DOI:
10.1039/D0QM00624F
(Research Article)
Mater. Chem. Front., 2021,
5, 284-292
Structural optimization of organic fluorophores for highly efficient photothermal therapy†
Received
25th August 2020
, Accepted 2nd October 2020
First published on 5th October 2020
Abstract
Developing highly efficient organic photothermal agents is desirable and imperative because of the great potential of photothermal therapy in tumor treatment. In this work, three boron dipyrromethene (BDP) molecules (Ph-BDP, Na-BDP and Py-BDP) with different conjugate areas were rationally designed and synthesized to study the effects of molecular structures on photoproperties. With the increase of conjugate areas, the absorption and emission wavelengths of BDP molecules were red-shifted, and the radiative transitions were weakened along with the improvement of non-radiative transitions and enhancement of intersystem crossing (ISC). After formation of BDP nanoparticles, the radiative transitions and ISC were weakened. Importantly, Py-BDP nanoparticles with the largest conjugate area had highest photothermal conversion efficiency (η = 42%) among these BDP nanoparticles, and indicated the best tumor treatment effect in vitro and in vivo. This work provides a systematic strategy for exploiting efficient photothermal agents.
Introduction
Phototherapy including photothermal therapy (PTT) and photodynamic therapy (PDT) has received wide attention in tumor treatment due to its non-invasiveness, slight side effects, and weak drug resistance.1–7 PDT usually induces apoptosis or necrosis of cancer cells by producing singlet oxygen (1O2) in the presence of oxygen or free radicals via photochemical reactions in cells.8–12 Therefore, PDT is susceptible to the tumor microenvironment (TME).13–19 In contrast, PTT relies on photothermal agents (PTAs) to cause local overheating under irradiation to kill tumor cells, which is less dependent on the TME.20–23 Recently, great progress has been made in the combination of PTT with other therapies.24–27 In addition, infrared thermal imaging could be used to monitor temperature and prevent damage to normal tissues caused by overtreatment during the process of PTT.28
Organic nanomaterials have attracted widespread attention due to their good biocompatibility and potential biodegradability.29 For the organic fluorophore, electron transitions in the excited states mainly in three ways: radiative transitions, non-radiative transitions, and intersystem crossing (ISC).30–32 To improve the photothermal conversion efficiency (PCE) of PTAs, process of non-radiative transitions must be increased.33–35 Liu and Pu et al. improved the PCE of dye molecules by preparing conjugated polymers nanoparticles.36–42 Peng and Yan et al. adjusted the assembly methods to increase the PCE of dyes.43,44 Although advances have been made in developing effective PTAs, there is a strong desire for a simple and effective method to maximize the photothermal activity of PTAs.45
Relative to porphyrin and cyanine, boron dipyrromethene (BDP) possesses various structures, high molar absorbance coefficient and robust photostability.46–50 It is straightforward to extend the absorption wavelength and improve the phototherapy performance of BDP derivatives by conjugating a class of phenylethylene groups at 1, 3, 5 and/or 7 positions of the BDP backbones (Scheme 1a).51,52 Relatively, it is easier to introduce groups at 3 and 5 positions due to the less steric effect.53–55
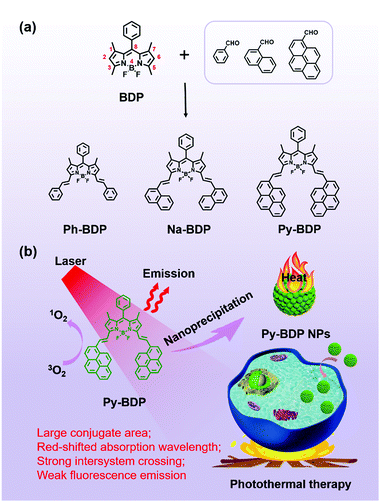 |
| Scheme 1 (a) The structure of BDP backbone and the structures of Ph-BDP, Na-BDP and Py-BDP. (b) Schematic illustration of the properties of Py-BDP and Py-BDP NPs, as well as the photothermal effects of Py-BDP NPs toward cancer cells. | |
Herein, to study the effect of conjugate areas on the photoproperties of BDP, phenyl, naphthyl and pyrenyl were introduced to BDP and form Ph-BDP, Na-BDP and Py-BDP, respectively (Scheme 1a). Compared to Ph-BDP and Na-BDP, Py-BDP generated the maximum singlet oxygen upon irradiation at the same condition. And nanoparticle (NP) formulations could effectively suppress emission and ISC of BDP molecules, and maximize the non-radiative relaxation (Scheme 1b). The Py-BDP NPs showed a high PCE and excellent tumor suppressing effect.
