DOI:
10.1039/D1GC02247D
(Paper)
Green Chem., 2021,
23, 9906-9915
A class of surfactants via PEG modification of the oleate moiety of lactonic sophorolipids: synthesis, characterisation and application†
Received
25th June 2021
, Accepted 13th September 2021
First published on 20th September 2021
Abstract
There is ever increasing demand to develop surfactants based on sophorolipids because they are produced by non-pathogenic organisms, biodegradable and less toxic to humans and the environment. Herein, commercially available lactonic sophorolipid was modified via epoxidation of the fatty acid units C
C and subsequent ring-opening of the oxirane with poly(ethylene glycol) of vary chain lengths to deliver a novel range of non-ionic sophorolipid-based surfactants. The methods employed for ring-opening reaction lead to a final surfactant synthesis involving heterogeneous catalysis (metal-exchanged montmorillonite), use of a benign solvent (ethyl acetate) and short reaction time (60 minutes). The resulting surfactants were structurally characterised and a prediction of their potential applications achieved using the hydrophilic-lipophilic balance (HLB) concept, foam capacity and stability of the surfactants at 0.25% surfactant solution. This new family of bio-derivable non-ionic surfactants will be useful as wetting and solubilising agents, oil-in-water emulsifiers and detergents.
Introduction
Sophorolipids (SLs) are a class of non-ionic glycolipid bio-surfactants first reported in 1961.1 They are synthesised by non-pathogenic yeasts such as Candida gropengiesseri,2Candida apicola,3Rhodotorula bogoriensis and Rhodotorula babjavae YS34 but the most widely used organism for the synthesis of SLs has been Starmerella bombicola.5 SLs consist of two molecules of glucose linked via a β-1,2 glycosidic bond (sophorose), this sophorose is in turn connected by another gylcosidic bond to a hydroxyl fatty acid. The sophorose acts as the hydrophilic head while the long chain hydroxyl fatty acid acts as the hydrophobic tail.
The production of SLs first requires biosynthesis of the hydroxy fatty acid from a fatty acid via enzymatic action of cytochrome P450 monooxygenase. This hydroxyl fatty acid is subsequently coupled to glucose by glycosyltransferase to yield a glucolipid. Thereafter, a second glucose is attached, via a β-1,2 glycosidic bond, to the glucolipid to deliver a SL having a free carboxylic acid end group 1 (Fig. 1). A further intermolecuclar esterification reaction yields a lactonic sophorolipid (LSL) 2 (Fig. 1).5 The commonly attached fatty acids on the sophorose are those in the C16–C18 range and include palmitic, stearic, oleic, linoleic and alpha-linolenic acids. Oleic acid is the most widely used of these acids because it is readily available and remains liquid at room temperature.6–11 Fatty acids containing chain length below C16 are directly metabolised through a β-oxidation mechanism while those of length above C18 are shortened and can only be introduced to the sophorose when appropriate chain length is attained. The sophorose can contain acetyl groups either at the 6′ or 6′′ hydroxyl positions (mono-acetylated) or at both positions (di-acetylated).12
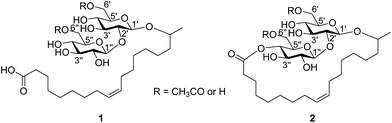 |
| Fig. 1 Free acid (1) and lactone (2) forms of sophorolipids. | |
SL surfactants have attracted commercial and academic interests for their applications in detergency, surface enhancement of electro spun fibre, bioremediation, enhanced oil recovery, medicine, home and personal care products and antimicrobial agents either as a single entity or in combination with rhamnolipids.13–18 They are readily biodegradable, less toxic and have low cytotoxicity compared to other conventional non-ionic surfactants such as Triton-X100, pluronic L31 and polyoxyethylene lauryl ether.19,20
Production yields for SLs can be up to 400 g L−1 depending on the strain of yeast used.21,22 Despite this relatively high efficiency, SLs’ large-scale production and application have yet to become significant owing to higher costs as compared to more common surfactants. This challenge is being tackled by exploring waste streams of agriculture, food and oil refining industries as low-cost renewable substrates for SL production.6,7,10,23
Modification of SLs is essential to improve properties and enhance the number of potential applications.24 A challenge with the LSL is poor water solubility as a result of the hydrophobic nature of the ester groups and because the sophorose groups are hindered in their association with water.25
LSL gives reduced surface tension, lower CMC values, and its poor water solubility makes it readily partition from the reaction mixture compared to the free SL.21 Alkaline or acid hydrolysis of LSL 2 cleaves the ester groups to produce hydroxyls and a fatty acid 3 tethered at the glycosidic linkage, this producing improved water solubility but as an anionic surfactant.26 Ozonolysis of the alkene of the fatty acid reduces the length of the carbon chain and can give surfactants with improved properties.27 A review by Delbeke et al. also highlighted modifications reported on the sophorose and fatty acid moieties of SLs.24 Some of these modifications, especially at the double bond of the fatty acid, include both ring-opening metathesis and cross-metathesis polymerisations 4, hydrogenation to 5, 6 and oxidation of the fatty acid group to form short-chained SLs 7 and 8, as shown in Scheme 1.1,3,28,30 Recently, a review by Baccile et al. presented the self-assembly properties and phase behaviour of sophorolipids along with many other bio-surfactants.31 To the best of our knowledge there has been no studies presented on modification of the fatty acid double bond in LSL to form an epoxide, through which further modifications could be made.
