DOI:
10.1039/D1MA00411E
(Paper)
Mater. Adv., 2021,
2, 5639-5644
A proton conductive hydrogen-bonded framework incorporating 18-crown-6-ether and dicarboxy-o-terphenyl moieties†
Received
6th May 2021
, Accepted 29th June 2021
First published on 30th June 2021
Abstract
To date, proton-conducting organic crystalline materials based on crown ethers have rarely been investigated. In this work, we reveal that flexible organic 18-crown-6 derivatives with one or two 4,4′-dicarboxy-o-terphenyl (CT) groups formed four kinds of crystalline frameworks: 1CT-18C6-I (P21/n), 2CT-18C6-I (P
), 2CT-18C6-II (P
) and 2CT-18C6-III (Cmc21). Single crystal X-ray diffraction analysis clearly suggested that the water molecules were involved a hydrogen-bonded network for two frameworks. In particular, a unique one-dimensional (1D) water pathway had formed in 2CT-18C6-III and the activation energy was evaluated by Arrhenius plots to be 0.14 eV, indicating that the proton jumps from H3O+ to the neighboring H2O in the hydrogen-bonded network of 2CT-18C6-III.
Introduction
Under the concept of green sustainable development, new clean fuel sources are absolutely necessary. Proton exchange membrane fuel cells (PEMFCs) are one of the most optimal technologies as an alternative to common fossil fuels.1 Proton exchange membranes (PEMs) with an ionic pathway for protons are critical for PEMFCs. Presently, organic conductive polymers, such as Nafion, sulfonated and phosphonic-based polyaromatics, and polybenzimidazoles, are employed as alternative PEM materials.2–7 They exhibit the following features required for PEMs: (a) the presence of proton-conducting functionalities,8–10 (b) poor electronic conductivities, and (c) good chemical and thermal stabilities. However, even the Nafion, achieving the outstanding proton conductivity of 10−1 S cm−1 at room temperature with 100% relative humidity (RH), still involves unavoidable defects. Thus, exploration of optimizing electrolyte materials has attracted much attention in this field. Highly crystalline materials with a periodic framework and infinite channels such as porous coordination polymers (PCPs) and metal–organic frameworks (MOFs) are, therefore, promising candidates for proton conductive materials.11
Proton conduction in PEMs is related to two mechanisms, namely, the Grotthuss and vehicle mechanisms.12–14 The vehicle mechanism indicates the diffusion of protons with the vehicles. However, the Grotthuss mechanism always occurs in a water-based degenerate system consisting of H3O+ and H2O within an infinite network of hydrogen bonds. A proton jumps from H3O+ to a neighbouring H2O as molecule rotations taking place, which contribute to a high proton conductivity.
As is well known, crown ether derivatives, such as dibenzo-18-crown-6-ether (DB18C6) with an excellent ability to bind alkali metal cations and water molecules,15,16 have drawn much attention as an ion conductive material.17 Theoretical calculation indicates that DB18C6 with H3O+ ions located in the cavities of the crown ether interact with water molecules.18,19 Therefore, crystalline frameworks composed of crown ethers are expected to show conductivity with the Grotthuss mechanism. In this connection, we were interested in hydrogen-bonded organic frameworks (HOFs),20–25 in which molecules are connected through non-covalent intermolecular interactions such as hydrogen bonds, because of their high crystallinity and high affinity toward protons. To date, some HOFs have been reported to show proton conductivity.26–32 However, proton conductive HOFs based on crown ethers are hitherto unknown.
Herein, we newly designed 18-crown-6 derivatives with one and two 4,4′-dicarboxy-o-terphenyl (CT) groups, 1CT-18C6 and 2CT-18C6, respectively, as building block molecules to develop proton conductive HOFs (Fig. 1). A working hypothesis is as follows: (1) the 18-crown-6 and carboxy groups can capture water molecules via hydrogen bonds; (2) the carboxy group also can form supramolecular synthons to organize the molecules into crystalline supramolecular frameworks;33 and (3) the rigid o-terphenyl moiety can form frameworks24,34–36 and provide space for proton conduction. To date, 2CT-18C6 has been applied as an organic ligand of MOFs,37 and proton conductive molecular crystalline materials based on 2CT-18C6 are hitherto-unknown.