Results and discussion
Synthesis and characterization
To synthesize BDP molecules with different conjugate areas, phenyl, naphthyl and pyrenyl were introduced at 3 and 5 positions of BDP (Scheme 1a), and the obtained molecules were named Ph-BDP, Na-BDP and Py-BDP respectively (Scheme 1b). Their chemical structures and molecular weights were proved by 1H nuclear magnetic resonance (NMR) spectra and MALDI-TOF mass spectrometry (MS) analysis (Fig. S1–S3, ESI†). The absorption and emission spectra of three BDP molecules at the same molar concentration are shown in Fig. 1. The absorption maxima of Ph-BDP, Na-BDP and Py-BDP are at 623, 638 and 692 nm with molar extinction coefficients of 1.074 × 105, 8.24 × 104 and 9.12 × 104 M−1 cm−1, respectively. The emission maxima of three BDP molecules are at 637, 664 and 725 nm, respectively. The red-shifted absorption and emission peaks are expected after increasing the conjugate areas,56 which are further explained by the density functional theory (DFT) calculation.
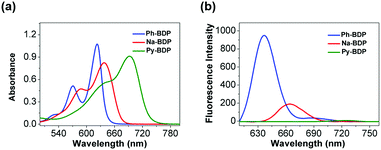 |
| Fig. 1 The (a) absorption and (b) emission spectra of Ph-BDP, Na-BDP and Py-BDP in N,N-dimethylformamide (10 μM). The excitation wavelengths were 620, 640 and 700 nm. | |
The gap of highest occupied molecular orbital (HOMO)–lowest unoccupied molecular orbital (LUMO) (HLG) determines the optical and electronic properties of organic molecules.57 Electron distributions of Ph-BDP, Na-BDP and Py-BDP are shown in Fig. 2a, increasing the conjugation degree of organic molecules could reduce HLG to some extent. The calculated energy gaps of three BDP molecules were 2.24, 2.19 and 1.99 eV, respectively. Among these three BDPs, Py-BDP showed the maximum redshifts in absorption and emission spectra, and the electrons were more easily excited. As shown in Fig. 2b, the energy of the excited states (Sn and Tn) gradually decreased and moved to the lowest excited states (S1 and T1) with the increase of conjugate areas. The smaller differences between different energy levels are more favorable for the ISC process to promote the generation of 1O2,58 that is, Py-BDP generates 1O2 more easily. When 1,3-diphenylisobenzofuran (DPBF) was used to detect the abilities of generating 1O2, it was found that Py-BDP reduced the absorbance of DPBF more significant than Ph-BDP and Na-BDP under the same conditions (Fig. 2c). This result is consistent with the theoretical calculations.