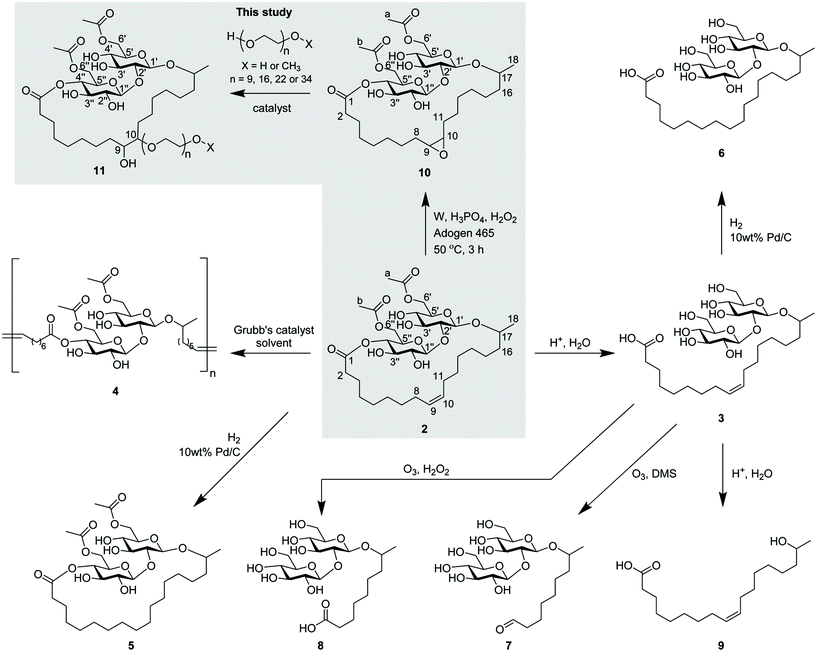 |
| Scheme 1 Modifications on the double bond of sophorolipid's fatty acid24,26,28–30 and synthesis of lactonic sophorolipid epoxide (ELSL) from lactonic sophorolipids (LSL) and subsequent modification to PEGylated surfactants (this study). | |
Hydrolyzing LSLs forms either SLs 3 with increased water solubility but that are anionic (cleaving the ester bond), resulting in challenges with hard water intolerance, or yields hydroxy fatty acid 9 (cleaving the glycosidic bond) in which the sophorose moiety of the LSLs has been lost depending on the pH of the reaction medium. We propose instead to tether poly(ethylene glycol) (PEG) chains via the C
C double bond site on the fatty acid, thus maintaining the LSL non-ionic character and retaining the lactonic structure. With bio-based PEG accessible industrially,32,33 and with many technologies already deployed for the production of bio-ethylene,34 then PEGylation affording a wholly bio-based non-ionic LSLs is a promising prospect. PEG is not toxic,35 although manufactured from toxic ethylene oxide, and has excellent properties such as high solubility in water and many organic solvents. Additionally, the possibility for variation in PEG chain length allows for a spectrum of properties and consequently broad applications in surfactants based on PEGylated LSLs.
Herein, a new range of surfactants from SLs via a heterogeneously catalysed ring opening reaction of SLs epoxides are presented. PEGs of varying chain lengths are demonstrated and reported, highlighting the versatility and tenability of this approach to non-ionic bio-based surfactants. Each surfactant is fully characterized and suitable applications suggested on the basis of hydrophilic-lipophilic balance (HLB) values.
Experimental section
List of chemicals
Poly(ethylene glycol) 400 (Sigma-Aldrich), poly(ethylene glycol) methyl ether 400 (Alfa-Aesar), poly(ethylene glycol) methyl ether 750 (Alfa-Aesar), poly(ethylene glycol) average Mn 950–1050 (Aldrich), poly(ethylene glycol) 1500 (Sigma-Aldrich), montmorillonite (Fulmont), ethyl acetate (Fischer Scientific), magnesium sulfate (Fischer Scientific), sodium bicarbonate (Fischer Scientific), silica-supported boron trifluoride (Sigma-Aldrich), phosphotungstic acid hydrate (PTA) (Aldrich), tungsten powder (Aldrich), >30% hydrogen peroxide solution in water (Sigma-Aldrich), Adogen 464 (Sigma-Aldrich), orthophosphoric acid (Fischer Scientific), lactonic sophorolipid (Ecover), iron(III) chloride (Sigma-Aldrich).
Preparation of Fe-montmorillonite catalyst
Fe-Montmorillonite clay catalyst was prepared following a previously reported procedure.36 Briefly, 1.62 g (10 mmol) iron(III) chloride was dissolved in 180 mL distilled water. To this solution, 7 g neutral montmorillonite (Fulmont) was added and the suspension heated at 60 °C for 22 hours. The resulting suspension was allowed to cool and settle and the solution decanted to leave the exchanged clay. The catalyst was washed several times by re-suspending in distilled water, centrifuging, and the solution discarded. Finally, the collected solid was dried in a vacuum oven at 90 °C for 5 hours and ground into a fine powder.
General procedure for epoxidation of lactonic sophorolipid
Epoxidation of LSL was carried out as follows: 0.75 g (4.10 mmol) tungsten powder (10 wt% with respect to sophorolipid), 3.5 mL hydrogen peroxide solution (>30% w/w water) and 3.5 mL water were added into a 250 mL round bottom flask and heated at 50 °C with agitation for 30 minutes. 0.62 g (6.36 mmol) orthophosphoric acid (∼9 wt% with respect to LSL) pre-diluted in 3.5 mL water was added dropwise over 15 minutes while stirring continuously. 7.38 g (10.72 mmol) LSL pre-dissolved in 30 mL ethyl acetate, 20 mL hydrogen peroxide solution and 0.43 g Adogen 464 (∼5 wt% with respect to LSL) were added to the reaction mixture and heated to 50 °C for 90 minutes. The reaction mixture was cooled and extracted with 250 mL ethyl acetate, the organic layer collected, dried over anhydrous magnesium sulphate, filtered and the solvent removed in vacuo to yield a fluffy hydroscopic product (7.49 g total product recovered, 99.2% yield). The product 10 was ground into powder and analysed by IR spectroscopy, ESI-MS, CHN elemental analysis and 1H and proton-decoupled 13C NMR spectroscopy. 1H NMR (700 MHz, CDCl3, ∂ ppm): 0.9 (∼3H, t, –CH(CH3)–), 1.40 (24H, overlapped, –(CH2)6CH(O)CH(CH2)6–), 2.08 (6H, s, (–CH2COOCH3)2), 2.38 (2H, t, –CH2COO–), 2.93 (∼2H, m, –HC(O)CH–), 3.50 (4H, m, overlapped, H4′, H2′, H2′′, H5′′), 3.72 (4H, m, overlapped, –CH(CH3)–, H3′, H3′′, H5′), 4.17 (1H, m br, H6′), 4.40 (3H, m br, overlapped, H6′′, H6′′, H6′), 4.59 (2H, d, H1′, H1′′), 4.98 (1H, H4′′); 13C NMR (175 MHz, CDCl3, ∂ ppm): 14.11, 20.81, 20.93, 22.26, 22.61, 22.67, 24.46, 25.59, 26.33, 27.20, 27.34, 27.79, 27.90, 28.51, 29.07, 29.12, 29.31, 29.50, 29.92, 30.09, 31.69, 31.87, 34.01, 37.54, 57.67, 57.91, 61.64, 62.28, 63.72, 69.80, 70.46, 72.37, 73.33, 74.01, 79.30, 102.29, 170.55, 171.48, 173.11; ESI-MS accurate mass, 727.3499 (MNa+, 727.3511 calc. for C34H56NaO15); CHN: %C (56.040 found, 57.940 calc.), %H (7.826 found, 8.010 calc.), %N (0.105 found); IR (ATR, ν, cm−1), 3386 (O–H str.), 2929 (a. CH2 str.), 2858 (s. CH2 str.), 1740 (C
O str.), 1451 (a. CH3 bend), 1368 (s. CH2 bend), 1236 (C–O–str.), 825 (epoxy sym. def.).
