DOI:
10.1039/D0NA00936A
(Paper)
Nanoscale Adv., 2021,
3, 1106-1120
The improved photocatalytic activity of highly expanded MoS2 under visible light emitting diodes†
Received
9th November 2020
, Accepted 27th December 2020
First published on 28th December 2020
Abstract
Photocatalytic degradation is a promising method to remove organic pollutants from water. Photocatalysts based on two-dimensional (2D) transition metal dichalcogenides (TMDs) such as MoS2 nanomaterials have gained tremendous popularity. This is due to their narrow band gap and high visible light absorption. Herein, a MoS2 photocatalyst with highly expanded interlayer spaces of 1.51 nm was synthesized in the presence of Pluronic F-127 as a template by a facile one-pot hydrothermal method. This expanded MoS2 (MF-1) managed to photodegrade 98% (2.62 × 10−2 min−1) of methylene blue (MB) dye under irradiation of 1 W visible light-emitting diode (LED) white light. The dominant performance of MF-1 is attributed to the highly expanded interlayer spacing, which exposed more active edge sites. Moreover, the formation of surface defects such as surface cracks and sulfur vacancies (Sv) facilitates the adsorption capacity and in situ generation of reactive oxygen species (ROS). The dominant ROS responsible for the photodegradation of MB is superoxide radical (˙O2−). The photocatalyst shows good recyclability without deterioration even after five consecutive cycles.
1. Introduction
The growth of the human population and rapid development of industrial activities has led to environmental pollution. Water pollution is often a result of irresponsible anthropogenic activities. For instance, discharge of untreated dye effluents from the textile industry is a major contributor to water pollution.1 It is estimated that 200
000 metric tons of wastewater containing harmful organic chemical substances are discharged annually without proper treatment technology.2 Untreated dye molecules in water bodies may pose substantial threats to aquatic life and human health, as they undergo chemical and biological transformation to toxic substances. According to sustainable development goal (SDG) 2030, clean water and sanitation is a particularly important goal to ensure that drinking water is safe and affordable for people worldwide.3 Hence, efficient technologies for the removal of water pollutants are greatly desired and anticipated.
In past decades, many methods have been introduced to remove dye pollutants effectively from wastewater, including adsorption,4 photocatalytic degradation,5 catalytic oxidation6 and biological processes.7 Amongst these, photocatalytic degradation has been widely reported as an efficient and biosafe remedy.8 This is due to the versatility and sustainability of photocatalysts, which can be activated under the irradiation of natural sunlight to degrade dye molecules.9 TiO2 is a traditional photocatalyst that has been actively applied in photocatalytic degradation activity. However, due to its wide band gap of ∼3.2 eV, it can only be activated under UV light irradiation.10 In other words, TiO2 can only be activated by UV light, which represents approximately less than 5% of solar light. Therefore, many researchers have attempted to tune the band gap of TiO2 to the visible light-active region or have synthesized new types of photocatalysts.10 Recently, the usage of an energy-efficient light source such as LED white light has attracted considerable interest than that of visible light from traditional UV lamps or high-power xenon lamps.11 Although LED light is more energy-efficient, presently, there are only a few reports on the use of LED white light-active photocatalysts such as ZnO0.6S0.4,12 Ag/AgBr,13 Fe3+-doped g-C3N4 (ref. 14) and g-C3N4/Nb2O5 (ref. 15) for the photodegradation of water pollutants. They are capable of photodegrading organic pollutants within 240 min on irradiation with 3–150 W visible LED light.
MoS2 nanostructures are a transition metal dichalcogenide (TMD) material that is commonly used as a co-catalyst in the photocatalytic activity.16,17 However, MoS2 is a potential candidate in photocatalysis due to its unique physiochemical and optical properties, which can be modified by reducing the MoS2 staking layer.18 Generally, bulk MoS2 is optically inactive and also shows low photoluminescence response due to its indirect band gap of 1.29 eV.19 Meanwhile, the direct band gap of single or few layers-thick MoS2 exhibits a peak at 1.8–1.9 eV; therefore, it is a highly suitable semiconductor oxide to be activated within the visible light region.20 Moreover, with such a narrow band gap, MoS2 possesses rapid transfer of charge carriers along with wide absorption in the range of visible light; therefore, it is a promising and valuable photocatalyst candidate.20,21
Generally, MoS2 is fabricated based on the tri-atomic-thick regime of S–Mo–S layers, where the Mo atom is located between two trigonal atomic layers of S atom.22 The bond between Mo and S atom is linked by strong covalent bonds, whereas the interlayer of S–Mo–S is bonded via van der Waals forces.23 The crystalline MoS2 consists of basal and edge planes, where the former is inherently and thermodynamically favored to have higher surface exposure.24 Despite this, the photocatalytic activity of MoS2 is often limited as a result of its dependency on the availability of edge sites.25 Therefore, many studies have been devoted to improving the exposure of MoS2 edge sites through morphology control,26 development of amorphous-state MoS2 with unsaturated Mo and S sites,27 surface defects28 and expansion of the interlayer distance.29 Generally, the intrinsic structure of MoS2 has an interlayer spacing of around 0.62 nm.30 It has been reported that expansion of the interlayer spacing of MoS2 can reach 0.91 nm. This spacing enabled the expanded MoS2 to form a composite with CdS nanorods, and the photocatalytic activity was enhanced by 21-fold.31
The interaction between the MoS2 layers is governed by weak van der Waals forces. Upon introduction of foreign substances such as oxygen,32 soft templates,22 polymers33 or carbon,34 the interlayer spacing of MoS2 can be expanded. Furthermore, the intercalation of foreign substances indirectly instigates defects in the crystal structure, which can enhance the exposure of the catalyst active sites. For instance, Li et al.22 synthesized expanded MoS2 with polyethylene glycol (PEG) under a hydrothermal route. Zhou et al.32 synthesized oxygen-incorporated defect-rich MoS2 nanosheets with expanded interlayers through a solvothermal method. The expanded interlayer spacing that exposes more edge sites manages to improve the performance of MoS2 for the electrocatalytic activity of the hydrogen evolution reaction (HER). This is because the expansion of the interlayers allows the intercalation of foreign ions, improving the electrochemical performance. However, the photocatalytic activity of the highly expanded interlayer MoS2 is still very limited.
In this study, highly expanded MoS2 was successfully synthesized by a facile one-pot hydrothermal method. A Pluronic F-127 surfactant template under acidic conditions was employed to produce highly expanded interlayers of MoS2. The highly expanded MoS2 exhibited high photocatalytic performance under 1 W of LED white light irradiation and great reusability.
2. Experimental
Preparation of MoS2 photocatalyst
A suspension was prepared by mixing 4 mmol of Na2MoO4·2H2O [99.5%, Merck] and 8 mmol of C2H5NS [99.0%, Merck] in 50 mL of deionized water. Then, the mixture was stirred for 30 min. Subsequently, F-127 (0.4 g) was added to the suspension, and the pH was adjusted to 1, 3, and 5, respectively, with HCl (37%, Merck). The suspensions with pH 1, 3 and 5 are denoted as MF-1, MF-3 and MF-5, respectively. Then, the solution was further stirred for 3 h before being transferred into a Teflon-lined stainless steel autoclave. The autoclave was subjected to hydrothermal treatment at 180 °C for 24 h. After hydrothermal treatment, the precipitate was washed with acetone, ethanol and lastly distilled water several times. For comparison purposes, MoS2 was synthesized without the addition of F-127 or adjustment of the pH; this sample is denoted as M. Meanwhile, the MoS2 sample with the addition of F-127 only is denoted as MF.
Photocatalytic degradation experiment
The photocatalytic degradation activity was studied using a custom-made photoreactor with 1 W LED as the light source. The distance between the light source and dye solution is 2 cm. Then, 10 mg of MoS2 was added to 50 mL of 20 mg L−1 MB dye solution and allowed to stir in the dark for 30 min to achieve adsorption–desorption equilibrium. The photodegradation reaction was initiated for 120 min, and 3 mL of suspension was collected at 15 min intervals. The collected suspension was filtered and analyzed using a UV-vis spectrophotometer at a maximum absorption wavelength of 664 nm. The efficiency of the synthesized MoS2 in the photocatalytic degradation of MB dyes was calculated by eqn (1). |  | (1) |
where Co represents the initial concentration of dye (ppm) and C is the recorded value of the variable reaction concentration (ppm). Chemical oxygen demand (COD) tests were conducted to identify the capacity of the photocatalyst in the mineralization of MB dye.35 The COD value obtained refers to the amount of oxygen needed by the organic dyes to be oxidized into CO2 and H2O species. The chemical oxygen demand (COD) levels before and after degradation of the solution were identified using the APHA Standard Method, whereby the samples were added to COD digestion solution and digested at 150 °C for 2 h. The COD level was then measured using a HACH DR2800 spectrophotometer and the COD removal (%) was calculated based on eqn (2): |  | (2) |
where CODi and CODf are the initial and final concentrations of COD in the pollutant solution, respectively.