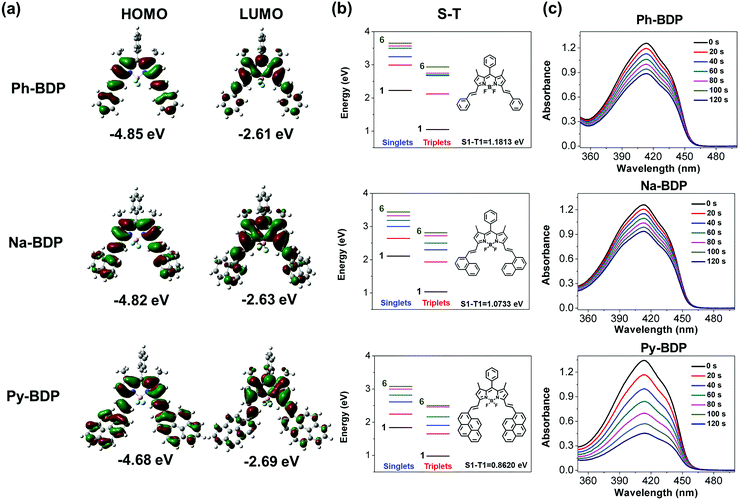 |
| Fig. 2 (a) HOMO and LUMO of the geometry structures and (b) excited singlet and triplet energy levels of Ph-BDP, Na-BDP and Py-BDP. Optimized with Gaussian 09/B3LYP/6-31G*. (c) Absorption spectra of DPBF in the presence of three BDP molecules under red LED light (27 mW cm−2) irradiation for different time. | |
Preparation and characterization of BDP NPs
Subsequently, BDP molecules could form NPs through nanoprecipitation method in the absence of surfactants, and they were named Ph-BDP NPs, Na-BDP NPs and Py-BDP NPs, respectively. The absorption maxima of three BDP NPs are at 643, 661 and 728 nm (Fig. 3a). Moreover, NPs show broadened absorption spectra with redshifts of about 20 nm as compared to dissolved BDP molecules because of the aggregation effect. At the same time, the fluorescence of NPs is poor because of the aggregation-caused quenching (ACQ) (Fig. S4, ESI†), which is shown under illumination of ultraviolet (UV) lamp (Fig. 3b). Moreover, three BDP NPs showed similar zeta potential of about −8 eV (Fig. 3c), and regular spherical shapes with sizes of about 200 nm as evidenced by transmission electron microscopy (TEM) images and dynamic light scattering (DLS) measurements (Fig. 3d–f). In addition, no obvious size changes were observed for BDP NPs in different conditions (Fig. S5, ESI†), indicating that NPs had excellent colloidal stability. These data demonstrate that there is no significant difference in the morphology, particle size, zeta potential and stability of three BDP NPs.
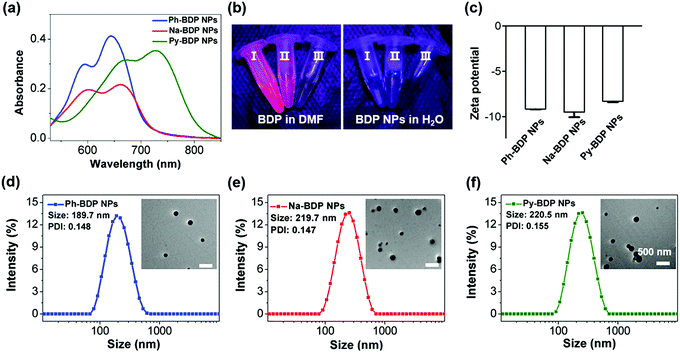 |
| Fig. 3 (a) The absorption spectra of Ph-BDP NPs, Na-BDP NPs and Py-BDP NPs in water. (b) Photograph of BDP and BDP NPs (I: Ph-BDP, II: Na-BDP, III: Py-BDP) under the UV lamp. (c) Zeta potential of different BDP NPs. The results are represented as mean ± SD (n = 3). Error bars represent standard deviations of three independent experiments. Size distribution of (d) Ph-BDP NPs, (e) Na-BDP NPs and (f) Py-BDP NPs measured by DLS and TEM images (inset). | |
Photothermal effects
9,10-Anthracenediyl-bi(methylene)-dimalonic acid (ABDA) was used to detect the ability of three BDP NPs to produce 1O2 (Fig. S6, ESI†). As expected, the ISC of BDP was quenched after the NPs were formed. The low quantum yield of 1O2 and fluorescence intensity is conducive to the photothermal effect.19 Subsequently, the photothermal activity was investigated. Under the same illumination conditions (685 nm laser, 0.55 W cm−2 for 5 min), the temperature of Ph-BDP NPs (25 μM), Na-BDP NPs (25 μM) and Py-BDP NPs (10 μM) were raised 26.7, 26.9 and 30.2 °C respectively (Fig. 4a–c). The PCE (η) of Ph-BDP NPs, Na-BDP NPs and Py-BDP NPs were 22%, 28% and 42%, respectively (Fig. S7, ESI†). The PCE of Py-BDP NPs is higher than some of the reported organic agents.32,42 Further research showed that the photothermal capability of Py-BDP NPs was concentration and power-intensity dependent (Fig. 4d and e). During the five cycles of heating and cooling, the increased temperature basically did not change, indicating that the Py-BDP NPs had high irradiation stability (Fig. 4f).