General procedure for ring opening of epoxidised lactonic sophorolipid with PEG or MePEG
Surfactants based on epoxidised lactonic sophorolipid (ELSL) were prepared as follows: 0.765 mmol PEG (hydroxyl on both chain ends) or MePEG (methyl ether on one chain end, hydroxyl on the other) was heated at 80 °C in a 50 mL round bottom flask followed by addition of 5 wt% (wrt PEG or MePEG) silica-BF3 or Fe-mont catalyst and allowed to mix thoroughly for 2 minutes. 0.766 mmol ELSL was dissolved in 5 mL ethyl acetate and added dropwise over 10 minutes, the temperature then raised to 100 °C. The ethyl acetate (used for drop-wise delivery of the ELSL) was allowed to evaporate over several minutes then the reaction was stoppered while stirring rigorously. Progress of reaction was monitored with 1H NMR spectroscopy with portions regularly taken for analysis. After 50 minutes the reaction mixture was cooled and transferred to a 250 mL beaker, diluted with 50 mL ethyl acetate and filtered to recover the catalyst. The filtrate was extracted with 25 mL distilled water in a separating funnel followed by addition of brine. The organic phase was collected and dried over anhydrous magnesium sulfate and crude product recovered following in vacuo removal of the solvent. In order to remove traces of residual Adogen 464 (carried over from the epoxidation reaction) the product was purified by re-dissolution in ethyl acetate and passed through a narrow column packed with Amberlyst 15 ion exchange resin, followed by removal of the solvent in vacuo. Product identification was by IR spectroscopy, ESI mass spectrometry, CHN elemental analysis and 1H and 13C NMR spectroscopy, analysis and mass recovered summarised below for each surfactant.
Epoxidised lactonic sophorolipid ring opened with PEG 400 (PLSL400)
0.49 g total product recovered. 1H NMR (700 MHz, CDCl3, ∂ ppm): 0.88 (0.87H, t, –CH(CH3)–), 1.42 (31H, overlapped, –(CH2)6HC(OH)CH((OCH2CH2)9OH)(CH2)6–), 2.07 (6H, s, (–CH2COOCH3)2), 2.36 (2H, t, –CH2COO–), 3.22 (∼1H, m, –HC(OH)CH((OCH2CH2)9OH)(CH2)6–), 3.56 (72H, m, overlapped, –HC(OH)CH((OCH2CH2)9OH)(CH2)6–, H4′, H2′, H2′′, H5′′, –CH(CH3)–, H3′, H3′′, H5′), 4.14 (1H, m, H6′), 4.27 (1H, m, H6′′), 4.40 (2H, m, overlapped, H6′′, H6′), 4.57 (2H, d, H1′, H1′′), 4.98 (1H, H4′′); 13C NMR (175 MHz, CDCl3, ∂ ppm): 14.10, 20.82, 20.89, 22.58, 22.70, 24.47, 26.22, 28.98, 29.03, 29.23, 31.61, 31.82, 61.55, 63.77, 70.02–70.47, 72,77, 73.88, 79.12, 83.75, 170.55, 171.29, 173.11; CHN: %C (53.249 found, 55.520 calc.), %H (8.610 found, 8.220 calc.), %N (ND); IR (ATR, ν, cm−1), 3418 (O–H str.), 2926 (s. CH3 str.), 2864 (s. CH2 str.), 1741, 1647 (C
O str.), 1456 (a. CH3 bend), 1367 (s. CH2 bend), 1242 (C–O–str.), 1095 (a. C–O–C str.).
Epoxidised lactonic sophorolipid ring opened with MePEG 400 (MPLSL400)
0.79 g total product recovered. 1H NMR (700 MHz, CDCl3, ∂ ppm): 0.89 (1.22H, t, –CH(CH3)–), 1.30 (31H, overlapped, (CH2)6HC(OH)CH((OCH2CH2)9OCH3)(CH2)6–), 2.07 (6H, s, (–CH2COOCH3)2), 2.37 (2H, t, –CH2COO–), 2.92 (2H, m, –HC(O)CH–), 3.22 (∼1H, m, –HC(OH)CH((OCH2CH2)9OCH3)–), 3.38 (3H, s, –HC(OH)CH–((OCH2CH2)9OCH3)–), 3.68 (44H, m, overlapped, –HC(OH)CH–((OCH2CH2)9OCH3)(CH2)6–, H4′, H2′, H2′′, H5′′, –CH(CH3)–, H3′, H3′′, H5′), 4.12 (1H, m, H6′), 4.37 (3H, m, overlapped, H6′′, H6′′, H6′), 4.59 (2H, d, H1′, H1′′), 4.99 (1H, H4′′); 13C NMR (175 MHz, CDCl3, ∂ ppm): 14.10, 20.80, 20.90, 21.32, 22.29, 22.57, 22.65, 24.47, 25.29, 25.62, 26.24, 27.16, 27.35, 27.57, 27.82, 27.90, 28.50, 28.98, 29.24, 29.34, 29.42, 29.89, 30.08, 31.62, 34.02, 37.53, 57.62, 57.85, 59.02, 61.66, 63.67, 69.72, 70.24–70.57, 71.91, 72.57, 73.29, 74.06, 74.99, 76.18, 79.30, 170.54, 171.39, 173.02; CHN: %C (55.155 found, 55.910 calc.), %H (8.515 found, 8.244 calc.), %N (ND); IR (ATR, ν, cm−1), 3438 (O–H str.), 2926 (s. CH3 str.), 2860 (s. CH2 str.), 1741 (C
O str.), 1455 (a. CH3 bend), 1367 (s. CH2 bend), 1240 (C–O–str.), 1109 (a. C–O–C str.).
Epoxidised lactonic sophorolipid ring opened with MePEG 750 (MPLSL750)
1.04 g total product recovered. 1H NMR (700 MHz, CDCl3, ∂ ppm): 0.87 (0.74H, t, –CH(CH3)–), 1.39 (26H, overlapped, –(CH2)6HC(OH)CH((OCH2CH2)16OCH3)(CH2)6–), 2.07 (6H, s, (–CH2COOCH3)2), 2.32 (2H, t, –CH2COO–), 3.09 (∼1H, m, –HC(OH)CH((OCH2CH2)16OCH3)–), 3.37 (3H, s, –HC(OH)CH((OCH2CH2)16OCH3)–), 3.64 (74H, m, overlapped, –HC(OH)CH((OCH2CH2)16OCH3)(CH2)6–, H4′, H2′, H2′′, H5′′, –CH(CH3)–, H3′, H3′′, H5′), 4.14 (1H, m, H6′), 4.36 (3H, m br, overlapped, H6′′, H6′′, H6′), 4.54 (2H, d, H1′, H1′′), 4.98 (1H, H4′′); 13C NMR (175 MHz, CDCl3, ∂ ppm): 14.09, 20.89, 22.58, 22.63, 26.21, 28.97, 29.39, 31.81, 50.47, 52.33, 59.01, 61.65, 63.60, 70.26–70.56, 71.90, 72.54, 74.07, 76.04, 171.04, 171.63, 173.57; CHN: %C (54.852 found, 55.833 calc.), %H (8.581 found, 8.611 calc.), %N (ND); IR (ATR, ν, cm−1), 3424 (O–H str.), 2863 (s. CH2 str.), 1741 (C
O str.), 1455 (a. CH3 bend), 1350 (s. CH2 bend), 1242 (C–O–str.), 1107 (a. C–O–C str.).