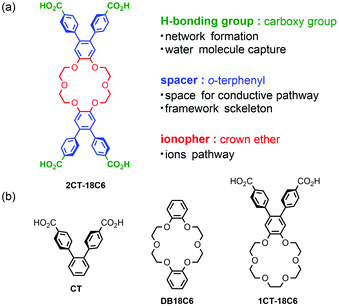 |
| Fig. 1 (a) The molecular structure of 2CT-18C6 and expected roles of the parts. (b) Related molecules 1CT-18C6, dibenzo-18-crown-6 (DB18C6), and 4,4′-dicarboxy-o-terphenyl (CT). | |
2CT-18C6 was revealed to form three kinds of crystalline frameworks (2CT-18C6-I, -II, and -III). In particular, 2CT-18C6-III has a unique one-dimensional (1D) water pathway and showed a proton conductivity of 6.75 × 10−8 S cm−1 at 85% RH. Notably, the activation energy (Ea) was evaluated by Arrhenius plots to be 0.14 eV, suggesting that the proton jumps from H3O+ to the neighbouring H2O in the hydrogen-bonding networks of 2CT-18C6-III. Thermogravimetric (TG) analysis also indicated that 2CT-18C6-III is stable up to 588 K. The present system is the first example of the tailoring of structural features of crown ethers with a 1D water pathway.
In this paper, we present the synthesis of crown ether derivatives, construction of crystalline frameworks, and evaluation of their proton conductivity. Moreover, the relationships between the structures of water pathways and proton conductivity are discussed.
Results and discussion
Synthesis and crystallization
Synthesis of 1CT-18C6 and 2CT-18C6 is shown in Scheme 1a. 4,5-Bis(methoxycarbonylphenyl)benzo-18-crown-6 (1) and 4,4′,5,5′-tetrakis(methoxycarbonylphenyl)dibenzo-18-crown-6 (2) were synthesized through a Suzuki–Miyaura cross-coupling reaction of (4-(methoxycarbonyl)phenyl)boronic acid and the corresponding brominated crown ether derivatives. Hydrolysis of 1 and 2 gave 1CT-18C6 and 2CT-18C6, respectively. Conditions to prepare the frameworks are shown in Scheme 1b. 1CT-18C6 dissolved in EtOH at room temperature for 1 day afforded colourless block crystals (1CT-18C6-I).‡ 2CT-18C6 forms three forms 2CT-18C6-I, -II, and -III.‡ Slow evaporation of a mixed solution of N,N-dimethylformamide (DMF) and water at 85 °C for several days successfully obtained two kinds of block-shaped P
crystals (2CT-18C6-I and 2CT-18C6-II) concomitantly. 2CT-18C6 dissolved in EtOH at room temperature afforded a colourless block Cmc21 crystal (2CT-18C6-III).
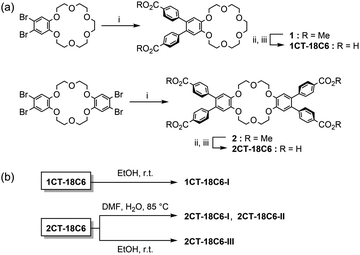 |
| Scheme 1 (a) Synthesis and (b) crystallization of 1CT-18C6 and 2CT-18C6. Reaction conditions: (i) 4-(methoxycarbonyl)phenylboronic acid, Pd(PPh3)4, K2CO3, THF, water, reflux; (ii) NaOHaq. THF, (iii) HCl. | |
Crystallography
In a 2CT-18C6-I crystal, a sodium cation is captured at the centre of the crown ether with a Na+⋯O distance ranging from 2.57 to 2.79 Å. Two DMF molecules are then coordinated to Na+ from axial directions. Because of the cation binding, the 18C6 moiety has a boat-shaped conformation with a bending angle of 76.4° (Fig. 2a), where the bending angle is defined as a dihedral angle between the mean planes of the two benzene rings of the dibenzo-18-crown-6-ether moiety. Three of the four carboxy groups form intermolecular hydrogen bonds and one binds to a DMF molecule, resulting in the formation of a ribbon like motif (Fig. 2b). It is noteworthy that the carboxy groups form no self-complementary dimer, which is often observed in hydrogen-bonded frameworks composed of highly-symmetric rigid π-conjugated molecules,22–25 but branched networks as shown in Fig. 2c. This result clearly indicates that the flexibility and lower symmetry of 2CT-18C6-I crucially affect the route to the formation of a hydrogen-bonded network. Packing of the boat shaped molecules provides small inclusion spaces, in which DMF molecules are included. In this framework, however, there is no 1D water alignment suitable for proton conduction. 2CT-18C6-II consists of three crystallographically independent molecules as well as DMF molecules. 2CT-18C6-II has almost the same structure as 2CT-18C6-I (Fig. S1, ESI†).