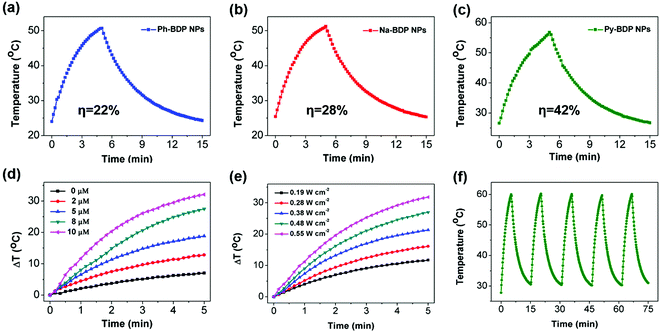 |
| Fig. 4 (a–c) Photothermal effect of three BDP NPs under irradiation of a 685 nm laser (0.55 W cm−2), and the laser was turned off after irradiation for 5 min. (d) Photothermal effect of Py-BDP NPs at various concentrations under irradiation of a 685 nm laser (0.55 W cm−2). (e) Photothermal effect of Py-BDP NPs (10 μM) with various laser power densities. (f) Photothermal effect of Py-BDP NPs upon five cycles of heating and cooling. | |
Cell uptake and cytotoxicity
To illustrate the cellular uptake of three BDP NPs, we assessed their internalization by human cervical carcinoma (HeLa) cells. It was observed by confocal laser scanning microscope (CLSM) that three BDP NPs could be efficiently internalized by cells (Fig. S8, ESI†). Subsequently, in vitro biocompatibility and phototoxicity of three BDP NPs were tested by using 3-(4,5-dimethylthiazol-2-yl)-2,5-diphenyltetrazolium bromide (MTT) assays. Three BDP NPs displayed a concentration-dependent cytotoxicity under laser irradiation (Fig. 5a–c). And the cell activity of cells in the absence of irradiation was all above 90%, indicating the good cell compatibility, of BDP NPs. It is worth noting that Py-BDP NPs could inhibit cell growth at a lower concentration (10 μM) than other two NPs (25 μM) due to the higher PCE. Furthermore, the experimental results in mouse breast carcinoma (4T1) cells (Fig. 5d–f) are similar to those in HeLa cells. In practical applications, it is not advisable to use large doses of drugs to achieve tumor ablation, thus Py-BDP NPs are more likely to meet the requirements.