Epoxidised lactonic sophorolipid ring opened with PEG 1000 (PLSL1000)
1.55 g total product recovered. 1H NMR (700 MHz, CDCl3, ∂ ppm): 0.87 (0.91H, t, –CH(CH3)–), 1.38 (26H, overlapped, (CH2)6HC(OH)CH((OCH2CH2)22OH)(CH2)6–), 2.06 (6H, s, (–CH2COOCH3)2), 2.34 (2H, t, –CH2COO–), 3.22 (∼1H, m, –HC(OH)CH((OCH2CH2)22OH)(CH2)6–), 3.59 (150H, m, overlapped, –HC(OH)CH((OCH2CH2)22OH)(CH2)6–, H4′, H2′, H2′′, H5′′, –CH(CH3)–, H3′, H3′′, H5′), 4.11 (1H, m, H6′), 4.35 (3H, m, overlapped, H6′′, H6′, H6′′), 4.52 (2H, d, H1′, H1′′), 4.99 (1H, H4′′); 13C NMR (175 MHz, CDCl3, ∂ ppm): 14.10, 20.89, 22.56, 26.20, 28.96, 29.02, 29.31, 29.39, 61.64, 63.02, 68.63, 70.10–70.54, 72.54, 72.63, 171.20; CHN: %C (53.724 found, 53.658 calc.), %H (8.702 found, 9.175 calc.), %N (ND); IR (ATR, ν, cm−1), 3448 (O–H str.), 2888 (s. CH2 str. PEG), 1741 (C
O str.), 1465 (a. CH3 bend), 1344 (s. CH2 bend), 1280 (end group C–O–str.), 1241 (C–O–str.), 1108 (a. C–O–C str.).
Epoxidised lactonic sophorolipid ring opened with PEG 1500 (PLSL1500)
1.53 g total product recovered. 1H NMR (700 MHz, CDCl3, ∂ ppm): 0.88 (0.91H, t, –CH(CH3)–), 1.41 (29H, overlapped, –(CH2)6HC(OH)CH((OCH2CH2)34OH)(CH2)6–), 2.07 (6H, s, (–CH2COOCH3)2), 2.35 (2H, t, –CH2COO–), 3.22 (∼1H, m, –HC(OH)CH((OCH2CH2)34OH)(CH2)6–), 3.61 (168H, m, overlapped, –HC(OH)CH((OCH2CH2)34OH)(CH2)6–, H4′, H2′, H2′′, H5′′, –CH(CH3)–, H3′, H3′′, H5′), 4.14 (1H, m, H6′), 4.33 (3H, m, overlapped, H6′′, H6′, H6′′), 4.50 (2H, d, H1′, H1′′), 4.99 (1H, H4′′); 13C NMR (175 MHz, CDCl3, ∂ ppm): 14.10, 20.89, 22.30, 25.12, 26.21, 28.96, 29.02, 29.22, 29.39, 31.60, 31.81, 61.66, 63.63, 70.28–70.58, 72.54; CHN: %C (54.393 found, 55.190 calc.), %H (8.269 found, 8.760 calc.), %N (0.048 found); IR (ATR, ν, cm−1), 3408 (O–H str.), 2888 (s. CH2 str. PEG), 1741 (C
O str.), 1466 (a. CH3 bend), 1360, 1341 (s. CH2 bend), 1279 (end group C–O–str.), 1240 (C–O str.), 1108 (a. C–O–C str.).
Foam capacity and stability by stirring agitation method
50 mL aqueous surfactant solutions at concentrations of 0.25% w/v were measured into 100 mL and 50 mL graduated cylinders. Foam was generated at room temperature using Lab GEN′ Cole-Parmer GLH-02 Homogenizer while agitating the various surfactant solutions at a stirring speed marked 2 (ca. 6000 rpm) and speed marked 3 (ca. 11
000 rpm) respectively for 1 min for foam capacity, after which the volume of the foam height was read. After stirring, foam stability was assessed at 30 min intervals by measuring the reduction in foam volume in the graduated cylinder.
Formula for foam capacity and stability
Foam capacity = Foam volume/Initial sample volume × 100% |
Foam stability = Foam volume after 30 minutes/Initial foam volume × 100% |
Results and discussion
To access a new family of SLS non-ionic surfactants we planned a synthesis strategy seeking to incorporate additional hydrophilicity via the alkene of the fatty ester moiety whilst keeping the lactone intact. Epoxidation of the double bond was achieved by using a combination of hydrogen peroxide as oxidant, a phosphotungstic acid hydrate (PTA) catalyst prepared in situ and a phase transfer catalyst, Adogen 464, as shown in Scheme 1. Complete LSL conversion was achieved under 3 hours with 99.2% selectivity and >99% mass recovery of the epoxidised lactonic sophorolipid (ELSL), as a hygroscopic solid.
1H NMR spectrum of the ELSL (Fig. 2, bottom) showed full conversion of the LSL with complete disappearance of double bond C9–C10 that was previously observed at 5.33 ppm (Fig. 2, top). Appearance of epoxy protons C9–C10 at 2.93 ppm for the ELSL further confirmed effective formation of the oxirane. Some residual Adogen 464 phase transfer catalyst was detected in the ELSL and proved inseparable.
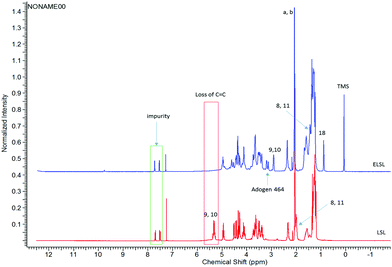 |
| Fig. 2 Proton NMR spectra of commercial lactonic sophorolipid (LSL, bottom) and epoxidised lactonic sophorolipid product (ELSL, top), showing functional changes upon a successful transformation from LSL to ELSL. AV 700 MHz Bruker spectrometer, CDCl3 solvent. | |
The methyl (–CH3) end (C18) of the fatty acid was not observed in the LSL but showed at the expected position in the epoxide. The C8 and C11 protons shifted by ∼0.5 ppm upfield following epoxidation. Fortunately, HSQC and HMBC (Fig. S1 and S2 in the ESI†) confirmed that the glycosidic bonds at C17 bonded to C1′, C4′′ bonded to C1 and between C1′′ and C2′′ were retained despite the acidic medium in which the reaction took place. The ELSL spectrum also does not show any peak representative of aldehyde or a carboxylic acid which corroborates the fact that the lactone was not hydrolytically ring-opening. The acetyl groups (a and b) on the sophorose were not hydrolysed but remained intact. Our NMR spectroscopy assignment agrees with the prior literature on the structure of LSL.30 Some impurities were observed on the proton and 13C NMR spectra for LSL despite the claim by manufacturer to have a purity of 99%, however, we were unsuccessful in determining the structure of the impurity. It is further observed in mass spectrometry below and assumed to have a molecular ion mass of 467 g mol−1.