Characterization of the MoS2 samples
The X-ray diffraction (XRD) of the MoS2 samples was studied using a PANalytical EMPYREAN diffractometer with Cu Kα (λ = 1.54 Å), scanning 2θ in the range of 5–80° and a step size of 0.04° s−1. The d-spacings of the samples were calculated using eqn (3). | nλ = 2dhkl sin θ | (3) |
where dhkl represents the perpendicular distances between the hkl plates, n is the order of diffraction, λ is the incident ray wavelength and θ is the angle of incidence, also referred to as the angle of scattering. Meanwhile, the crystallite sizes were calculated using the Debye–Scherrer formula (eqn (4)). |  | (4) |
where D is the crystallite size, λ is the incident ray wavelength, β is the full width at half maximum (FWHM) and θ refers to the Bragg diffraction angle. The surface morphologies of the MoS2 samples were observed and captured using a field-emission scanning electron microscope (FESEM, FEI QuantaFEG650 S) with 20 kV radiation. The synthesized sample was mounted on FESEM stubs and coated with a layer of gold. High-resolution images were captured using a high resolution transmission electron microscope (HRTEM, FEI Tecnai G2 F20), operated at an acceleration voltage of 200 kV with 0.2 nm resolution. Raman and photoluminescence (PL) tests were performed using a Renishaw inVia Raman Microscope (Gloucestershire, UK) with 514 nm and 325 nm Ar-ion lasers, respectively. The optical properties of the MoS2 samples were recorded using an ultraviolet-visible spectrophotometer (UV-Vis, Perkin Elmer Lambda 35 UV-Vis spectrophotometer) from 200–800 nm. The band gaps of the samples were obtained using Tauc's equation (eqn (5)):where α is the absorption coefficient, hv is the photon energy, Eg represents the optical band gap and A is the proportionality constant, which is n = 2 for direct transition mode. The chemical and elemental analysis of the samples was performed using a JEOL JPS-9030 X-ray photoelectron spectrometer (XPS, Tokyo, Japan) equipped with an X-ray source of Mg Kα (1253.6 eV). The binding energy of the XPS element peaks obtained was calibrated with the C 1s standard position at 284.2 eV. The procedure for XPS analysis was conducted based on three requirements: (i) the spin–orbit splitting energies of Mo 3d and S2p are fixed to 3.13 ± 0.2 eV and 1.18 ± 0.2 eV, respectively, (ii) the constraint of the FWHM for both peaks in a doublet is fixed to be constant, and (iii) the peak area ratio of the doublet peak is assigned based on the degeneracy of the spin state. The stoichiometric ratios (S/Mo) for the samples were obtained based on the integrated peak area of the ratio of the Mo 3d and S 2p states with the use of relative sensitivity factors (RSF) of 9.5 and 1.67, respectively.
Photoelectrochemical study of the MoS2 samples
Photoelectrochemical measurements of the samples were performed on a Metrohm Autolab instrument (PGSTAT302N). The electrochemical workstation involved a three-electrode system with the sample coated on fluorine-doped tin oxide glass (1 × 1 cm) as the working electrode, Ag/AgCl as the reference electrode and Pt as the counter electrode. 0.5 M of aqueous Na2SO4 at pH 6.5 was used as an electrolyte. The photocurrent test was conducted using a 150 W Xe lamp with an applied bias of 0.2 V. The electrochemical impedance spectroscopy (EIS) was performed with an AC amplitude of 50 mV and at a frequency of 0.1 Hz to 100 kHz. The Mott–Schottky (M–S) plot of the sample MF-1 was measured in the potential range of −0.4 to 0.8 V (vs. Ag/AgCl) with a potential step at 500 Hz frequency. The conversion of the potential E vs. Ag/AgCl (pH 6.5) to E vs. normal hydrogen electrode (NHE, pH 7.0) was based on eqn (6). The conduction band energy (ECB) was calculated based on eqn (7).36 | ENHE,pH 7 = E(Ag/AgCl,pH 6.5) + ΔV − 0.059 × (7.0 − 6.5) | (6) |
where ΔV = 0.21 V refers to the Ag/AgCl potential against NHE, ECB is the conduction band potential, EVB represents the valence band potential and Eg is the band gap energy (eV).
3. Results and discussion
3.1. XRD analysis
Fig. 1a shows the XRD pattern of the pristine MoS2 (M), which exhibits a typical diffraction pattern of MoS2 (JCPDS no. 75-1539). The peaks at 14.2°, 32.1°, 34.9°, 43.7° and 57.0° correspond to the (002), (100), (102), (006) and (110) lattice planes of MoS2, respectively.37 A distinctive peak of (002) at 14.2° indicates that the S–Mo–S layers are stacked in an ordered manner.38 On the other hand, the (002) plane of MF (Fig. 1b) shifted to a lower angle (8.1°). Interestingly, a new peak at 16.2° corresponding to the (004) plane emerged. This suggests that the interlayer spacing of MoS2 had expanded. This is in concordance with a previous study,39 in which the peak of the expanded MoS2 at 14.4° shifted to 8.9° while a new peak at 17.8° corresponding to the (004) plane was observed. The relationship between the peak shifting and the expansion of interlayer spaces was further verified through calculation of the d-spacing.
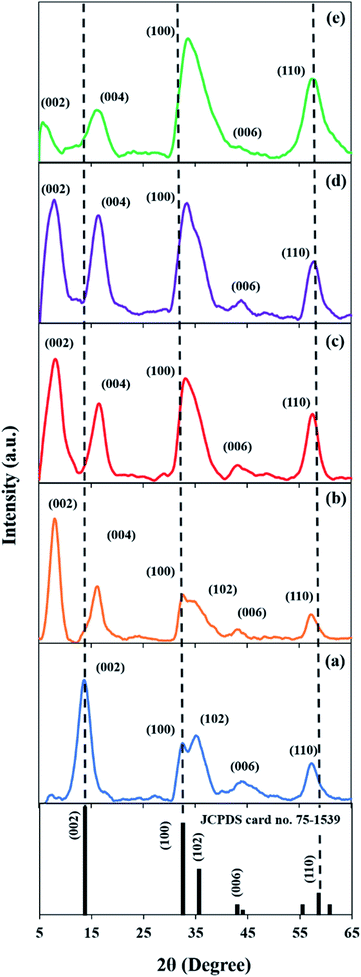 |
| Fig. 1 XRD patterns of (a) M, (b) MF, (c) MF-5, (d) MF-3, and (e) MF-1. | |
Based on Table 1, the intrinsic d-spacing of M is 0.62 nm. Meanwhile, the d-spacing of MF is 1.09 nm, which is 1.75 times higher than that of M. This occurrence is due to the intercalation of F-127 surfactant between the crystal lattice, which caused the MoS2 lattice structure to expand. This was also observed by Chen et al.,40 as the usage of cetyltrimethylammonium bromide (CTAB) surfactant successfully expanded the MoS2 interspacing to 56%. Notably, MF-1 exhibited the largest expanded interlayer spacing of 1.51 nm, which is more than double that of M. This very large lattice expansion will cause MF-1 to undergo uniaxial lattice strain along the [001] direction and thus induces in-plane compression (Fig. 2). It is noteworthy that the (002) peaks of the samples were broadened, indicating changes in the crystallite size. By using the Scherrer equation (eqn (4)), the FWHM for the (002) diffraction peak was calculated (Table 1). It can be observed that the samples with expanded interlayer spacings have much lower crystallite sizes compared to sample M, which also indicates the presence of disordered and shorter-range structures in samples MF, MF-5, MF-3 and MF-1.
