- S. Sreedharan, M. R. Gill, E. Garcia, H. K. Saeed, D. Robinson, A. Byrne, A. Cadby, T. E. Keyes, C. Smythe, P. Pellett, J. B. D. L. Serna and J. A. Thomas, J. Am. Chem. Soc., 2017, 139, 15907 CrossRef CAS.
- A. N. Butkevich, G. Lukinavicius, E. D’Este and S. W. Hell, J. Am. Chem. Soc., 2017, 139, 12378 CrossRef CAS.
- M. V. Sednev, V. N. Belov and S. W. Hell, Methods Appl. Fluoresc., 2015, 3, 042004 CrossRef.
- M. Chen, X. He, K. Wang, D. He, X. Yang and H. Shi, TrAC, Trends Anal. Chem., 2014, 58, 120 CrossRef CAS.
- S. Sharma, A. Umar, S. Sood, S. K. Mehta and S. K. Kansal, J. Alloys Compd., 2018, 748, 818 CrossRef CAS.
- Y. B. Liu, L. Zhou, Y. N. Li, R. P. Deng and H. J. Zhang, Nanoscale, 2017, 9, 491 RSC.
- Y. Xu, L. Ren, D. Dang, Y. Zhi, X. Wang and L. Meng, Chem. – Eur. J., 2018, 24, 10383 CrossRef CAS.
- L. Yan, Y. Zhang, B. Xu and W. Tian, Nanoscale, 2016, 8, 2471 RSC.
- H. Moon, C. Lee, W. Lee, J. Kim and H. Chae, Adv. Mater., 2019, 31, 1804294 CrossRef.
- X. Michalet, F. F. Pinaud, L. A. Bentolila, J. M. Tsay, S. Doose, J. J. Li, G. Sundaresan, A. M. Wu, S. S. Gambhir and S. Weiss, Science, 2005, 307, 538 CrossRef CAS.
- S. E. Irvine, T. Staudt, E. Rittweger, J. Engelhardt and S. W. Hell, Angew. Chem., Int. Ed., 2008, 47, 2685 CrossRef CAS.
- M. D. Lesoine, U. Bhattacharjee, Y. J. Guo, J. Vela, J. W. Petrich and E. A. Smith, J. Phys. Chem. C, 2013, 117, 3662 CrossRef CAS.
- Y. X. Wang, G. L. Ding, J. Y. Mao, Y. Zhou and S. T. Han, Sci. Technol. Adv. Mater., 2020, 21, 278 CrossRef CAS.
- Y. Y. Dong, Y. Z. Zhao, S. Y. Zhang, Y. Dai, L. Liu, Y. J. Li and Q. Chen, J. Mater. Chem. A, 2018, 6, 21729 RSC.
- W. S. Wang, G. Y. Zhao, C. F. Kuang, L. Xu, S. C. Liu, S. Y. Sun, S. T. Ping, Y. Yang, Y. K. Xu and X. Liu, Opt. Commun., 2018, 423, 167 CrossRef CAS.
- S. Ye, W. Yan, M. J. Zhao, X. Peng, J. Song and J. L. Qu, Adv. Mater., 2018, 30, 1800167 CrossRef.
- G. Lemenager, E. De Luca, Y. P. Sun and P. P. Pompa, Nanoscale, 2014, 6, 8617 RSC.
- N. Prabhakar and J. M. Rosenholm, Curr. Opin. Colloid Interface Sci., 2019, 39, 220 CrossRef CAS.
- E. Rittweger, K. Y. Han, S. E. Irvine, C. Eggeling and S. W. Hell, Nat. Photonics, 2009, 3, 144 CrossRef CAS.
- Y. K. Tzeng, O. Faklaris, B. M. Chang, Y. Kuo, J. H. Hsu and H. C. Chang, Angew. Chem., Int. Ed., 2011, 50, 2262 CrossRef CAS.