IR spectra of ELSL, in comparison to LSL, showed the olefinic C–H stretching vibration disappearing at 3003 cm−1 and the epoxy symmetric deformation vibration appearing at 825 cm−1. The presence of signals at 1740 cm−1 for C
O stretching and 1236 cm−1 for C–O–stretching vibration corroborates NMR spectroscopy that the acetyl groups of the sophorose unit remain.
CHN analysis matches relatively well if the theoretical calculated values: %C (56.040 found, 57.940 calc.), %H (7.826 found, 8.010 calc.), %N (0.105 found). The slight disparity is linked to the unknown impurity carried through from the LSL starting material. The trace nitrogen detected is possibly from residual Adogen 464 used for epoxidation procedure and other unknown impurities. ESI-MS accurate mass was measured for LSL as 711.3559 (MNa+, C34H56NaO14) and ELSL as 727.3499 (MNa+, 727.3511 calculated for C34H56NaO15). The anticipated difference of 16 between their m/z values confirms the addition of oxygen atom across the double bond of LSL. Residual Adogen 464 along with the suspect aforementioned impurity in the commercial LSL was also detected in the ESI† spectrum of ELSL as shown in Fig. 3.
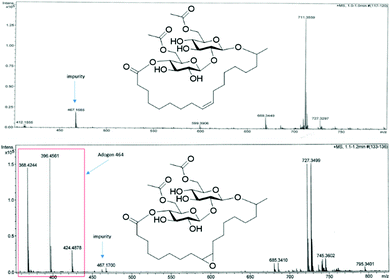 |
| Fig. 3 ESI mass spectra of LSL and epoxidised LSL (ELSL) and their inherent impurities. | |
Lewis acid catalysts were required for oxirane ring-opening reactions involving weak nucleophiles. Heterogeneous metal and non-metal Lewis acid catalysts were employed: silica-supported boron trifluoride, Si–BF3 and iron exchanged montmorillonite clay, Fe-mont. Since Si–BF3 is assumed to be toxic, Fe-mont was the preferred catalyst, although both catalysts proved effective. ELSL was dissolved in 5 mL ethyl acetate to allow for dropwise addition to heated PEGs. The ethyl acetate was then boiled off before the reaction vessel was lightly stoppered. The prepared catalyst, Fe-mont., first protonated ELSL after which the melted PEG was added to the epoxide to yield the product. A range of PEG chain lengths were investigated that included both diols (PEG 400, PEG 1000, PEG 1500) and mono methoxy-terminated (MePEG 400 and MePEG 750) (Scheme 1). Resultant surfactants were off-white to amber colour in appearance and named as presented in Table 1. Those based on PEG 400, MePEGs 400 and 750 were viscous liquid while those with PEGs 1000 and 1500 were hard-to-cut-through waxy solids.
Table 1 Synthesised surfactants from lactonic sophorolipid and their codes
Surfactant code |
Surfactant structure showing sophorolipid (S) and the attached PEG end |
Surfactant description |
ELSL |
S |
Epoxidised lactonic sophorolipid prior to reaction of (Me)PEG |
PLSL400 |
S-(OCH2CH2)9OH |
Epoxidised lactonic sophorolipid ring opened with PEG 400 |
MPLSL400 |
S-(OCH2CH2)9OCH3 |
Epoxidised lactonic sophorolipid ring opened with MePEG 400 |
MPLSL750 |
S-(OCH2CH2)16OCH3 |
Epoxidised lactonic sophorolipid ring opened with MePEG 750 |
PLSL1000 |
S-(OCH2CH2)22OH |
Epoxidised lactonic sophorolipid ring opened with PEG 1000 |
PLSL1500 |
S-(OCH2CH2)34OH |
Epoxidised lactonic sophorolipid ring opened with PEG 1500 |
As diols such as PEG are capable of reacting on both hydroxyl end groups, initial studies with methyl oleate epoxide (to mimic the hydrophobic chain of our sophorolipid) and triethylene glycol and PEG400 were carried out under similar and different reaction conditions. These model reactions were carried out, first, to allow for a more thorough structural interpretation of the PEGylated compounds and to determine any possible double addition of sophorolipids onto the PEG diols. Analysis via NMR and spectroscopy, gas chromatography and ESI-MS showed no clear evidence of disubstitution at the PEG ends (section 3.0 in the ESI†), but a small amount of double addition to the diol cannot be fully discounted from the data available.
IR spectra (Fig. 4) show successful transformation from the original lactonic sophorolipid, LSL, to the epoxide, ELSL, and then to PEGylated surfactants. The epoxy's symmetric deformation at 818 cm−1 disappeared in all the surfactants irrespective of PEG chain used. The methylene symmetric stretch vibration increased in intensity while the asymmetric stretch reduced in intensity as the number of ethylene oxide (EO) units in the PEG increased. It was observed that the C–O–C ether stretch vibration centre shifted from 1095 cm−1 to 1109 cm−1 with increasing EO repeating unit in the PEG. As there was no band observed at 1725–1700 cm−1 belonging to a carboxylic acid C
O stretch and the ester C
O stretch at 1741 cm−1 remained intact in all surfactants, it was deduced that neither the acetates on the sophorose nor the ester of the lactone were hydrolysed in the final isolated surfactants.
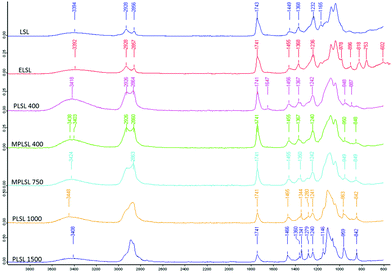 |
| Fig. 4 FT-IR spectra of LSL (top) and ELSL (second from top) compared with those of PEGylated surfactants. | |
Comparison between 1D and 2D NMR spectra of ELSL and PLSL 400 (Fig. 5-proton only and Fig. S3 and S4 in the ESI†) further confirmed that the surfactants were successfully formed. The epoxy protons peak 9, 10 disappeared while the β-epoxy protons peak 8, 11 shifted towards those of the other methylene protons in the fatty acid group. While the proton peak “10” to which the PEG is attached was conspicuous resonating at 3.22 ppm, the proton peak 9 that bears the hydroxyl end overlapped with the protons of the surfactant sophorose group. 13C and DEPT-135 spectra did not show the corresponding positions of these peaks (“9” and “10”) but HSQC (Fig. S4†) correlated the 3.22 ppm proton with the 83.75 ppm carbon. Since the position of attack cannot be controlled, attack of PEG on the oxirane ring from both faces (above or below) is possible as shown in the ESI Fig. S11,† though NMR spectroscopy could not significantly differentiate them but it is assumed it is a 50
:
50 mix of both present in the product. Additionally, there are possibilities of having the PEG chain attached to either position 9 or 10, with the corresponding –OH group in the alternative position, thus producing two regioisomers. Interestingly, COSY and HMBC (Fig. S3†) showed that positions 17 and 1′, 1′′ and 2′, and 1 and 4′′ are still bonded after synthesis. All other peaks retained their chemical shift positions after ring-opening of the oxirane with PEGs. The regio- and stereo-chemistry of the compounds could not be confirmed as they were non-crystalline thus not allowing for crystallography.