Table 1 Properties of the synthesized MoS2 samples
Sample |
Interlayer spacing (nm) |
Crystallite size (nm) |
B/A ratio |
S/Mo ratio |
Mo4+/Mo6+ |
M |
0.62 |
13.55 |
0.57 |
2.0 |
— |
MF |
1.09 |
3.85 |
0.59 |
1.7 |
3.4 |
MF-5 |
1.05 |
4.86 |
0.59 |
1.6 |
2.4 |
MF-3 |
1.07 |
3.37 |
0.65 |
1.7 |
3.1 |
MF-1 |
1.51 |
3.88 |
0.66 |
1.4 |
1.2 |
 |
| Fig. 2 Schematic of sample M and the expansion of the d-spacing of sample MF-1. | |
3.2. Morphology
Fig. 3 shows the images and morphologies of the synthesized MoS2 samples. The mechanism of MoS2 hydrothermal treatment was initialized with the nucleation and crystallization process. This led to the formation of MoS2 nanosheets, followed by the gradual self-assembly of these nanosheets into a coral structure (Fig. 3a). When F-127 was introduced, the coral structure of MF (Fig. 3b) was less packed compared to that of M. This is because the non-ionic surfactant (F-127) introduced a good dispersing effect, which prevents the particles from clumping together. This hinders the formation of a highly packed structure. Furthermore, the structure of MoS2 changes drastically from a coral shape to an irregular shape as the pH becomes more acidic. At low pH, the hydroxyl groups on the two hydrophilic tails of the F-127 surfactant are protonated, which creates an electrostatic interaction with the Mo ions.22 This inhibits the self-assembly process; thus, a random distribution of MoS2 plate-structures was formed in MF-1 (Fig. 3e).
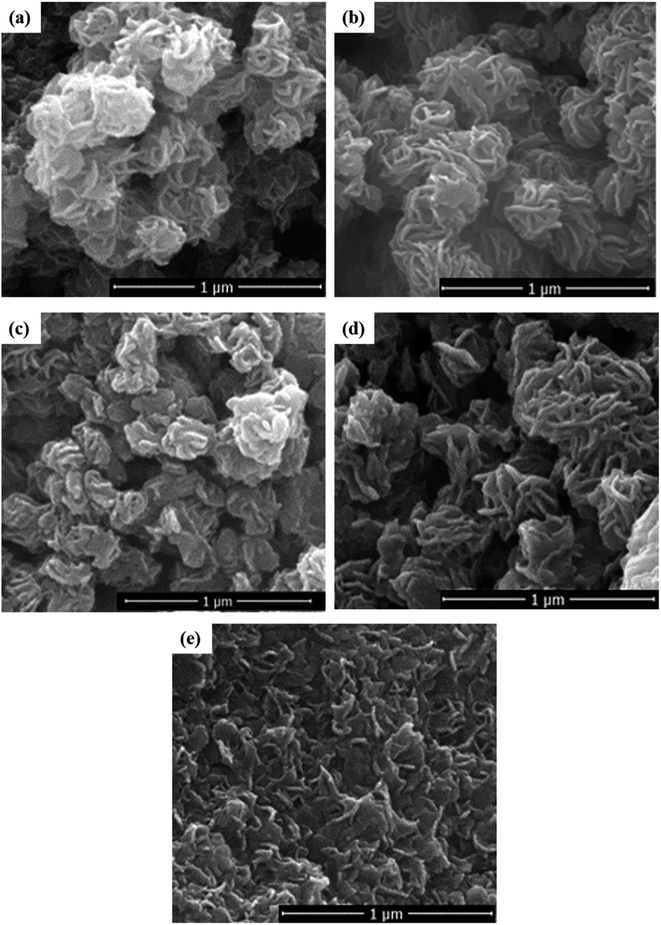 |
| Fig. 3 FESEM images of (a) M, (b) MF, (c) MF-5, (d) MF-3, and (e) MF-1. | |
Fig. 4(a) and (b) show that the HRTEM microstructures of both M and MF are composed of highly dense MoS2 nanosheets with wrinkled surfaces that are extruded out like lamellar structures. On the other hand, the microstructure of MF-1 (Fig. 4c) is loosely and randomly arranged, which agrees with the FESEM image (Fig. 3e). At higher magnification (Fig. 4d–f), it can be observed that the stacking layer increased in the order of M > MF > MF-1. Additionally, the measured interlayer spacing distances of M, MF and MF-1 are 0.62, 1.09 and 1.51 nm, respectively (inset Fig. 4d–f), which coincides with the d-spacing values calculated from XRD. Notably, the low stacking layer of MF-1 is randomly arranged, is oriented in a disorderly fashion and contains a dislocated edge (encircled in Fig. 4f). The presence of F-127 surfactant under very acidic conditions (pH 1) induces greater expansion of the lattice structure along the c-axis and results in the formation of surface defects. This enhances the edge site exposure and thus provides additional catalytic active sites, benefitting the photocatalytic activity. Additionally, MF-1 has lower stacking layers, which can be proved by the greater downward shift of the (002) peak compared with that of M and MF. This is in agreement with Panigrahi and Pathak,41 who reported that the (002) peak was shifted from 14.0° to 13.4°. Therefore, MF-1 consists of loosely assembled structures, low stacking layers, and a greater number of vertically disordered and defect structures, which signifies that MF-1 demonstrates higher active edge site exposure compared to the other samples.
 |
| Fig. 4 Low resolution TEM images of (a) M, (b) MF and (c) MF-1 and high resolution TEM images of (d) M, (e) MF and (f) MF-1 with measured stacking layers and interlayer distances (inset). | |
3.3 Raman spectra
As shown in Fig. 5, the Raman spectra of all the samples possess typical vibration modes of E12g (378.3 cm−1) and A1g (405.9 cm−1) peaks for MoS2. This corresponds to the in-plane and out-of-plane vibrations of 2H-MoS2, respectively.42 All the samples possess a similar feature, whereby the excitation of the A1g peak mode is higher than the E21g peak mode. This indicates that the surfaces of M, MF, MF-5, MF-3 and MF-1 are dominated by the terminated edges.23 The frequency differences between the E12g and A1g peaks also provide information regarding the stacking layers.24,33 The frequency differences between the E12g and A1g peaks decreased gradually in the order of M > MF > MF-5 > MF-3 > MF-1. This indicates that MF-1 has the fewest stacking layers, as confirmed by the HRTEM results. Moreover, there is an additional peak at ∼820 cm−1 which is attributed to the bonding of Mo and O atoms43 in samples MF, MF-5, MF-3 and MF-1. The intensity of the peaks significantly increased when the pH of the synthesis conditions was further lowered. This is due to the oxidation of MoS2 nanosheets resulting from the expansion of the interlayer spacing.
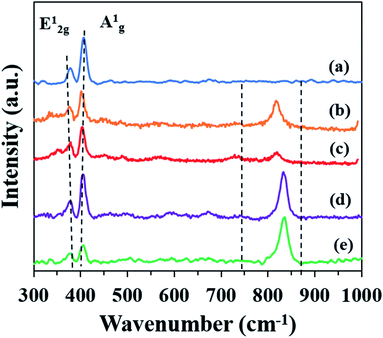 |
| Fig. 5 Raman spectra of (a) M, (b) MF, (c) MF-5, (d) MF-3, and (e) MF-1. | |
3.4. Optical properties
Fig. 6a depicts the UV-vis spectra of all the samples, and the tangent line drawn across the figure indicates the absorption edges of the respective samples. It can be observed that the samples have absorption edges within the range of 500 nm to 640 nm. This implies that the samples can be activated under the irradiation of LED light, which possesses a broad light spectrum ranging from 400 to 800 nm (Fig. S1†). Additionally, the measured band gap energy (Eg) of the samples (Fig. 6b) falls within 2.0–2.5 eV, which also indicates that the photocatalytic activity of the samples could be carried out under visible light irradiation. In Fig. 6c, the intensity of the PL decreases in the order of MF-1 > MF-3 > MF > MF-5 > M. This indicates that MF-1 exhibits lower stacking layers, which agrees with other reports.44,45 In other words, the MoS2 with lower stacking layers possesses stronger PL intensity, which is attributed to its stronger interaction with the light.46 Therefore, MF-1 has greater capability to absorb visible light.