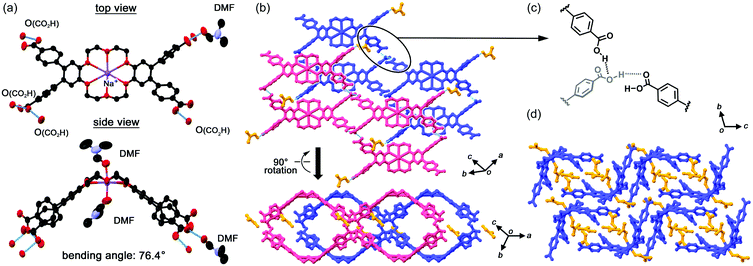 |
| Fig. 2 Crystal structure of 2CT-18C6-I. (a) Anisotropic ellipsoid plot with 50% probability. (b) One-dimensional ribbon-like motifs formed through intermolecular hydrogen bonds. (c) Branched hydrogen-bonded network formed by three carboxy groups. (d) Packing diagram, where the crown ether moiety and DMF molecules are coloured by purple and orange, respectively. | |
In the 2CT-18C6-III crystal, a Cs-symmetric 2CT-18C6 molecule has quite a shallow boat conformation with a bending angle of 25.2° (Fig. 3a), which is significantly different from 2CT-18C6-I. The observed differences in the conformation are probably caused by the existence of strongly bound cationic species in the macrocycle. The carboxy groups form hydrogen bonds with only water molecules to give the crystal, which contains no solvent but water molecules with a host/guest ratio of 1
:
3 stoichiometry (Fig. 3b). One water molecule forms hydrogen bonds with the crown ether's oxygen and the carboxy groups with a distance of 2.86 and 2.69 Å, respectively, and is isolated from neighbouring water molecules (yellow spheres). The other four molecules (red spheres), on the other hand, form hydrogen bonds with the carboxy groups (O⋯O distances: 2.72, 2.89, and 2.94 Å) to form a 1D zig-zag alignment along the c axis (Fig. 3c). The distance between the oxygen atoms of the neighbouring water molecules is 2.97 and 3.74 Å (Fig. 3d).
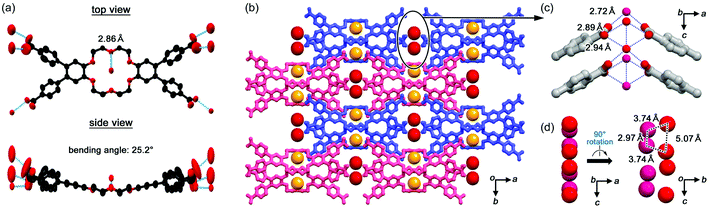 |
| Fig. 3 Crystal structure of 2CT-18C6-III. (a) Anisotropic ellipsoid plot with 50% probability. (b) Packing diagram, where two types of water molecules are coloured with red and orange. (c) Hydrogen bonds among carboxy groups and water molecules. (d) Alignment of water in the channel with intermolecular distances. | |
1CT-18C6-I also contains only water molecules with a host/guest ratio of a 1
:
3 stoichiometry. Two water molecules are hydrogen bonded by the crown ether's oxygen atoms and the others are by the carboxy groups. In total, the water molecules are aligned one dimensionally along the b axis. The distance between oxygen atoms of the neighbouring water molecules ranges from 2.77 to 3.68 Å. Although these distances are relatively shorter than that in the case of 2CT-18C6-III, the alignment crosses through the crown ether ring (Fig. 4).