 |
| Fig. 5
1H NMR spectra of ELSL (A, top) and PLSL 400 surfactant (B, bottom) showing chemical shifts and functional changes during transformation. | |
As nitrogen, purportedly from residual Adogen 464, was detected in ELSL, CHN analysis of resulting surfactants was performed after epoxide ring-opening reaction.
Results presented in Table 2 showed that nitrogen was not detected (ND) in all the surfactants except PLSL 1500 which contained 0.048% trace nitrogen after synthesis and isolation. This would suggest that the Amberlyst 15 ion exchange resin removed most of the residual Adogen 464 left over from the oxidation step. Unfortunately, the other unknown impurity (impurities) from the commercial LSL remained within the final isolated PEGylated surfactants.
Table 2 CHN composition of ELSL and synthesised sophorolipid-based surfactants
Compound code |
Elemental analysis |
%C |
%H |
%N |
ELSL |
56.040 |
7.826 |
0.105 |
PLSL 400 |
53.249 |
8.610 |
ND |
MPLSL 400 |
55.155 |
8.515 |
ND |
MPLSL 750 |
54.852 |
8.581 |
ND |
PLSL 1000 |
53.724 |
8.702 |
ND |
PLSL 1500 |
54.393 |
8.269 |
0.048 |
A 3D model of the simplest structure of these new class of surfactants with and without solvent available surface and its optimised structure with the lowest dynamic energy are shown in the ESI section 4.0.†
Application of the surfactants based on HLB concept
The amphiphilic nature of a surfactant can be expressed in terms of hydrophilic-lipophilic balance (HLB) in addition to grouping them on the basis of their ionization in solution. The HLB concept, initiated by Griffin,37 expresses the partitioning tendency of the hydrophilic and lipophilic ends of surfactants in an oil in water (O/W) or a water in oil (W/O) emulsion. This partitioning is believed to affect the physicochemical behaviours of these surface-active agents and therefore can be applied to predict the potential application for a surfactant. Table 3 shows HLB values and their likely areas of applications. From this table it is suggested that water soluble surfactants have an HLB value higher than 13 if intended for detergency, an application commonly fulfilled by non-ionic surfactants.
Table 3 HLB ranges and applications38,39
HLB range |
Application |
3.5–6 |
Water in oil emulsifier |
7–9 |
Wetting agent |
8–15 |
Oil in water emulsifier |
13–15 |
Detergent |
15–18 |
Solubiliser |
In order to have an indication for possible application of the synthesised surfactants, the hydrophilic-lipophilic balance (HLB) concept was used. HLB for the sophorolipid-based surfactants was obtained using Griffin's equation:37
The hydrophilic groups in a typical sophorolipid-based surfactant are as shown in Fig. 6. The acetals of the glycosidic bonds could act as hydrogen bond accepting sites with water molecules as the donors, akin to ethers in PEG, thus these glycosidic bonds are included as hydrophilic groups. The hydroxyls are assumed to be the dominant contributors to hydrophilicity, while the acetates and lactone ester will contribute some hydrogen bond accepting ability. HLB was calculated for the surfactants as shown in the ESI (Section S2.0†) and respective values obtained are presented in Table S1.†
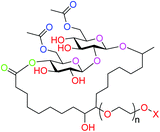 |
| Fig. 6 Hydrophilic groups identified in sophorolipid-based surfactants for HLB calculation (ethers and hydroxyls in red; acetals in purple; lactone ester in green and acetate esters in blue). | |
The calculated HLB values presented in Fig. 7 indicate that the non-PEGylated ELSL could serve as a wetting agent.
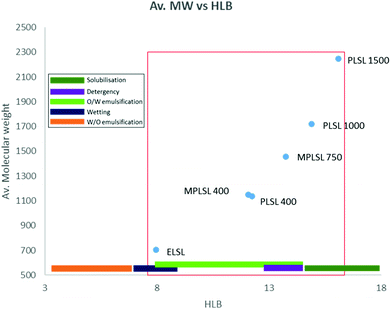 |
| Fig. 7 HLB values and average molecular weight obtained for sophorolipid-based surfactants and their potential applications. | |
Introduction of the PEG and MePEG chains increased hydrophilicity and thus extended the applications possible. PLSL 400, MPLSL 400, MPLSL 750 and PLSL 1000 are in a region suggesting application as oil-in water emulsifiers, while MPLSL 750 and PLSL 1000 could also serve as detergents and PLSL 1500 could be applied as solubilising agents. Evident, and expected from this study, is that introduction of longer PEG chains moves the materials to be overall more hydrophilic in nature and it is this that expands their applicability whilst maintaining their non-ionic amphiphilic nature.
As the surfactants’ structures are complex, the calculated HLB values are merely indicative and not true values. True values can be evaluated experimentally using improved methods from other studies.40,41
Foam capacity
Two of the synthesised surfactants, PLSL 1000 and PLSL 1500 were selected and tested for their foam capacities. It was observed that foam formation was very high due to the nature of their head group, which consist of a hydrophilic disaccharide head, alongside with ∼22 and ∼34 ether and an hydrophobic fatty acid tail of 18 carbon atoms in PLSL 1000 and 1500 respectively. The result of these polarities likely led to the outstanding increase in foam formation. Foam volume in surfactant solution generally increases with increase in surfactant concentration below the critical micelle concentration (CMC) until the neighbourhood of the CMC is reached, where foam volume gets to a maximum value or increases gradually to a peak value somehow above the CMC. The amount of foam formed in PLSL 1000 was 88% at (6000 rpm) and 104% at (11
000 rpm) as shown in Table 4, with a percentage difference of 16% when the speed was almost doubled. PLSL 1500 had a foam capacity of 104% at (6000 rpm) and 120% at (11
000 rpm) with a percentage difference of 16%. It was noticed that the foam volume increased as the ethylene oxide unit increased from 22 to 34. Sophorolipids generally are known to greatly reduce surface tension and with addition of PEG showed how the foam formation was high for this category of surfactants. The foaming capacity and stirring speed of the PLSL 1000 and 1500 surfactants were compared with foam capacity results of cetyl trimethyl ammonium bromide (CTAB) cationic surfactant, sodium dodecyl sulphate (SDS) anionic surfactant and Tween 80 (T80) non-ionic synthetic industrial surfactants as shown in Table 4.42
Table 4 Influence of stirring speed on foam volume of surfactant solutions at different concentrations
Entry |
Surfactant, Concentration (%) |
Foam volume (mL) |
6000 rpm |
8000 rpm |
9500 rpm |
1100rpm |
13 500 rpm |
Foam volumes for entries 1–3 are approximate values of what was reported.42 |
1 |
CTAB, 0.5 |
— |
13 |
27 |
— |
27 |
2 |
T80, 0.5 |
— |
11 |
24 |
— |
24 |
3 |
SDS, 0.5 |
— |
15 |
27 |
— |
27 |
4 |
PLSL 1000, 0.25 |
44 |
— |
— |
52 |
— |
5 |
PLSL 1500, 0.25 |
52 |
— |
— |
60 |
— |
It was obvious that the two modified sophorolipid surfactants demonstrated a greater foaming capacity even at a lower concentration and stirring speed than CTAB, SDS and Tween 80.