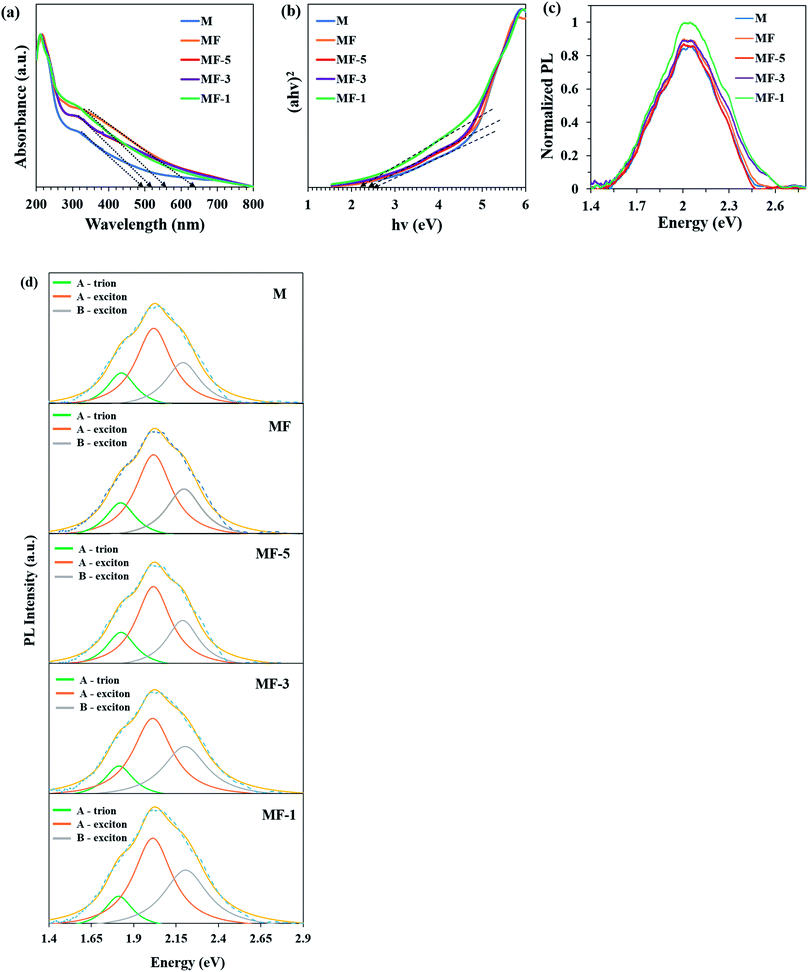 |
| Fig. 6 (a) UV-vis spectra and (b) band gaps of the samples. (c) Normalized PL peaks of the samples. (d) Deconvoluted PL peaks of the samples. | |
As shown in Fig. 6d, the PL peaks for all the samples were deconvoluted into three peaks through a Lorentzian function. The samples are fitted at 1.8, 2.0 and 2.2 eV, which correspond to the A-trions, A-exciton and B-exciton of MoS2 PL emission, respectively.21,47 The A-exciton and B-exciton are associated with the excitonic transition at the K-point of the Brillouin zone. Meanwhile, the A-trion peak was attributed to the transition resulting from charge impurities in the MoS2 layer.48 Technically, non-radiative recombination originating from defects will cause the A and B emission intensities to vary.49,50 To identify the defect densities, the B/A intensity ratios are recorded in Table 1. Notably, the B/A intensity ratio of MF-1 is ∼16% higher than that of M, which indicates that MF-1 possesses greater defects compared to the other samples.
3.5 Chemical composition and elemental states
Fig. 7 shows the XPS measurements of the elemental compositions and the oxidation states of the MoS2 samples. The Mo 3d spectrum of M consists of a set of doublet peaks which correspond to Mo4+ of the Mo 3d3/2 and Mo 3d5/2 peaks (Fig. 7a). Meanwhile, the Mo 3d spectra of MF, MF-5, MF-3 and MF-1 (Fig. 7b–e) can be fitted with two doublets. The doublets located at lower binding energies correspond to Mo4+ of Mo 3d3/2 and Mo 3d5/2, while the smaller doublets located at higher binding energies demonstrate the presence of partially oxidative Mo6+.51 This further proves the existence of MoS2 nanosheet oxidation observed in the Raman analysis. The occurrence of different Mo oxidation states indicates the presence of crystal defects in the samples. As for the S 2p peak, samples M, MF, MF-5, MF-3 and MF-1 show similar doublet peaks, attributed to S 2p3/2 and S 2p1/2 of S2− for MoS2 (Fig. 7f–j).
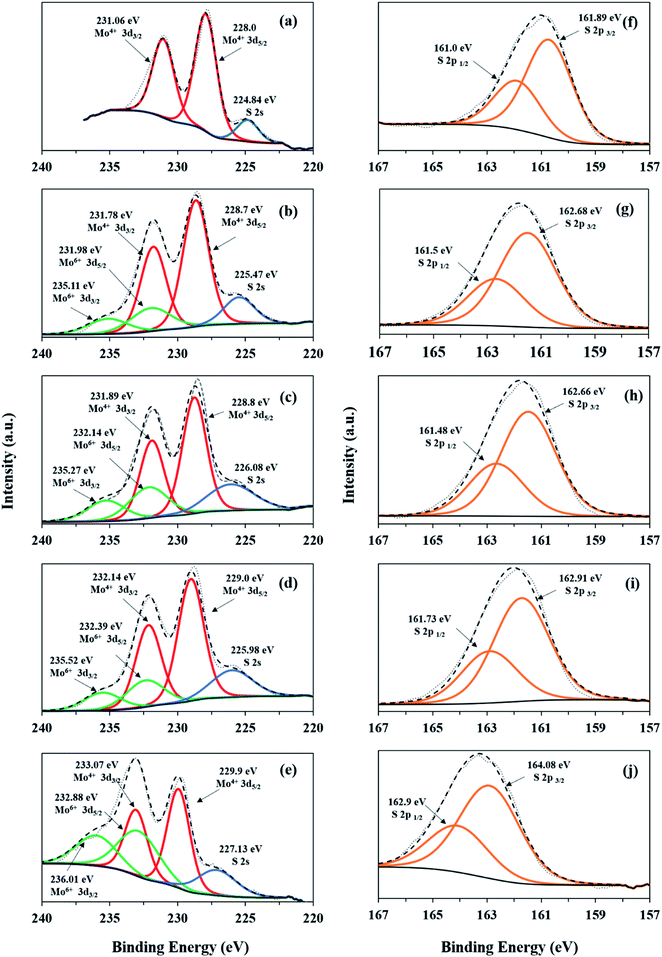 |
| Fig. 7 XPS spectra: Mo 3d and S 2p core level regions of M (a and f), MF (b and g), MF-5 (c and h), MF-3 (d and i), and MF-1 (e and j). | |
The stoichiometric ratios (S/Mo) of the samples were calculated from the XPS data using the major peak areas of Mo 3d and S 2p, which are assigned to MoS2. According to Table 1, the S/Mo ratio of M is equal to 2, which corresponds to the pristine MoS2. However, MF, MF-5, MF-3 and MF-1 possess S/Mo ratios less than 2. This indicates that the samples synthesized in the presence of surfactant and low pH conditions thus contain unsaturated Mo atoms. This condition also implies the presence of S vacancies in samples MF, MF-5, MF-3 and MF-1. Notably, MF-1 has the lowest S
:
Mo ratio, which indicates that the Mo atoms in MF-1 are highly unsaturated. This is because in an acidic environment, the S atoms in MF-1 can be easily etched, which leads to the formation of Sv sites. Formation of Sv in MoS2 can cause the electrons to accumulate at the vacancy sites, acting as trapping centers for photogenerated electron–hole pairs.48 This will induce the formation of A-trions in the PL emissions. However, oxygen and moisture in the atmosphere tend to adsorb on the Sv sites and then act as charge transfer channels. This will remove the excess electrons accumulated at the vacancy sites and thus enhance the PL intensity by subsequently converting the A-trions to A-excitons.52 This can be observed by the weaker A-trion peak and higher PL intensity of MF-1 compared to the other samples.
Moreover, the unsaturated Mo atoms at the Sv sites of MF, MF-5, MF-3 and MF-1 are highly vulnerable to oxidation in aqueous solution, forming Mo6+ species such as HMoO4− and MoO42−.53 Additionally, MoO3 may form due to bonding of O2 with the Sv sites. Therefore, the Mo6+ observed in Mo 3d is assigned to the Mo–O bonding, which is detected in the PL (Fig. 6b).54 The Mo4+/Mo6+ ratios of MF, MF-5, MF-3 and MF-1 decrease with increasing interlayer spacing. The decrease of the Mo4+/Mo6+ ratio indicates that more O2 molecules were adsorbed onto the Sv sites, forming Mo–O bonds. Thus, the presence of Sv provides additional adsorption sites for O2 molecules through Lewis acid–base interactions. This could efficiently reduce the O2 to reactive oxygen species (ROS), such as superoxide radicals (˙O2−).55 Because MF-1 possesses the lowest Mo4+/Mo6+ ratio, MF-1 is capable of forming and weakening O2 molecules to form ROS species.