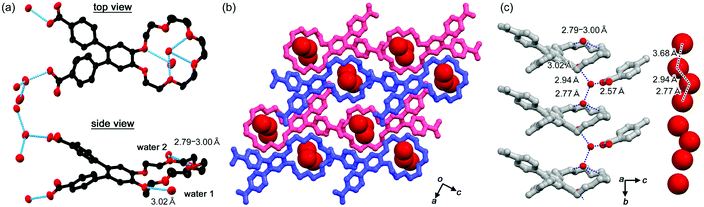 |
| Fig. 4 Crystal structure of 1CT-18C6-I. (a) Anisotropic ellipsoid plot with 50% probability. (b) Packing diagram, where water molecules are shown by the space-fill model. (c) Hydrogen bonds among the crown ether, carboxy groups, and water molecules. (d) Alignment of water in the channel with intermolecular distances. | |
Phase purity and thermal stability
The powder X-ray diffraction (PXRD) patterns of crystalline bulk samples of 1CT-18C6-I and 2CT-18C6-III are in good agreement with the ones simulated from their single-crystal data respectively (Fig. 5), indicating high phase purity and good stability. In the TG curves, 1CT-18C6-I starts losing slowly from room temperature and displays 8.9% weight loss to 373 K, which corresponds to the loss of the water molecules (cal. 8.9%). Then the framework is maintained until 588 K (Fig. 5a). Similarly, 2CT-18C6-III shows a 4.5% weight loss of water molecules from room temperature to 373 K (cal. 4.5%) and the framework also collapses after 588 K (Fig. 5b). On the other hand, variable temperature-PXRD plots show both 1CT-18C6-I and 2CT-18C6-III with an obvious change of structure with the increase of the temperature, which result from the reorganization of the hydrogen bonds connected with the loss of the water molecules (Fig. 5c and d).
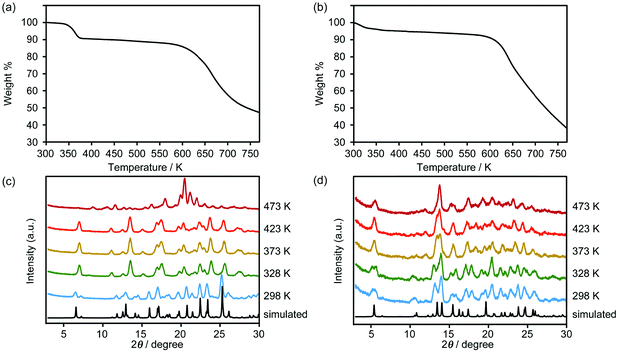 |
| Fig. 5 TG plots of as-formed crystals of (a) 1CT-18C6-I and (b) 2CT-18C6-III. Variable temperature PXRD patterns of (c) 1CT-18C6-I and (d) 2CT-18C6-III, where simulated patterns based on SXRD analysis are shown at the bottom. | |
Proton conductivity
The structures of 1CT-18C6-I and 2CT-18C6-III were constructed by a hydrophilic crown ether moiety, assembly of carboxy groups, and 1D alignment of water molecules. In addition, TG and variable temperature-PXRD analyses suggested the frameworks of 1CT-18C6-I and 2CT-18C6-III were maintained after the removal of water molecules. These results indicated that there were enough sites for water molecules supplied at a higher relative humidity. These structural features could provide an orderly route for proton transport. Therefore, 1CT-18C6-I and 2CT-18C6-III are expected to show proton conductivity. Due to the limitation of the size of the samples, a compressed pellet of the samples was used for the measurement for proton conductivity. As a reference, proton conductivity under the same conditions was measured for pelletized samples of CT and DB18C6.