Foam stability
Foam stability at (11
000 rpm) was visually assessed by measuring the foam volume a minute immediately after foam generation, then at 30 minutes intervals for a period of 120 minutes. For the first 30 minutes, the foam volume for PLSL 1000 dropped to 30.8%. This trend, likewise, was also observed in PLSL 1500, in which the foam volume dropped to 26.7% as shown in Fig. 8. The foam volume for PLSL 1000 then slightly dropped from 30.8% to 23.1% 30 minutes after and remained like that over an hour. PLSL 1500 followed same trend with the foam volume dropping from 26.7% to 20% 30 minutes later and remained like that over an hour. The sharp fall witnessed for the first 30 minutes could be due to the type of micelles that was formed as a result of their critical packing parameter (CPP). The hydrophilic head group area was greater than the volume and length of the hydrophobic area, this in turn created an in-balance in their hydrophilic-lipophilic balance (HLB) i.e. more area covered by the hydrophilic head compared to the tail. This fall of foam in the PLSL 1000 and PLSL 1500 followed the sequence of the ethylene oxide unit, the reduction in foam was higher in PLSL 1500 than in PLSL 1000 as a result of higher PEG chain length. Causes of reduction of foam volume can be linked to drainage, coarsening and coalescence due to the micelle structure that was formed.43
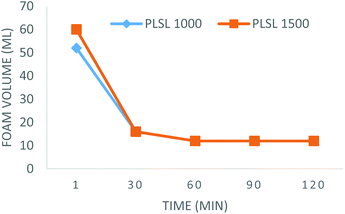 |
| Fig. 8 Foam stability of surfactants over time at solution concentration of 0.25% at 11 000 rpm. | |
Expectedly, results of foam capacity and stability agree with the HLB evaluation of the surfactants properties. The fact that PLSL1000 and PLSL1500 were soluble and formed clear solution in water confirmed that are solubilisers as predicted in Fig. 7.
Conclusion
The olefinic bond in commercially available lactonic sophorolipid was successfully epoxidised without significant side products. This modification extends the scope of derivatization of this class of industrially significant bio-surfactant. Herein, five non-ionic surfactants were synthesised via ring opening of the oxirane with PEGs of different chain length, with either hydroxyl or methoxy terminuses. Extensive characterisation of the isolated products confirmed that oxidation to the oxirane and subsequent PEGylation was successful and that neither the glycosidic nor the ester bonds (lactone and acetyls) were hydrolytically cleaved during synthesis. The catalysts used are heterogeneous and less toxic when compared with homogeneous alternatives. Foam capacity experiments showed the PEGylated lactonic sophorolipids to be better foam formers than commercial surfactants such as cetyl trimethyl ammonium bromide, sodium dodecyl sulphate and Tween 80. Based on Griffin's method for calculating HLB values, the surfactants, including the intermediate oxirane, are potential solubilizing and wetting agents, detergents, and oil-in-water emulsifiers. Of particular merit is the ability to tune the application by variation of PEG chain length, demonstrating the versatility possible with these new bio-derivable surfactants.
Author contributions
JKO designed and carried out the experiments, analysed the data and prepared the manuscripts. OEO performed the foam stability experiments. TJF, CRM, JHC and DT supervised the research and gave valuable suggestions in the experiments and manuscript editing. All authors read and gave approval to the final version of the manuscript.
Abbreviations
HLB | Hydrophilic-lipophilic balance |
LSL | Lactonic sophorolipid |
ELSL | Epoxidized lactonic sophorolipid |
CHN analyser | Carbon–hydrogen–nitrogen elemental analyser |
HMBC | Hetero nuclear multi bond correlation |
HSQC | Heteronuclear single quantum correlation |
COSY | Correlation spectroscopy |
FT-IR | Fourier transform infrared |
PTA | Phosphotungstic acid |
NMR | Nuclear magnetic resonance |
ESI-MS | Electrospray ionisation mass spectrometry |
DEPT | Distortionless enhancement through polarized transfer |
CDCl3 | Deuterated chloroform |
Conflicts of interest
Authors declare no conflicts of interest.
Acknowledgements
Authors appreciate all other funding bodies (TETfund, Nigeria and Dr Wild fund, UK) that contributed to the success of this research. Authors would like to thank Unilever PLC, UK for providing funds to support the research.
References
- P. Gorin, J. Spencer and A. Tulloch, Can. J. Chem., 1961, 39, 846–855 CrossRef CAS.
- D. Jones and R. Howe, J. Chem. Soc. C, 1968, 2801–2808 RSC.
- A. Tulloch and J. Spencer, Can. J. Chem., 1968, 46, 1523–1528 CrossRef CAS.
- S. Sen, S. N. Borah, A. Bora and S. Deka, Microb. Cell Fact., 2017, 16, 95 CrossRef PubMed.
-
D. W. G. Develter and S. J. J. Fleurackers, in Surfactants from renewable resources, ed. M. Kjellin and I. Johansson, John Wiley & Sons, United Kingdom, 2010, ch. 11, pp. 211–238 Search PubMed.
- X. Yu, X. Li, T. Jin, Y. Su, J. Li, M. Gao, L. Zheng, S. Tan and G. Chen, Biochem. Eng. J., 2021, 167, 107908 CrossRef CAS.
- J. V. Jadhav, A. P. Pratap and S. B. Kale, Process Biochem., 2019, 78, 15–24 CrossRef CAS.
- C. Ceresa, L. Fracchia, M. Williams, I. M. Banat and M. A. Díaz De Rienzo, J. Biotechnol., 2020, 309, 34–43 CrossRef CAS PubMed.