3.6 Photodegradation of MB
Fig. 8a shows the photocatalytic activity of the expanded MoS2 under the illumination of 1 W LED white light. The photolysis of MB dye without the presence of photocatalyst was negligible. It is interesting to observe that MF-1 shows the highest photodegradation of MB dye. MF-1 manages to photodegrade up to 98% of the MB dye within 120 min under LED white light irradiation. The photodegradation activity of the samples decreases as follows: MF-1 (k = 2.62 × 10−2 min−1) > MF-3 (1.85 × 10−2 min−1) > MF (1.08 × 10−2 min−1) > M (9.4 × 10−3 min−1) > MF-5 (8.2 × 10−3 min−1). All the samples are easily activated under visible LED white light and can photodegrade the dye within 120 min.
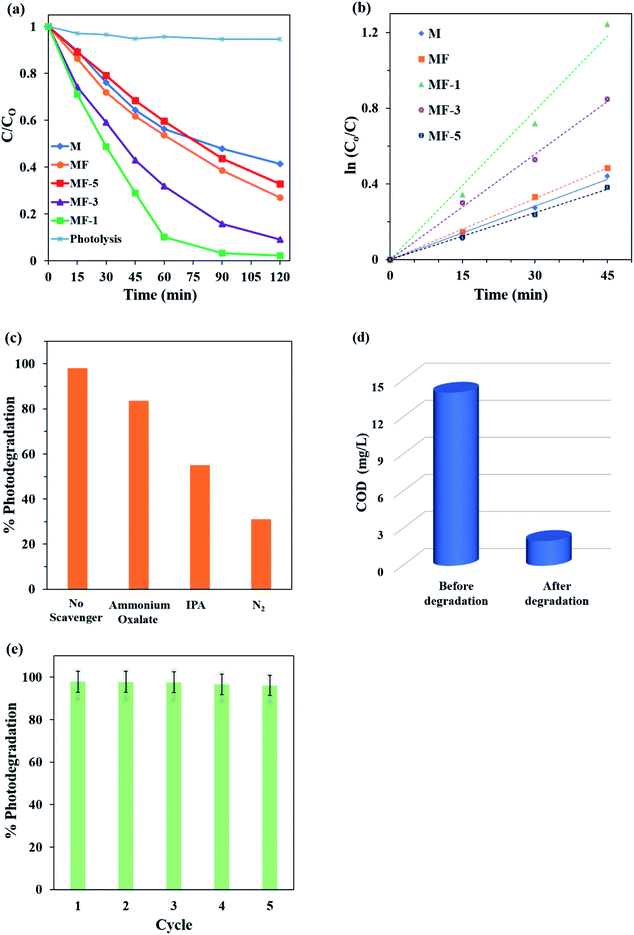 |
| Fig. 8 Comparisons of the (a) photocatalytic performance and (b) pseudo-first-order kinetics of samples M, MF, MF-5 and MF-1. (c) Percentages of photodegradation in the presence of different scavengers. (d) Percentages of COD before and after degradation of MB by MF-1. (e) Recyclability performance of MF-1. | |
According to the Langmuir–Hinshelwood model, the photodegradation process is well fitted with pseudo-first-order kinetics (Fig. 8b). It is noteworthy that the photodegradation of MF is 14% higher than that of M. As expected, the expanded MoS2 layer of MF has reduced stacking layers; thus, more active edge sites are exposed for photocatalytic activity. However, MF-1 is 39% and 25% more active than M and MF, respectively. This is mainly due to its highly expanded interlayer spaces, which are about 2.4 and 1.38 times higher than those of M and MF. The highly expanded MF-1 has caused the adjacent layers of MoS2 to decouple, thus causing the individual monolayer to act like a free single MoS2 layer. Decoupling of the interlayer spacing will occur when the interlayer is highly expanded.56 The decoupling of the interlayer spacing in MF-1 will increase the edge site exposure, providing more catalytic active sites for the photodegradation of MB dye. Therefore, MF-1 with the largest expansion of interlayer spacing will have the greatest performance in the photocatalytic degradation of MB dye.
The formation of surface defects also served to create additional edge sites for the photocatalytic activity of MF-1. This is because the internal surface of the MoS2 layer is more accessible, which agrees well with Xie et al.28 They discovered that defects induced additional active edge sites and increased the HER activity by 9 fold. In this study, the presence of Sv in MF-1 facilitated the generation of ROS from O2. Generally, the adsorption of O2 on pristine MoS2 is governed by low adsorption energy, which is insufficient to activate O2 molecule to form ROS. This is due to its large inactive basal plane surface without Mo dangling bonds.57 However, surface defects such as cracking and Sv have enhanced the exposure of Mo active metal, providing larger adsorption energy for O2 molecules. This promotes the migration of electrons from the electron-rich Mo atoms to the adsorbed O2 molecules, thus generating more ROS such as superoxide radicals (˙O2−) and hydroxyl radical (˙OH) for the photodegradation of MB dye. This was proven by Sarkar and co-workers, who showed that MoS2 that is rich with Mo is highly effective in the generation of ROS.58 Therefore, the highly expanded interlayer spacing of MF-1 with lower stacking layers, high edge site exposure, rich defects and oxygen passivation of Sv enabled it to possess high photocatalytic activity under LED visible light irradiation.
3.7 Photoelectrochemical (PEC) measurements
Transient photocurrent–time measurements of the samples were performed under the irradiation of visible light with four on–off cycles. As shown in Fig. 9a, all the samples showed instant photocurrent response upon illumination of light that diminished instantly when the light was shut off. This also agrees with a previous study,59 which implies steady photocatalytic activity. Among the samples, MF-1 depicts the highest photocurrent response without a dramatic reduction even after four consecutive cycles. This proves that MF-1 with highly expanded interlayer spacing has greater photoresponse in generating and separating photogenerated charge carriers. Moreover, in the EIS Nyquist plots (Fig. 9b), MF-1 possesses the smallest arc radius of its semi-circle. This implies that MF-1 experiences the lowest charge transfer resistance. Therefore, MF-1 with low charge transfer resistance and high separation of photogenerated charge manages to enhance the photodegradation of MB dye.
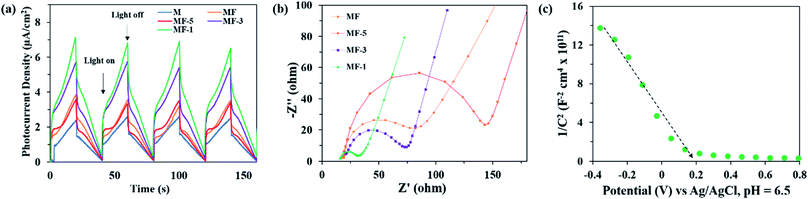 |
| Fig. 9 (a) Transient photocurrent and (b) electron impedance spectroscopy of the samples; (c) Mott–Schottky plot of sample MF-1. | |
The Mott–Schottky (M–S) plot of MF-1 (Fig. 9c) demonstrates a negative slope, which suggests that MF-1 is a p-type semiconductor. By extrapolating the M–S plot to 1/C2 = 0, the flat band potential (EFB) of MF-1 is 0.2 V (V vs. Ag/AgCl, pH 6.5). In general, a p-type semiconductor has EFB of approximately 0.3 V above the EVB; thus, the EVB of MF-1 is 0.5 V (vs. Ag/AgCl, pH 6.5).60 Using eqn (6) and (7), the calculated EVB (vs. NHE, pH 7) and ECB (vs. NHE, pH 7) of MF-1 were +0.7 V and −1.5 V, respectively. Based on these data, the band energy diagram of MF-1 is illustrated in Fig. 10.