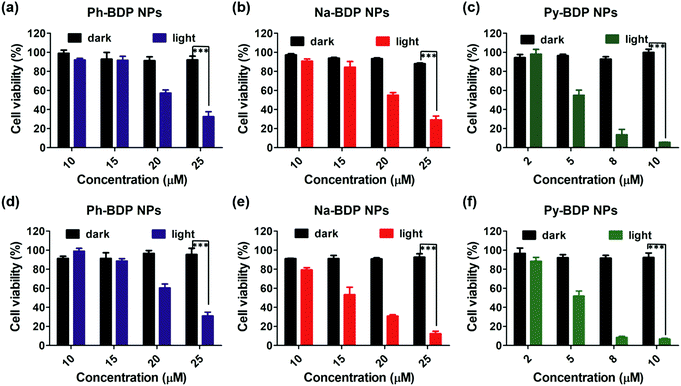 |
| Fig. 5 Cell viability of three BDP NPs against (a–c) HeLa and (d–f) 4T1 cells without (dark) or with (light) 685 nm laser irradiation (0.55 W cm−2, 5 min). The results are represented as mean ± SD (n = 3). Error bars represent standard deviations of three independent experiments. ***p < 0.001. | |
PTT effects of NPs
On the basis of above results, the PTT effects of three BDP NPs in vivo were studied. Mouse cervical carcinoma (U14) cells were subcutaneously injected into the legs of Kunming mice. After 6 days, the tumor-bearing mice were randomly divided into four groups with different treatments: PBS + light irradiation (L), Ph-BDP NPs + L, Na-BDP NPs + L and Py-BDP NPs + L. After intratumoral injection of NPs for 1 h, the tumor sites were treated with 685 nm laser (0.55 W cm−2, 10 min). In the process of illumination, the temperatures of tumor sites were monitored by near infrared thermal camera. The temperature at tumors in Py-BDP NPs + L increased to about 54 °C after illumination for 10 min (Fig. 6a and b). However, there was little difference in temperature at tumor of mice treated by Ph-BDP NPs + L and Na-BDP NPs + L, probably due to the similar PCEs of these two NPs. The tumor volumes of mice were recorded every other day. In Fig. 6c, the tumor volumes of mice treated by Ph-BDP NPs + L, Na-BDP NPs + L and Py-BDP NPs + L were smaller than those by PBS + L, demonstrating the effective inhibition of tumor growth by BDP NPs under laser irradiation. And the growth of tumors in the Py-BDP NPs + L was the most dramatically inhibited, which was also evidenced by the weights and picture of isolated tumors (Fig. 6d and e). In the process of treatment, the body weights of mice in each group displayed a rising trend (Fig. 6f). The above experimental results further verify that Py-BDP NPs possess the best ability of PTT.
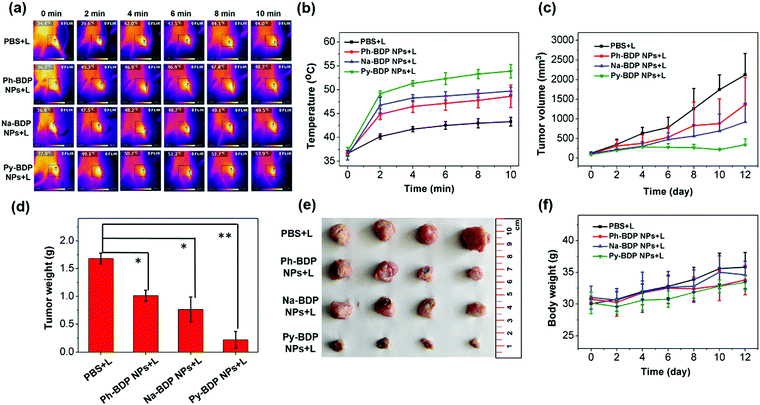 |
| Fig. 6 (a) Infrared thermal images of tumors injected with PBS or three BDP NPs, and then exposed to a 685 nm laser (0.55 W cm−2, 10 min). (b) Temperature changes of tumors in different groups. (c) Tumor volume changes of mice in different groups during treatment. (d) Tumor weights and (e) the tumor picture in the different groups. (f) Body weights of the mice during the therapy period. The results are represented as mean ± SD (n = 4). Error bars represent standard deviations of four independent experiments. *p < 0.05; **p < 0.01. | |
The antitumor effect of Py-BDP NPs was further investigated through the systemic administration. Py-BDP@F127 NPs were prepared by encapsulating Py-BDP into Pluronic F127 micelles (Fig. 7a). Since Py-BDP showed an emission maximum at 725 nm, the NIRF imaging performance of Py-BDP@F127 NPs was studied in living mice. After Py-BDP@F127 NPs were injected into tail veins of tumor bearing mice, the whole bodies of mice were imaged at different time. As shown in Fig. S9a (ESI†), the fluorescence intensity of tumor sites increased gradually, indicating that Py-BDP@F127 NPs could accumulate in tumors. Semiquantitative analysis of the fluorescence intensity of the tumor sites showed that the NPs reached a maximal accumulation in the tumors at 9 h post injection (Fig. S9b, ESI†).