- H. Wang, G. Kaur, M. H. To, S. L. K. W. Roelants, R. D. Patria, W. Soetaert and C. S. K. Lin, J. Cleaner Prod., 2020, 246, 118995 CrossRef CAS.
- G. Kaur, H. Wang, M. H. To, S. L. K. W. Roelants, W. Soetaert and C. S. K. Lin, J. Cleaner Prod., 2019, 232, 1–11 CrossRef.
- P. Jiménez-Peñalver, A. Rodríguez, A. Daverey, X. Font and T. Gea, Rev. Environ. Sci. Bio/Technol., 2019, 18, 413–435 CrossRef.
- H.-J. Asmer, S. Lang, F. Wagner and V. Wray, J. Am. Oil Chem. Soc., 1988, 65, 1460–1466 CrossRef CAS.
- A. M. Ziemba, K. P. Lane, B. Balouch, A. R. D'Amato, F. Totsingan, R. A. Gross and R. J. Gilbert, ACS Appl. Bio Mater., 2019, 2, 3153–3158 CrossRef CAS.
- M. Elshikh, I. Moya-Ramírez, H. Moens, S. Roelants, W. Soetaert, R. Marchant and I. M. Banat, J. Appl. Microbiol., 2017, 123, 1111–1123 CrossRef CAS PubMed.
- R. A. Gross, M. Ganesh and W. Lu, Trends Biotechnol., 2010, 28, 435–443 CrossRef CAS PubMed.
- C. N. Mulligan, R. N. Yong and B. F. Gibbs, J. Hazard. Mater., 2001, 85, 111–125 CrossRef CAS.
- C. Schippers, K. Gessner, T. Müller and T. Scheper, J. Biotechnol., 2000, 83, 189–198 CrossRef CAS PubMed.
-
M. Baviere, D. Degouy and J. Lecourtier, US Pat., 5326407, 1994 Search PubMed.
- D. W. Develter and L. M. Lauryssen, Eur. J. Lipid Sci. Technol., 2010, 112, 628–638 CrossRef CAS.
- J. N. Putro, S. Ismadji, C. Gunarto, F. E. Soetaredjo and Y. H. Ju, Colloids Surf., A, 2019, 578, 123618 CrossRef CAS.
- I. N. Van Bogaert, J. Zhang and W. Soetaert, Process Biochem., 2011, 46, 821–833 CrossRef CAS.
- L. Van Renterghem, S. L. K. W. Roelants, N. Baccile, K. Uyttersprot, M. C. Taelman, B. Everaert, S. Mincke, S. Ledegen, S. Debrouwer, K. Scholtens, C. Stevens and W. Soetaert, Biotechnol. Bioeng., 2018, 115, 1195–1206 CrossRef CAS PubMed.
- X. Ma, L. Meng, H. Zhang, L. Zhou, J. Yue, H. Zhu and R. Yao, Appl. Microbiol. Biotechnol., 2020, 104, 77–100 CrossRef CAS PubMed.
- E. Delbeke, M. Movsisyan, K. Van Geem and C. Stevens, Green Chem., 2016, 18, 76–104 RSC.
- J. Penfold, M. Chen, R. K. Thomas, C. Dong, T. J. P. Smyth, A. Perfumo, R. Marchant, I. M. Banat, P. Stevenson, A. Parry, I. Tucker and I. Grillo, Langmuir, 2011, 27, 8867–8877 CrossRef CAS PubMed.
- S. J. J. Fleurackers, Eur. J. Lipid Sci. Technol., 2006, 108, 5–12 CrossRef CAS.
-
D. Develter and S. Fleurackers, European Patent, 2008, EP1953237A1 Search PubMed.
- W. Gao, R. Hagver, V. Shah, W. Xie, R. A. Gross, M. F. Ilker, C. Bell, K. A. Burke and E. B. Coughlin, Macromolecules, 2007, 40, 145–147 CrossRef CAS.
- K. S. Bisht, W. Gao and R. A. Gross, Macromolecules, 2000, 33, 6208 CrossRef CAS.
- Y. Peng, J. Decatur, M. A. R. Meier and R. A. Gross, Macromolecules, 2013, 46, 3293–3300 CrossRef CAS.
- N. Baccile, C. Seyrig, A. Poirier, S. Alonso-de Castro, S. L. K. W. Roelants and S. Abel, Green Chem., 2021, 23, 3842–3944 RSC.
-
Croda, Bio Based Ethoxylate, https://www.crodacropcare.com/en-gb/discovery-zone/bio-based-ethoxylates.
-
Croda, https://www.crodahomecare.com/en-gb/product-finder/product/4334-ECO_1_Renex_1_PEG_1_1000, https://www.crodahomecare.com/en-gb/product-finder/product/4334-ECO_1_Renex_1_PEG_1_1000, (accessed 06/08, 2021).
- A. Lundgren and T. Hjertberg, Surfactants Renewable Resour., 2010, 109–126 Search PubMed.
-
Merck, https://www.sigmaaldrich.com/NG/en/sds/mm/8.07488, https://www.sigmaaldrich.com/NG/en/sds/mm/8.07488, (accessed 08/08/2021, 2021).
- J. K. Ogunjobi, T. J. Farmer, C. R. McElroy, S. W. Breeden, D. J. Macquarrie, D. Thornthwaite and J. H. Clark, ACS Sustainable Chem. Eng., 2019, 7, 8183–8194 CrossRef CAS.
- W. C. Griffin, J. Soc. Cosmet. Chem., 1954, 5, 249–256 Search PubMed.
-
K. P. Anathapadmanabhan, Surfactants Solutions: Adsorption and Aggregation Properties, CRC Press Inc., Florida, USA, 1993 Search PubMed.
- W. C. Griffin, J. Soc. Cosmet. Chem, 1954, 5, 249–256 Search PubMed.
- J. F. Ontiveros, C. Pierlot, M. Catté, V. Molinier, J.-L. Salager and J.-M. Aubry, Colloids Surf., A, 2014, 458, 32–39 CrossRef CAS.
- J. F. Ontiveros, C. Pierlot, M. Catté, V. Molinier, J.-L. Salager and J.-M. Aubry, J. Colloid Interface Sci., 2015, 448, 222–230 CrossRef CAS PubMed.
- M. H. Amaral, J. das Neves, Â. Z. Oliveira and M. F. Bahia, J. Surfactants Deterg., 2008, 11, 275–278 CrossRef CAS.
- L. K. Shrestha, D. P. Acharya, S. C. Sharma, K. Aramaki, H. Asaoka, K. Ihara, T. Tsunehiro and H. Kunieda, J. Colloid Interface Sci., 2006, 301, 274–281 CrossRef CAS PubMed.
Footnote |
† Electronic supplementary information (ESI) available. See DOI: 10.1039/d1gc02247d |
|
This journal is © The Royal Society of Chemistry 2021 |
Click here to see how this site uses Cookies. View our privacy policy here.