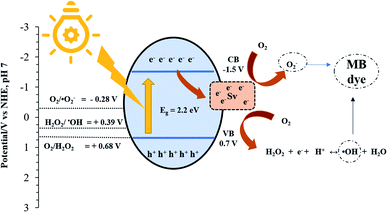 |
| Fig. 10 Schematic of the photodegradation of MB over MF-1. | |
3.8 Proposed photodegradation mechanism
Fig. 10 displays the proposed photodegradation mechanism. Upon illumination of light, electrons (e−) and holes (h+) are photogenerated on the surface of the MF-1 photocatalyst (eqn (8)). Then, the photogenerated electrons are transferred to the conduction band (CB) and migrate to the Sv sites, which act as trapping sites for the photogenerated electrons. This greatly prevents the photogenerated charges from recombining and thus prolongs the lifespan of the redox reaction. At the Sv sites, the electron-rich Mo atoms possess high adsorption energy with O2 molecules, which facilitates the reduction of O2 molecules to ˙O2− radicals [E0 (O2/˙O2−) = −0.284 V] (eqn (9)).58 It is noteworthy that the adsorbed H2O could not undergo oxidation to form ˙OH radicals, as the valence band (VB) position of MF-1 (EVB = 0.7 eV) is less positive than the water oxidation potential [E0 (˙OH/OH−) = +2.38 V]. However, the ˙OH radical can still be generated when the adsorbed O2 undergoes multi-electron and proton redox reactions (eqn (10) and (11)).61 The chemical equations involved are described below: | MF-1 + hv → eCB− + hVB+ | (8) |
| O2 + 2e− + 2H+ → H2O2 | (10) |
| H2O2 + e− + H+ → ˙OH + H2O | (11) |
˙O2−, ˙OH, h+ + MB dye → CO2 + H2O + inorganic molecules |
In order to verify the proposed mechanism, free radical trapping experiments were conducted using ammonium oxalate and isopropyl alcohol to trap h+ and ˙OH radicals, respectively. Pure N2 was also used as a scavenger and was purged throughout the photocatalytic reaction to restrain the generation of ˙O2− radical.62 As shown in Fig. 8c, the presence of N2 gas dramatically decreased the photocatalytic activity to 31%, while in the presence of isopropyl and ammonium oxalate, the photocatalytic activity was reduced to 55% and 83%, respectively. Therefore, this experiment confirmed that ˙O2− radical plays the most important role in the photocatalytic degradation of MB dye, followed by ˙OH and h+ radicals. This further justifies that the presence of Sv facilitates the transfer of electrons from MF-1 to O2 and reduces O2 to ˙O2− radicals.
Table 2 Comparative study of the photodegradation activity of MoS2 materials
Materials |
Light source |
Dye |
Catalyst dosage (g L−1) |
Concentration of dye (mg L−1) |
Degradation percentage (%) |
Time taken (min) |
Ref. |
MoS2 |
100 W Xe |
MB |
0.2 |
10 |
89.2 |
150 |
59
|
(1T/2H) MoS2 |
400 W W |
MO |
0.5 |
20 |
95 |
120 |
60
|
TiO2/MoS2 |
500 W Xe |
RhB |
0.1 |
5 |
97.8 |
180 |
61
|
PbBiO2I/MoS2 |
300 W Xe |
RhB |
0.3 |
10 |
99 |
180 |
62
|
BiSI/MoS2 |
250 W Mg |
CV |
0.5 |
5 |
90 |
250 |
63
|
MoS2/Zn0.5Cd0.5S |
300 W Xe |
RhB |
0.5 |
10 |
74 |
120 |
64
|
MoS2 |
1 W LED |
MB |
0.2 |
20 |
98 |
120 |
This work |
Based on Fig. 8d, the COD value of the MB dye solution plummeted from the original value of 14 mg L−1 to 2 mg L−1 within 2 h of photocatalytic degradation by MF-1. The 86% COD removal efficiency is sufficient to prove that MF-1 is a promising photocatalyst in the application of dye wastewater treatment. Moreover, the stability of a photocatalyst is an important factor to obtain a highly reusable photocatalyst (Fig. 8e). It can be clearly seen that the photocatalytic degradation does not decelerate dramatically after five consecutive cycles. This indicates that MF-1 is highly stable even after being reused for five cycles. In comparison with previous reported studies (Table 2), highly expanded MoS2 in our work prove that MoS2 can acts as photocatalyst with great photocatalytic activity.65–68 Therefore, it can be concluded that the highly expanded interlayer spaces of MF-1 show remarkable photoactivity by using a more energy-efficient light source and demonstrate high stability.
4. Conclusion
In summary, a highly expanded MoS2 photocatalyst exhibits high photoactivity under 1 W LED visible light irradiation. The incorporation of F-127 non-ionic surfactant under acidic conditions, especially at pH 1, manages to produce highly expanded MoS2 with interlayer spaces of 1.51 nm. The exposure of the active edge sites from the highly expanded MF-1 sample has greatly enhanced the photocatalytic performance by 1.7 times compared to pristine MoS2. Moreover, oxygen passivation of Sv improves the optical absorption of the photocatalyst under the visible light irradiation and enhances the generation of ˙O2− radicals for the photocatalytic reaction. Therefore, due the highly expanded interlayer spacing of MF-1 with high reusability, it could potentially serve as a long-term-usage photocatalyst under energy-efficient LED white light to remove dyes pollutants from wastewater.
Conflicts of interest
There are no conflicts to declare.
Acknowledgements
This study was funded by the University of Malaya under SATU grant (ST011-2018 & ST020-2019) from the University of Malaya. We also would like to acknowledge National Taipei University of Technology-University of Malaya Joint 5 Research Program (NTUT-UM-109-02) and Ministry of Science and Technology (MOST, 108-2221-E-027-072) for the financial support.
References
- C. D. Raman and S. Kanmani, Textile dye degradation using nano zero valent iron: A review, J. Environ. Manage., 2016, 177, 341–355 CrossRef CAS.
- B. Valley, B. Jing, M. Ferreira and Y. Zhu, Rapid and Efficient Coacervate Extraction of Cationic Industrial Dyes from Wastewater, ACS Appl. Mater. Interfaces, 2019, 11(7), 7472–7478 CrossRef CAS.
-
Sustainable Development Goals (SDG), Goal 6: Clean water and sanitation, Retrieved https://www.undp.org/content/undp/en/home/copyright-and-termsofuse.html July 9, 2020, Search PubMed.
- L. Liu, Z. Y. Gao, X. P. Su, X. Chen, L. Jiang and J. M. Yao, Adsorption Removal of Dyes from Single and Binary Solutions Using a Cellulose-based Bioadsorbent, ACS Sustainable Chem. Eng., 2015, 3(3), 432–442 CrossRef CAS.
- A. D. Liyanage, S. D. Perera, K. Tan, Y. Chabal and K. J. Balkus, Synthesis, Characterization, and Photocatalytic Activity of Y-Doped CeO2 Nanorods, ACS Catal., 2014, 4(2), 577–584 CrossRef CAS.
- R. Javaid and U. Y. Qazi, Catalytic Oxidation Process for the Degradation of Synthetic Dyes: An Overview, Int. J. Environ. Res. Public Health, 2019, 16, 11 Search PubMed.
- A. M. Lotito, U. Fratino, A. Mancini, G. Bergna and C. Di Iaconi, Effective aerobic granular sludge treatment of a real dyeing textile wastewater, Int. Biodeterior. Biodegrad., 2012, 69, 62–68 CrossRef CAS.
- Y. Wang, X. Ye, G. Chen, D. Li, S. Meng and S. Chen, Synthesis of BiPO4 by crystallization and hydroxylation with boosted photocatalytic removal of organic pollutants in air and water, J. Hazard. Mater., 2020, 399, 122999 CrossRef CAS.
- M. T. L. Lai, C. W. Lai, K. M. Lee, S. W. Chook, T. C. K. Yang, S. H. Chong and J. C. Juan, Facile one-pot solvothermal method to synthesize solar active Bi2WO6 for photocatalytic degradation of organic dye, J. Alloys Compd., 2019, 801, 502–510 CrossRef CAS.
- N. Rahimi, R. A. Pax and E. M. Gray, Review of functional titanium oxides. I: TiO2 and its modifications, Prog. Solid State Chem., 2016, 44(3), 86–105 CrossRef CAS.
- W.-K. Jo and R. J. Tayade, New Generation Energy-Efficient Light Source for Photocatalysis: LEDs for Environmental Applications, Ind. Eng. Chem. Res., 2014, 53(6), 2073–2084 CrossRef CAS.
- D. Wu, W. Wang, T. W. Ng, G. Huang, D. Xia, H. Y. Yip, H. K. Lee, G. Li, T. An and P. K. Wong, Visible-light-driven photocatalytic bacterial inactivation and the mechanism of zinc oxysulfide under LED light irradiation, J. Mater. Chem. A, 2016, 4(3), 1052–1059 RSC.
- K. Dai, L. Lu, J. Dong, Z. Ji, G. Zhu, Q. Liu, Z. Liu, Y. Zhang, D. Li and C. Liang, Facile synthesis of a surface plasmon resonance-enhanced Ag/AgBr heterostructure and its photocatalytic performance with 450 nm LED illumination, Dalton Trans., 2013, 42(13), 4657–4662 RSC.
- Q. Ou, S. Xu, Y. Long and X. Zhang, Porous visible light-responsive Fe3+-doped carbon nitride for efficient degradation of sulfadiazine, Environ. Sci. Pollut. Res., 2020, 27(22), 27849–27858 CrossRef CAS.