Temperature-dependent Nyquist plots under 85% RH for four samples of 1CT-18C6-I, 2CT-18C6-III, CT, and DB18C6 are summarized in Fig. 6a and b, Fig. S3, and S4 (ESI†), respectively.
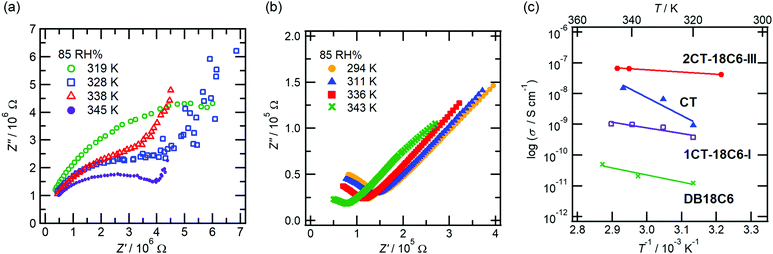 |
| Fig. 6 Proton conductivity for 1CT-18C6-I and 2CT-18C6-III, CT and DB18C6. Nyquist plots for (a) 1CT-18C6-I and (b) 2CT-18C6-III. (c) Arrhenius plot for proton conductivities under 85 RH%. | |
The Nyquist plots (Fig. 6a and b and Fig. S3, ESI†) of 1CT-18C6-I, 2CT-18C6-III, and CT are the sum of a straight line and a semicircle in the low and high frequency range, respectively. On the other hand, each Nyquist diagram of DB18C6 was composed of a single semicircle (Fig. S4, ESI†). Since the pellet sample was used to measure the proton conductivity, the Nyquist plot showed the proton conductions which are contributed from a bulk and an interface. Since bulk conduction is usually observed in the higher frequency range than the interface,11,38 the semicircles in the Nyquist diagram of 1CT-18C6-I, 2CT-18C6-III, and CT are attributed to bulk conduction. For the calculation of proton conductivity, we applied the equivalent circuit model. The model for the conductivity of DB18C6 is that a resistor and constant phase element (CPE) are arranged in parallel, while the model for that of 1CT-18C6-I, 2CT-18C6-III, and CT is that two parallel pairs of resistors and CPEs were lined up in series.
Regarding the conductivity estimated from the equivalent circuit model, an Arrhenius plot was summarized from the bulk conductivity of 1CT-18C6-I, 2CT-18C6-III, and CT, and the conductivity of DB18C6 (Fig. 6c). From the Arrhenius plot, the proton conductivities at room temperature and Ea of 1CT-18C6-I, 2CT-18C6-III, CT, and DB18C6 were estimated, and summarized in Table 1. The proton conductivity at room temperature was higher in the order of 2CT-18C6-III, 1CT-18C6-I, CT, and DB18C6, and the Ea was also lower in the same order. The proton conductivities of MFM-511 and MFM-512, which are the MOF samples with the carboxy group involved in proton transport, are 4.0 × 10−8 (87% RH) and 2.5 × 10−7 S cm−1 (77% RH) at 298 K, respectively.38 The value of proton conductivity from 2CT-18C6-III is in the similar order to MFM-511 and MFM-512.
Table 1 Proton conductivity at 300 K and activation energy (Ea) of 1CT-18C6-I, 2CT-18C6-III, CT, and DB18C6, estimated from the Arrhenius plot
|
σ @300 K/S cm−1 |
E
a/eV |
1CT-18C6-I
|
1.95 × 10−10 |
0.354 |
2CT-18C6-III
|
3.43 × 10−8 |
0.140 |
CT |
8.69 × 10−11 |
1.150 |
DB18C6 |
3.97 × 10−12 |
0.452 |
Referring to the proton transport, proton conduction can be classified as the vehicle and the Grotthuss mechanisms, which are divided by Ea = 0.4 eV. When Ea is larger and smaller than 0.4 eV, proton conductivity is estimated to be by the vehicle and Grotthuss mechanisms, respectively.