- F. Yuan, Y. K. Wang, G. Sharma, Y. Dong, X. Zheng, P. Li, A. Johnston, G. Bappi, J. Z. Fan, H. Kung, B. Chen, M. I. Saidaminov, K. Singh, O. Voznyy, O. M. Bakr, Z. H. Lu and E. H. Sargent, Nat. Photonics, 2020, 14, 171 CrossRef CAS.
- H. Choi, S. J. Ko, Y. Choi, P. Joo, T. Kim, B. R. Lee, J. W. Jung, H. J. Choi, M. Cha, J. R. Jeong, I. W. Hwang, M. H. Song, B. S. Kim and J. Y. Kim, Nat. Photonics, 2013, 7, 732 CrossRef CAS.
- D. Y. Jin, P. Xi, B. M. Wang, L. Zhang, J. Enderlein and A. M. van Oijen, Nat. Methods, 2018, 15, 415 CrossRef CAS.
- R. T. Wu, Q. Q. Zhan, H. C. Liu, X. Y. Wen, B. J. Wang and S. L. He, Opt. Express, 2015, 23, 32401 CrossRef CAS.
- F. Ding, Y. B. Zhan, X. J. Lu and Y. Sun, Chem. Sci., 2018, 9, 4370 RSC.
- L. Cheng, C. Wang and Z. Liu, Nanoscale, 2013, 5, 23 RSC.
- I. Tavernaro, C. Cavelius, H. Peuschel and A. Kraegeloh, Beilstein J. Nanotechnol., 2017, 8, 1283 CrossRef CAS.
- N. T. Urban, M. R. Foreman, S. W. Hell and Y. Sivan, ACS Photonics, 2018, 5, 2574 CrossRef CAS.
- E. Cortes, P. A. Huidobro, H. G. Sinclair, S. Guldbrand, W. J. Peveler, T. Davies, S. Parrinello, F. Gorlitz, C. Dunsby, M. A. A. Neil, Y. Sivan, I. P. Parkin, P. M. W. French and S. A. Maier, ACS Nano, 2016, 10, 10454 CrossRef CAS.
- G. Matela, P. Gao, G. Guigas, A. F. Eckert, K. Nienhaus and G. U. Nienhaus, Chem. Commun., 2017, 53, 979 RSC.
- K. I. Willig, A. C. Stiel, T. Brakemann, S. Jakobs and S. W. Hell, Nano Lett., 2011, 11, 3970 CrossRef CAS.
- B. Hein, K. I. Willig, C. A. Wurm, V. Westphal, S. Jakobs and S. W. Hell, Biophys. J., 2010, 98, 158 CrossRef CAS.
- B. Hein, K. I. Willig and S. W. Hell, Proc. Natl. Acad. Sci. U. S. A., 2008, 105, 14271 CrossRef CAS.
- M. Kamper, H. S. Ta, N. A. Jensen, S. W. Hell and S. Jakobs, Nat. Commun., 2018, 9, 4762 CrossRef.
- C. M. Niemeyer, Angew. Chem., Int. Ed., 2010, 49, 1200 CrossRef CAS.
- W. I. Tatkiewicz, J. Seras-Franzoso, E. García-Fruitós, E. Vazquez, N. Ventosa, K. Peebo, I. Ratera, A. Villaverde and J. Veciana, ACS Nano, 2013, 7, 4774 CrossRef CAS.
- L. Shang, P. Gao, H. Wang, R. Popescu, D. Gerthsend and G. U. Nienhaus, Chem. Sci., 2017, 8, 2396 RSC.
- J. B. Xiao, Y. L. Bai, Y. F. Wang, J. W. Chen and X. L. Wei, Spectrochim. Acta, Part A, 2010, 76, 93 CrossRef.
- K. M. Tsoi, Q. Dai, B. A. Alman and W. C. W. Chan, Acc. Chem. Res., 2013, 46, 662 CrossRef CAS.
- D. S. Lidke, P. Nagy and D. J. Arndt-Jovin, Curr. Protoc. Cell Biol., 2007, 25, 25.1.1, DOI:10.1002/0471143030.cb2501s36.