- K. C. Devarayapalli, S. V. Prabhakar Vattikuti, T. V. Madhukar Sreekanth, P. Chidanandha Nagajyothi and J. Shim, Pyrolysis-Synthesized g-C3N4/Nb2O5 Nanocomposite for Enhanced Photocatalytic Activity under White LED Light Irradiation, ChemistrySelect, 2019, 4(45), 13250–13258 CrossRef CAS.
- Z. Li, X. Meng and Z. Zhang, Recent development on MoS2-based photocatalysis: A review, J. Photochem. Photobiol., C, 2018, 35, 39–55 CrossRef CAS.
- Z. Liang, R. Shen, Y. H. Ng, P. Zhang, Q. Xiang and X. Li, A review on 2D MoS2 cocatalysts in photocatalytic H2 production, J. Mater. Sci. Technol., 2020, 56, 89–121 CrossRef.
- Y. Liu, Y. Xie, L. Liu and J. Jiao, Sulfur vacancy induced high performance for photocatalytic H2 production over 1T@2H phase MoS2 nanolayers, Catal. Sci. Technol., 2017, 7(23), 5635–5643 RSC.
- Y. Okuno, O. Lancry, A. Tempez, C. Cairone, M. Bosi, F. Fabbri and M. Chaigneau, Probing the nanoscale light emission properties of a CVD-grown MoS2 monolayer by tip-enhanced photoluminescence, Nanoscale, 2018, 10(29), 14055–14059 RSC.
- G. Deokar, N. S. Rajput, P. Vancso, F. Ravaux, M. Jouiad, D. Vignaud, F. Cecchet and J. F. Colomer, Large area growth of vertically aligned luminescent MoS2 nanosheets, Nanoscale, 2017, 9(1), 277–287 RSC.
- G. Pradhan and A. K. Sharma, Anomalous Raman and photoluminescence blue shift in mono- and a few layered pulsed laser deposited MoS2 thin films, Mater. Res. Bull., 2018, 102, 406–411 CrossRef CAS.
- M. Li, D. Wang, J. Li, Z. Pan, H. Ma, Y. Jiang, Z. Tian and A. Lu, Surfactant-assisted hydrothermally synthesized MoS2 samples with controllable morphologies and structures for anthracene hydrogenation, Chin. J. Catal., 2017, 38(3), 597–606 CrossRef CAS.
- H. Wang, Z. Lu, D. Kong, J. Sun, T. M. Hymel and Y. Cui, Electrochemical Tuning of MoS2 Nanoparticles on Three-Dimensional Substrate for Efficient Hydrogen Evolution, ACS Nano, 2014, 8, 5 CrossRef.
- Y. C. Dong, S.-K. Park, Y.-H. Chung, S.-H. Yu, D.-H. Lim, N. Jung, H. C. Ham, H.-Y. Park, Y. Piao, S. J. Yoo and Y.-E. Sung, Edge-exposed MoS2 nano-assembled structures as efficient electrocatalysts for hydrogen evolution reaction, Nanoscale, 2014, 6, 2131–2136 RSC.
- H. K. Sadhanala, S. Senapati, K. V. Harika, K. K. Nanda and A. Gedanken, Green synthesis of MoS2 nanoflowers for efficient degradation of methylene blue and crystal violet dyes under natural sun light conditions, New J. Chem., 2018, 42(17), 14318–14324 RSC.
- J. Kibsgaard, Z. Chen, B. N. Reinecke and T. F. Jaramillo, Engineering the surface structure of MoS2 to preferentially expose active edge sites for electrocatalysis, Nat. Mater., 2012, 11(11), 963–969 CrossRef CAS.
- C. G. Morales-Guio and X. Hu, Amorphous molybdenum sulfides as hydrogen evolution catalysts, Acc. Chem. Res., 2014, 47(8), 2671–2681 CrossRef CAS.
- J. Xie, H. Zhang, S. Li, R. Wang, X. Sun, M. Zhou, J. Zhou, X. W. Lou and Y. Xie, Defect-rich MoS2 ultrathin nanosheets with additional active edge sites for enhanced electrocatalytic hydrogen evolution, Adv. Mater., 2013, 25(40), 5807–5813 CrossRef CAS.
- M. R. Gao, M. K. Chan and Y. Sun, Edge-terminated molybdenum disulfide with a 9.4-A interlayer spacing for electrochemical hydrogen production, Nat. Commun., 2015, 6, 7493 CrossRef.
- M. Lin, G. Huang, W. Chen, Z. Wang, J. Ye, H. Li, D. Chen and J. Y. Lee, Cationic surfactant-assisted hydrothermal synthesis of few-layer molybdenum disulfide/graphene composites: Microstructure and electrochemical lithium storage, J. Power Sources, 2014, 264, 262–271 CrossRef.
- Q. Liu, X. Li, Q. He, A. Khalil, D. Liu, T. Xiang, X. Wu and L. Song, Gram-Scale Aqueous Synthesis of Stable Few-Layered 1T-MoS2 : Applications for Visible-Light-Driven Photocatalytic Hydrogen Evolution, Small, 2015, 11(41), 5556–5564 CrossRef CAS.
- J. Zhou, G. Fang, A. Pan and S. Liang, Oxygen-Incorporated MoS2 Nanosheets with Expanded Interlayers for Hydrogen Evolution Reaction and Pseudocapacitor Applications, ACS Appl. Mater. Interfaces, 2016, 8(49), 33681–33689 CrossRef CAS.
- X. Zeng, L. Niu, L. Song, X. Wang, X. Shi and J. Yan, Effect of Polymer Addition on the Structure and Hydrogen Evolution Reaction Property of Nanoflower-Like Molybdenum Disulfide, Metals, 2015, 5(4), 1829–1844 CrossRef.
- J. Shao, Q. Qu, Z. Wan, T. Gao, Z. Zuo and H. Zheng, From Dispersed Microspheres to Interconnected Nanospheres: Carbon-Sandwiched Monolayered MoS2 as High-Performance Anode of Li-Ion Batteries, ACS Appl. Mater. Interfaces, 2015, 7, 22927–22934 CrossRef CAS.
- R. M. Mohamed and F. A. Harraz, Mechanistic investigation and photocatalytic activity of yttrium vanadate (YVO4) nanoparticles for organic pollutants mineralization, J. Mater. Res. Technol., 2020, 9(3), 5666–5675 CrossRef CAS.
- T. Giannakopoulou, I. Papailias, N. Todorova, N. Boukos, Y. Liu, J. Yu and C. Trapalis, Tailoring the energy band gap and edges' potentials of g-C3N4/TiO2 composite photocatalysts for NOx removal, Chem. Eng. J., 2017, 310, 571–580 CrossRef CAS.
- B. Mao, B. Wang, F. Yu, K. Zhang, Z. Zhang, J. Hao, J. Zhong, Y. Liu and W. Shi, Hierarchical MoS2 nanoflowers on carbon cloth as an efficient cathode electrode for hydrogen evolution under all pH values, Int. J. Hydrogen Energy, 2018, 43, 11038–11046 CrossRef CAS.
- J. Shao, Q. Qu, Z. Wan, T. Gao, Z. Zuo and H. Zheng, From Dispersed Microspheres to Interconnected Nanospheres: Carbon-Sandwiched Monolayered MoS2 as High-Performance Anode of Li-Ion Batteries, ACS Appl. Mater. Interfaces, 2015, 7(41), 22927–22934 CrossRef CAS.
- H. Dong, Y. Xu, C. Zhang, Y. Wu, M. Zhou, L. Liu, Y. Dong, Q. Fu, M. Wu and Y. Lei, MoS2 nanosheets with expanded interlayer spacing for enhanced sodium storage, Inorg. Chem. Front., 2018, 5(12), 3099–3105 RSC.
- J. Chen, Y. Xia and J. Yang, Graphene/surfactant-assisted synthesis of edge-terminated molybdenum disulfide with enlarged interlayer spacing, Mater. Lett., 2018, 210, 248–251 CrossRef CAS.
- P. K. Panigrahi and A. Pathak, Aqueous Medium Synthesis Route for Randomly Stacked Molybdenum Disulfide, J. Nanopart., 2013, 2013, 1–10 Search PubMed.
- J. G. Kim, W. S. Yun, S. Jo, J. D. Lee and C.-H. Cho, Effect of interlayer interactions on exciton luminescence in atomic-layered MoS2 crystals, Sci. Rep., 2016, 6, 1 CrossRef.