Since the Ea values of DB18C6 and CT are higher than 0.4 eV, the proton conducting mechanism of DB18C6 and CT is attributed to the vehicle one. On the other hand, 1CT-18C6-I and 2CT-18C6-III had lower Ea values than 0.4 eV, and therefore, the proton conduction of them is due to the Grotthuss mechanism. Both 1CT-18C6-I and 2CT-18C6-III had continuous hydrogen-bonded networks, resulting in the effective proton-conducting pathways and corresponding to the Grotthuss mechanism. Notably, 2CT-18C6-III has two unique linear water pathways. Water molecules in 2CT-18C6-III were not only arranged along the 1D array of the crown ether unit, but also arranged in the parts formed by the hydrogen-bonded networks surrounded by a carboxy group. Therefore, 2CT-18C6-III exhibited the highest proton conductivity of 3.43 × 10−8 S cm−1 under 85% RH at 300 K.
Conclusions
In this study, we newly applied 18-crown-6 derivatives with one or two 4,4′-dicarboxy-o-terphenyl (CT) moieties as building block molecules to develop organic crystalline materials with proton conductivity. The 18-crown-6 and carboxy groups capture water molecules via hydrogen bonds and constructed good self-adaptability to water molecule frameworks especially for 2CT-18C6-III with a unique 1D water pathway, which provides available space for proton conduction. Based on this study, it is possible to develop a novel proton conductive flexible framework with good adaptability to guest molecules. In addition, the high chemical stability and superior thermal stability indicate a promising and potential application in PEMFCs.
Conflicts of interest
There are no conflicts to declare.
Acknowledgements
This work was supported by KAKENHI (JP18H01966, JP19H04557, and JP21H01919) from JSPS and by the Dynamic Alliance for Open Innovation Bridging Human, Environment and Materials from MEXT Japan. X. C. thanks the Chinese Scholarship Council (CSC) for financial support. The authors thank Prof. Y. Sagara (currently at Tokyo Institute of Technology) and N. Tamaoki at RIES, Hokkaido University, for purification of compounds by preparative HPLC. X-Ray diffraction data, including preliminary data sets, were partly collected at BL02B1 and BL40XU in SPring-8 with the approval of JASRI (proposal No. 2019A1161, 2019B1134, and 2020A1117). The authors thank Dr. Kunihisa Sugimoto and Nobuhiro Yasuda at SPring-8 for collection of X-ray diffraction data. The authors also thank the reviewers for their accurate comments.
Notes and references
- R. Bashyam and P. Zelenay, Nature, 2006, 443, 63–66 CrossRef CAS PubMed.
- R. Devanathan, Energy Environ. Sci., 2008, 1, 101–119 RSC.
- A. Kraytsberg and Y. Ein-Eli, Energy Fuels, 2014, 28, 7303–7330 CrossRef CAS.
- R. P. Bisbey and W. R. Dichtel, ACS Cent. Sci., 2017, 3, 533–543 CrossRef CAS PubMed.
- A. I. Cooper, ACS Cent. Sci., 2017, 3, 544–553 CrossRef CAS.
- H. Zhang and P. K. Shen, Chem. Rev., 2012, 112, 2780–2832 CrossRef CAS.
- M. Yoon, K. Suh, S. Natarajan and K. Kim, Angew. Chem., Int. Ed., 2013, 52, 2688–2700 CrossRef CAS.
- S. Bureekaew, S. Horike, M. Higuchi, M. Mizuno, T. Kawamura, D. Tanaka, N. Yanai and S. Kitagawa, Nat. Mater., 2009, 8, 831–836 CrossRef CAS PubMed.
- D. Umeyama, S. Horike, M. Inukai, T. Itakura and S. Kitagawa, J. Am. Chem. Soc., 2012, 134, 12780–12785 CrossRef CAS PubMed.
- J. A. Hurd, R. Vaidhyanathan, V. Thangadurai, C. I. Ratcliffe, I. L. Moudrakovski and G. K. H. Shimizu, Nat. Chem., 2009, 1, 705–710 CrossRef CAS.