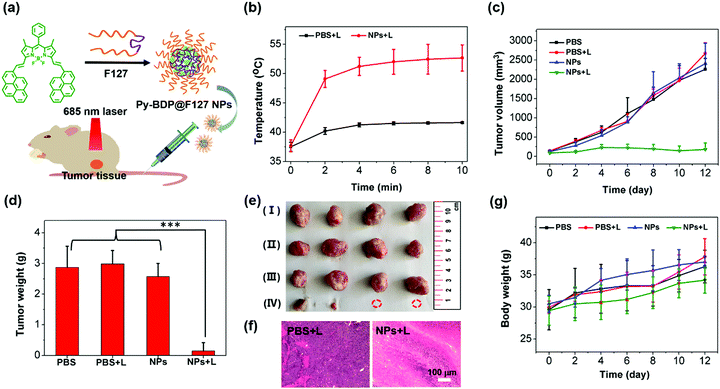 |
| Fig. 7 (a) Schematic illustration of the preparation of Py-BDP@F127 NPs and their applications in PTT of tumors in vivo. (b) Temperature changes of tumors in different groups. (c) Tumor volume changes of mice in each group during treatment. (d) Tumor weights of mice after various treatments. (e) Tumor images after different treatments. I: PBS, II: PBS + L, III: NPs, IV: NPs + L. (f) H&E staining of tumor tissues. (g) Body weights of the mice during the therapy period. The results are represented as mean ± SD (n = 4). Error bars represent standard deviations of four independent experiments. ***p < 0.001. | |
The therapeutic effect of Py-BDP@F127 NPs was studied after intravenous injection into U14 tumor bearing Kunming mice. The mice were randomly divided into four groups, named PBS, PBS + L, Py-BDP@F127 NPs and Py-BDP@F127 NPs + L respectively. The mice in PBS + L and Py-BDP@F127 NPs + L were intravenously injected with PBS and Py-BDP@F127 NPs, and after 9 h, the tumor sites were irradiated with 685 nm laser (0.4 W cm−2, 10 min). The temperature changes of tumors monitored by infrared thermal camera clearly showed that the temperature of tumors in NPs + L was significantly higher than that in PBS + L (Fig. S10, ESI†). The tumor growth of mice in PBS, PBS + L and NPs could not be inhibited, and the tumor volumes increased with an average of 18–20 times (Fig. 7c). However, the tumor growth in Py-BDP@F127 NPs + L was markedly inhibited, which was further confirmed by the weights (Fig. 7d) and picture of isolated tumors (Fig. 7e) after treatment. From hematoxylin and eosin (H&E) staining images of tumor regions (Fig. 7f), severe damage to tumor cells could be detected in NPs + L, while no distinct cell destruction could be observed for the PBS + L. These results prove that the photothermal properties of Py-BDP@F127 NPs could effectively inhibit the tumor growth.
Moreover, Py-BDP@F127 NPs also showed superior physiological safety in vivo. In the process of treatment, the body weights of mice in each group displayed a rising trend, showing that the systematic toxicity of all treatment methods was very low (Fig. 7g). After the treatment, H&E staining was performed on isolated organs (heart, liver, spleen, lung and kidney) of mice.59,60 As shown in Fig. S11 (ESI†), no obvious damage was found in different organs. This further indicated that Py-BDP@F127 NPs had good biocompatibility at current dose.