- M. Lunz, A. L. Bradley, W. Y. Chen, V. A. Gerard, S. J. Byrne, Y. K. Gun'ko, V. Lesnyak and N. Gaponik, Phys. Rev. B: Condens. Matter Mater. Phys., 2010, 81, 205316 CrossRef.
- M. V. Sednev, V. N. Belov and S. W. Hell, Methods Appl. Fluoresc., 2015, 3, 042004 CrossRef.
- L. Wang, M. Tran, E. D’Este, J. Roberti, B. Koch, L. Xue and K. Johnsson, Nat. Chem., 2020, 12, 165 CrossRef CAS.
- L. Wang, M. S. Frei, A. Salim and K. Johnsson, J. Am. Chem. Soc., 2019, 141, 2770 CrossRef CAS.
- G. Lukinavicius, G. Y. Mitronova, S. Schnorrenberg, A. N. Butkevich, H. Barthel, V. N. Belov and S. W. Hell, Chem. Sci., 2018, 9, 3324 RSC.
- C. A. Wurm, K. Kolmakov, F. Göttfert, H. Ta, M. Bossi, H. Schill, S. Berning, S. Jakobs, G. Donnert, V. N. Belov and S. W. Hell, Optical Nanoscopy, 2012, 1, 7 CrossRef.
- K. Kolmakov, C. A. Wurm, D. N. H. Meineke, F. Gottfert, V. P. Boyarskiy, V. N. Belov and S. W. Hell, Chem. – Eur. J., 2014, 20, 146 CrossRef CAS.
- C. G. Wang, A. Fukazawa, M. Taki, Y. Sato, T. Higashiyama and S. Yamaguchi, Angew. Chem., Int. Ed., 2015, 54, 15213 CrossRef CAS.
- T. Stephan, A. Roesch, D. Riedel and S. Jakobs, Sci. Rep., 2019, 9, 12419 CrossRef.
- H. Rampelt, R. M. Zerbes, M. van der Laan and N. Pfanner, Biochim. Biophys. Acta, Mol. Cell Res., 2017, 1864, 737 CrossRef CAS.
- M. Chvanov, J. Phys. Chem. B, 2006, 110, 22903 CrossRef.
- B. Westermann, Nat. Rev. Mol. Cell Biol., 2010, 11, 872 CrossRef CAS.
- O. Zhao, C. Huang and F. Liu, Chem. Soc. Rev., 2011, 40, 2508 RSC.
- D. L. Ma, H. Z. He, K. H. Leung, D. S. H. Chan and C. H. Leung, Angew. Chem., Int. Ed., 2013, 52, 7666 CrossRef CAS.
- C. Forster and K. Heinze, Chem. Soc. Rev., 2020, 49, 1057 RSC.
- M. L. Saha, X. Yan and P. J. Stang, Acc. Chem. Res., 2016, 49, 2527 CrossRef CAS.
- A. Byrne, C. S. Burke and T. E. Keyes, Chem. Sci., 2016, 7, 6551 RSC.
- T. Tezgerevska, K. G. Alley and C. Boskovic, Coord. Chem. Rev., 2014, 268, 23 CrossRef CAS.
- M. Pan, W. M. Liao, S. Y. Yin, S. S. Sun and C. Y. Su, Chem. Rev., 2018, 118, 8889 CrossRef CAS.
- Q. Zhao, F. Li and C. Huang, Chem. Soc. Rev., 2010, 39, 3007 RSC.
- Y. Shen, T. Shao, B. Fang, W. Du, M. Z. Zhang, J. J. Liu, T. Y. Liu, X. H. Tian, Q. Zhang, A. D. Wang, J. X. Yang, J. Y. Wu and Y. P. Tian, Chem. Commun., 2018, 54, 11288 RSC.
- N. Ji, Nat. Methods, 2017, 14, 374 CrossRef CAS.
- X. Huang, J. Fan, L. Li, H. Liu, R. Wu, Y. Wu, L. Wei, H. Mao, A. Lal, P. Xi, L. Tang, Y. Zhang, Y. Liu, S. Tan and L. Chen, Nat. Biotechnol., 2018, 36, 451 CrossRef CAS.