- S. Hussain, J. Singh, D. Vikraman, A. K. Singh, M. Z. Iqbal, M. F. Khan, P. Kumar, D. C. Choi, W. Song, K. S. An, J. Eom, W. G. Lee and J. Jung, Large-area, continuous and high electrical performances of bilayer to few layers MoS2 fabricated by RF sputtering via post-deposition annealing method, Sci. Rep., 2016, 6, 30791 CrossRef CAS.
- M. A. Islam, J. Church, C. Han, H. S. Chung, E. Ji, J. H. Kim, N. Choudhary, G. H. Lee, W. H. Lee and Y. Jung, Noble metal-coated MoS2 nanofilms with vertically-aligned 2D layers for visible light-driven photocatalytic degradation of emerging water contaminants, Sci. Rep., 2017, 7(1), 14944 CrossRef.
- S. Catalán-Gómez, S. Garg, A. Redondo-Cubero, N. Gordillo, A. de Andrés, F. Nucciarelli, S. Kim, P. Kung and J. L. Pau, Photoluminescence enhancement of monolayer MoS2 using plasmonic gallium nanoparticles, Nanoscale Adv., 2019, 1(2), 884–893 RSC.
- S. Catalán-Gómez, S. Garg, A. Redondo-Cubero, N. Gordillo, A. de Andrés, F. Nucciarelli, S. Kim, P. Kung and J. L. Pau, Photoluminescence enhancement of monolayer MoS2 using plasmonic gallium nanoparticles, Nanoscale Adv., 2019, 1, 884–893 RSC.
- M. R. Habib, H. Li, Y. Kong, T. Liang, S. M. Obaidulla, S. Xie, S. Wang, X. Ma, H. Su and M. Xu, Tunable photoluminescence in a van der Waals heterojunction built from a MoS2 monolayer and a PTCDA organic semiconductor, Nanoscale, 2018, 10(34), 16107–16115 RSC.
- L. P. L. Mawlong, K. K. Paul and P. K. Giri, Direct Chemical Vapor Deposition Growth of Monolayer MoS2 on TiO2 Nanorods and Evidence for Doping-Induced Strong Photoluminescence Enhancement, J. Phys. Chem. C, 2018, 122, 15017–15025 CrossRef CAS.
- K. M. McCreary, A. T. Hanbicki, S. V. Sivaram and B. T. Jonker, A- and B-exciton photoluminescence intensity ratio as a measure of sample quality for transition metal dichalcogenide monolayers, APL Mater., 2018, 6, 111106 CrossRef.
- T. Verhagen, V. L. P. Guerra, G. Haider, M. Kalbac and J. Vejpravova, Towards the evaluation of defects in MoS2 using cryogenic photoluminescence spectroscopy, Nanoscale, 2020, 12, 3019–3028 RSC.
- W. Su, P. Wang, Z. Cai, J. Yang and X. Wang, One-pot hydrothermal synthesis of Al-doped MoS2@graphene aerogel nanocomposite electrocatalysts for enhanced hydrogen evolution reaction, Results Phys., 2019, 12, 250–258 CrossRef.
- L. Xu, L. Zhao, Y. Wang, M. Zou, Q. Zhang and A. Cao, Analysis of photoluminescence behavior of high-quality single-layer MoS2, Nano Res., 2019, 12(7), 1619–1624 CrossRef CAS.
- W. T. Yein, Q. Wang, Y. Liu, Y. Li, J. Jian and X. Wu, Piezo-potential induced molecular oxygen activation of defect-rich MoS2 ultrathin nanosheets for organic dye degradation in dark, J. Environ. Chem. Eng., 2020, 8(1), 103626 CrossRef CAS.
- H. Nan, Z. Wang, W. Wang, Z. Liang, Y. Lu, Q. Chen, D. He, P. Tan, F. Miao, X. Wang, J. Wang and Z. Ni, Strong Photoluminescence Enhancement of MoS2 through Defect Engineering and Oxygen Bonding, ACS Nano, 2014, 8(6), 5738–5745 CrossRef CAS.
- Y. Zheng, Z. Yu, H. Ou, A. M. Asiri, Y. Chen and X. Wang, Black Phosphorus and Polymeric Carbon Nitride Heterostructure for Photoinduced Molecular Oxygen Activation, Adv. Funct. Mater., 2018, 28(10), 1705407 CrossRef.
- Y. Zhang, H. Li, H. Wang, R. Liu, S.-L. Zhang and Z.-J. Qiu, On Valence-Band Splitting in Layered MoS2, ACS Nano, 2015, 9(8), 8514–8519 CrossRef CAS.
- B. B. Xiao, P. Zhang, L. P. Han and Z. Wen, Functional MoS2 by the Co/Ni doping as the catalyst for oxygen reduction reaction, Appl. Surf. Sci., 2015, 354, 221–228 CrossRef CAS.
- D. Sarkar, B. Mondal, A. Som, S. J. Ravindran, S. K. Jana, C. K. Manju and T. Pradeep, Holey MoS2 Nanosheets with Photocatalytic Metal Rich Edges by Ambient Electrospray Deposition for Solar Water Disinfection, Global Challenges, 2018, 2(12), 1800052 CrossRef.
- H. Qin, R. T. Guo, X. Y. Liu, W. G. Pan, Z. Y. Wang, X. Shi, J. Y. Tang and C. Y. Huang, Z-Scheme MoS2/g-C3N4 heterojunction for efficient visible light photocatalytic CO2 reduction, Dalton Trans., 2018, 47(42), 15155–15163 RSC.
- W. Yin, L. Bai, Y. Zhu, S. Zhong, L. Zhao, Z. Li and S. Bai, Embedding Metal in the Interface of a p–n Heterojunction with a Stack Design for Superior Z-Scheme Photocatalytic Hydrogen Evolution, ACS Appl. Mater. Interfaces, 2016, 8(35), 23133–23142 CrossRef CAS.
- T. B. Nguyen and R.-a. Doong, Fabrication of highly visible-light-responsive ZnFe2O4/TiO2 heterostructures for the enhanced photocatalytic degradation of organic dyes, RSC Adv., 2016, 6(105), 103428–103437 RSC.
- Y. Deng, L. Tang, G. Zeng, C. Feng, H. Dong, J. Wang, H. Feng, Y. Liu, Y. Zhou and Y. Pang, Plasmonic resonance excited dual Z-scheme BiVO4/Ag/Cu2O nanocomposite: synthesis and mechanism for enhanced photocatalytic performance in recalcitrant antibiotic degradation, Environ. Sci.: Nano, 2017, 4(7), 1494–1511 RSC.
- Z. Zhou, Y. Lin, P. Zhang, E. Ashalley, M. Shafa, H. Li, J. Wu and Z. Wang, Hydrothermal fabrication of porous MoS2 and its visible light photocatalytic properties, Mater. Lett., 2014, 131, 122–124 CrossRef CAS.
- M. R. Saber, G. Khabiri, A. A. Maarouf, M. Ulbricht and A. S. G. Khalil, A comparative study on the photocatalytic degradation of organic dyes using hybridized 1T/2H, 1T/3R and 2H MoS2 nano-sheets, RSC Adv., 2018, 8(46), 26364–26370 RSC.
- C. Wang, H. Lin, Z. Liu, J. Wu, Z. Xu and C. Zhang, Controlled Formation of TiO2/MoS2 Core-Shell Heterostructures with Enhanced Visible-Light Photocatalytic Activities, Part. Part. Syst. Charact., 2016, 33(4), 221–227 CrossRef CAS.
- M. Li, S. Yin, T. Wu, J. Di, M. Ji, B. Wang, Y. Chen, J. Xia and H. Li, Controlled preparation of MoS2/PbBiO2I hybrid microspheres with enhanced visible-light photocatalytic behaviour, J. Colloid Interface Sci., 2018, 517, 278–287 CrossRef CAS.
- S. Bargozideh and M. Tasviri, Construction of a novel BiSI/MoS2 nanocomposite with enhanced visible-light driven photocatalytic performance, New J. Chem., 2018, 42(22), 18236–18241 RSC.
- X.-L. Yin, S.-R. Han and L.-L. Li, MoS2/Zn0.5Cd0.5S hierarchical nano-heterostructure for efficient light absorption and photocatalytic pollution degradation, Optik, 2020, 212, 164680 CrossRef CAS.
Footnote |
† Electronic supplementary information (ESI) available. See DOI: 10.1039/d0na00936a |
|
This journal is © The Royal Society of Chemistry 2021 |
Click here to see how this site uses Cookies. View our privacy policy here.