- D.-W. Lim and H. Kitagawa, Chem. Rev., 2020, 120, 8416–8467 CrossRef CAS PubMed.
- K. D. Kreuer, Chem. Mater., 1996, 8, 610–641 CrossRef CAS.
- W. Weppner, K. D. Kreuer and A. Rabenau, Angew. Chem., Int. Ed. Engl., 1982, 122, 208–209 Search PubMed.
- P. Ramaswamy, N. E. Wong and G. K. H. Shimizu, Chem. Soc. Rev., 2014, 43, 5913–5932 RSC.
- C. J. Pedersen, J. Am. Chem. Soc., 1967, 89, 2495–2496 CrossRef CAS.
- C. J. Pedersen, J. Am. Chem. Soc., 1967, 89, 7017–7036 CrossRef CAS.
- G. W. Gokel, W. M. Leevy and M. E. Weber, Chem. Rev., 2004, 104, 2723–2750 CrossRef CAS PubMed.
- M. Bühl and G. Wipff, J. Am. Chem. Soc., 2002, 124, 4473–4480 CrossRef.
- K. M. Fromm and R. D. Bergougnant, Solid State Sci., 2007, 9, 580–587 CrossRef CAS.
- Y. F. Han, Y. X. Yuan and H. B. Wang, Molecules, 2017, 22, 266 CrossRef PubMed.
- J. Luo, J. W. Wang, J. H. Zhang, S. Lai and D. C. Zhong, CrystEngComm, 2018, 20, 5884–5898 RSC.
- R. B. Lin, Y. He, P. Li, H. Wang, W. Zhou and B. Chen, Chem. Soc. Rev., 2019, 48, 1362–1389 RSC.
- I. Hisaki, C. Xin, K. Takahashi and T. Nakamura, Angew. Chem., Int. Ed., 2019, 58, 11160–11170 CrossRef CAS.
- I. Hisaki, J. Inclusion Phenom. Macrocyclic Chem., 2020, 96, 215–231 CrossRef CAS.
- B. Wang, R.-B. Lin, Z. Zhang, S. Xiang and B. Chen, J. Am. Chem. Soc., 2020, 142, 14399–14416 CrossRef CAS PubMed.
- A. Karmakar, R. Illathvalappil, B. Anothumakkool, A. Sen, P. Samanta, A. V. Desai, S. Kurungot and S. K. Ghosh, Angew. Chem., Int. Ed., 2016, 55, 10667–10671 CrossRef CAS PubMed.
- Y. Qin, T.-I. Gao, W.-P. Xie, Z. Li and G. Li, ACS Appl. Mater. Interfaces, 2019, 11, 31018–31027 CrossRef CAS PubMed.
- Z.-B. Sun, Y.-L. Li, Z.-H. Zhang, Z.-F. Li, B. Xiao and G. Li, New J. Chem., 2019, 43, 1063710644 Search PubMed.
- P. Tholen, C. A. Peeples, R. Schaper, C. Bayraktar, T. S. Erkal, M. M. Ayhan, B. Çoşut, J. Beckmann, A. O. Yazaydin, M. Wark, G. Hanna, Y. Zorlu and G. Yücesan, Nat. Commun., 2020, 11, 3180 CrossRef CAS PubMed.
- Q. Yang, Y. Wang, Y. Shang, J. Du, J. Yin, D. Liu, Z. Kang, R. Wang, D. Sun and J. Jiang, Cryst. Growth Des., 2020, 20, 3456–3465 CrossRef CAS.
- Y. Wang, J. Yin, D. Liu, C. Gao, Z. Kang, R. Wang, D. Sun and J. Jiang, J. Mater. Chem. A, 2021, 9, 2683–2688 RSC.
- B.-B. Hao, X.-X. Wang, C.-X. Zhang and Q. Wang, Cryst. Growth Des., 2021, 21, 3908–3915 CrossRef CAS.
- G. R. Desiraju, Angew. Chem., Int. Ed. Engl., 1995, 34, 2311–2327 CrossRef CAS.