- S. Sreedharan, M. R. Gill, E. Garcia, H. K. Saeed, D. Robinson, A. Byrne, A. Cadby, T. E. Keyes, C. Smythe, P. Pellett, J. B. de la Serna and J. A. Thomas, J. Am. Chem. Soc., 2017, 139, 15907 CrossRef CAS.
- D. O'Connor, A. Byrne, G. B. Berselli, C. Long and T. E. Keyes, Analyst, 2019, 144, 1608 RSC.
- X. J. Zheng, W. C. Zhu, F. Ni, H. Ai and C. L. Yang, Sens. Actuators, B, 2018, 255, 3148 CrossRef CAS.
- H. K. Xu, H. H. Zhang, G. Liu, L. Kong, X. J. Zhu, X. H. Tian, Z. P. Zhang, R. L. Zhang, Z. C. Wu, Y. P. Tian and H. P. Zhou, Anal. Chem., 2019, 91, 977 CrossRef CAS.
- S. Pajk, H. Majaron, M. Novak, B. Kokot and J. Strancar, Eur. Biophys. J. Biophys. Lett., 2019, 48, 485 CrossRef CAS.
- T. Förster and K. Kasper, Z. Phys. Chem., 1954, 1, 275 CrossRef.
- H. Saigusa and E. C. Lim, Acc. Chem. Res., 1996, 29, 171 CrossRef CAS.
- J. D. Luo, Z. L. Xie, J. W. Y. Lam, L. Cheng, H. Y. Chen, C. F. Qiu, H. S. Kwok, X. W. Zhan, Y. Q. Liu, D. B. Zhu and B. Z. Tang, Chem. Commun., 2001, 1740 RSC.
- Y. N. Hong, J. W. Y. Lam and B. Z. Tang, Chem. Commun., 2009, 4332 RSC.
- Y. N. Hong, J. W. Y. Lam and B. Z. Tang, Chem. Soc. Rev., 2011, 40, 5361 RSC.
- J. Mei, N. L. C. Leung, R. T. K. Kwok, J. W. Y. Lam and B. Z. Tang, Chem. Rev., 2015, 115, 11718 CrossRef CAS.
- M. M. Islam, Z. Hu, Q. S. Wang, C. Redshaw and X. Feng, Mater. Chem. Front., 2019, 3, 762 RSC.
- J. Mei, Y. N. Hong, J. W. Y. Lam, A. J. Qin, Y. H. Tang and B. Z. Tang, Adv. Mater., 2014, 26, 5429 CrossRef CAS.
- L. L. Yan, Y. Zhang, B. Xu and W. J. Tian, Nanoscale, 2016, 8, 2471 RSC.
- Z. K. He, C. Q. Ke and B. Z. Tang, ACS Omega, 2018, 3, 3267 CrossRef CAS.
- R. Hu, N. L. C. Leung and B. Z. Tang, Chem. Soc. Rev., 2014, 43, 4494 RSC.
- Y. F. Wang, Y. X. Zhang, J. J. Wang and X. J. Liang, Adv. Drug Delivery Rev., 2019, 143, 161 CrossRef CAS.
- D. F. Dang, H. K. Zhang, Y. Z. Xu, R. H. Xu, Z. Wang, R. T. K. Kwok, J. W. Y. Lam, L. Zhang, L. J. Meng and B. Z. Tang, ACS Nano, 2019, 13, 11863 CrossRef CAS.
- Y. X. Wang, W. Zhao, K. G. Wang, J. T. Bai, R. Juris, C. Barattini, Q. Wang and G. R. Wang, J. Nanosci. Nanotechnol., 2020, 20, 2308 CrossRef CAS.
Footnotes |
† Electronic supplementary information (ESI) available. See DOI: 10.1039/d0cs00676a |
‡ Y. Xu and R. Xu contributed equally to this work. |
|
This journal is © The Royal Society of Chemistry 2021 |
Click here to see how this site uses Cookies. View our privacy policy here.