- K. Kobayashi, T. Shirasaka, E. Horn and N. Furukawa, Tetrahedron Lett., 2000, 41, 89–93 CrossRef CAS.
- I. Hisaki, S. Nakagawa, N. Ikenaka, Y. Imamura, M. Katouda, M. Tashiro, H. Tsuchida, T. Ogoshi, H. Sato, N. Tohnai and M. Miyata, J. Am. Chem. Soc., 2016, 138, 6617–6628 CrossRef CAS PubMed.
- I. Hisaki, Y. Suzuki, E. Gomez, Q. Ji, N. Tohnai, T. Nakamura and A. Douhal, J. Am. Chem. Soc., 2019, 141, 2111–2121 CrossRef CAS.
- D.-W. Lim, S. A. Chyun and M. P. Suh, Angew. Chem., Int. Ed., 2014, 53, 7819–7822 CrossRef CAS PubMed.
- P. Rought, C. Marsh, S. Pili, I. P. Silverwood, V. G. Sakai, M. Li, M. S. Brown, S. P. Argent, I. Vitorica-Yrezabal, G. Whitehead, M. R. Warren, S. Yang and M. Schröder, Chem. Sci., 2019, 10, 1492–1499 RSC.
Footnotes |
† Electronic supplementary information (ESI) available: Synthesis of compounds, crystal data, crystal structure of 2CT-18C6-II and impedance spectroscopy of frameworks. CCDC 2057700, 2078832, 2078834 and 2078835. For ESI and crystallographic data in CIF or other electronic format see DOI: 10.1039/d1ma00411e |
‡ Crystal data for 1CT-18C6-I, C30H36O13; Fw = 604.59; monoclinic, P21/n (#14), Z = 4, a = 13.6633(2) Å, b = 8.2601(1) Å, c = 26.8789(6) Å, β = 90.208(2)°, V = 3033.54(9) Å3, T = 223 K, D = 1.324 g cm−3, 12 904 collected, 5424 unique (Rint = 0.031) reflections, and the final R1 and wR2 values are 0.059 [I > 2.0σ(I)] and 0.184 (all data), respectively. CCDC 2057700. Crystal data for 2CT-18C6-I, C58.5H64.5N3.5 Na O17.5; Fw = 1119.62; triclinic, P (#2), Z = 2, a = 11.3113(2) Å, b = 13.4267(2) Å, c = 21.7047(4) Å, α = 104.103(2)°, β = 95.267(1)°, γ = 111.048(2)°, V = 2924.57 (10) Å3, T = 293 K, D = 1.271 g cm−3, 31 008 collected, 11 017 unique (Rint = 0.026) reflections, and the final R1 and wR2 values are 0.061 [I > 2.0σ(I)] and 0.209 (all data), respectively. CCDC 2078832. Crystal data for 2CT-18C6-II, C57H60N3NaO17; Fw = 1082.07; triclinic, P (#2), Z = 6, a = 13.1094(8) Å, b = 25.3405(16) Å, c = 26.7457(16) Å, α = 105.281(5)°, β = 90.128(5)°, γ = 91.834(5)°, V = 8565.8(9) Å3, T = 293 K, D = 1.259 g cm−3, 89 237 collected, 32 350 unique (Rint = 0.116) reflections, and the final R1 and wR2 values are 0.081 [I > 2.0σ(I)] and 0.352 (all data), respectively. CCDC 2078834. Crystal data for 2CT-18C6-III, C48H42O17; Fw = 890.81; orthorhombic, Cmc21 (#36), Z = 4, a = 27.4687(10) Å, b = 20.3236(8) Å, c = 8.0251(6) Å, V = 4480.1(4) Å3, T = 298 K, D = 1.321 g cm−3, 29 283 collected, 4181 unique (Rint = 0.128) reflections, and the final R1 and wR2 values are 0.094 [I > 2.0σ(I)] and 0.288 (all data), respectively. CCDC 2078835. |
|
This journal is © The Royal Society of Chemistry 2021 |
Click here to see how this site uses Cookies. View our privacy policy here.