Conclusions
In summary, three BDP fluorophores (Ph-BDP, Na-BDP and Py-BDP) with different conjugate areas were synthesized. The results of DFT simulation show that the energy gap decreases with the increase of conjugate area, and Py-BDP with the largest conjugate area possesses better phototherapy performance. The formed BDP NPs could prevent the production of ROS and quench the fluorescence of BDP molecules, thus improving the photothermal activity. Finally, the photothermal property of Py-BDP NPs was validated by the potent phototoxicity and effective tumor treatment. This work highlights the potential of precise design of photoactive organic nanomaterials with tunable photothermal activity.
Conflicts of interest
There are no conflicts to declare.
Acknowledgements
The work was supported by the National Natural Science Foundation of China (project no. 51973214).
References
- Q. Tang, W. Xiao, C. Huang, W. Si, J. Shao, W. Huang, P. Chen, Q. Zhang and X. Dong, pH-triggered and enhanced simultaneous photodynamic and photothermal therapy guided by photoacoustic and photothermal imaging, Chem. Mater., 2017, 29, 5216–5224 CrossRef CAS.
- J. Zou, L. Xue, N. Yang, Y. Ren, Z. Fan, W. Wang, W. Si, Y. Zhang, W. Huang and X. Dong, A glutathione responsive pyrrolopyrrolidone nanotheranostic agent for turn-on fluorescence imaging guided photothermal/photodynamic cancer therapy, Mater. Chem. Front., 2019, 3, 2143–2150 RSC.
- X. Zhao, S. Long, M. Li, J. Cao, Y. Li, L. Guo, W. Sun, J. Du, J. Fan and X. Peng, Oxygen-dependent regulation of excited-state deactivation process of rational photosensitizer for smart phototherapy, J. Am. Chem. Soc., 2020, 142, 1510–1517 CrossRef CAS.
- T. Sun, X. Chen, X. Wang, S. Liu, J. Liu and Z. Xie, Enhanced efficacy of photothermal therapy by combining a semiconducting polymer with an inhibitor of a heat shock protein, Mater. Chem. Front., 2019, 3, 127–136 RSC.
- W. Lin, W. Zhang, S. Liu, Z. Li, X. Hu, Z. Xie, C. Duan and G. Han, Engineering pH-responsive BODIPY nanoparticles for tumor selective multimodal imaging and phototherapy, ACS Appl. Mater. Interfaces, 2019, 11, 43928–43935 CrossRef CAS.
- C. Zhang, X. Cheng, M. Chen, J. Sheng, J. Ren, Z. Jiang, J. Cai and Y. Hu, Fluorescence guided photothermal/photodynamic ablation of tumours using pH-responsive chlorin e6-conjugated gold nanorods, Colloids Surf., B, 2017, 160, 345–354 CrossRef CAS.
- C. Zhang, W. Chen, T. Zhang, X. Jiang and Y. Hu, Hybrid nanoparticle composites applied to photodynamic therapy: strategies and applications, J. Mater. Chem. B, 2020, 8, 4726–4737 RSC.
- C. Zhao, Y. Li, L. Shao, X. Wang, J. Lu, X. Li, L. Chen, X. Cui, W. Sheng, X. Deng and Y. Wu, Reactive oxygen species-responsive theranostic nanoparticles for enhanced hypoxic tumor photodynamic therapy via synchronous HIF-1α inhibition and ATP depletion, Mater. Chem. Front., 2019, 3, 1793–1799 RSC.
- R. Tian, W. Sun, M. Li, S. Long, M. Li, J. Fan, L. Guo and X. Peng, Development of a novel anti-tumor theranostic platform: a near-infrared molecular upconversion sensitizer for deep-seated cancer photodynamic therapy, Chem. Sci., 2019, 10, 10106–10112 RSC.
- Q. Wang, B. Xia, J. Xu, X. Niu, J. Cai, Q. Shen, W. Wang, W. Huang and Q. Fan, Biocompatible small organic molecule phototheranostics for NIR-II fluorescence/photoacoustic imaging and simultaneous photodynamic/photothermal combination therapy, Mater. Chem. Front., 2019, 3, 650–655 RSC.
- Y. Jiang, J. Li, Z. Zeng, C. Xie, Y. Lyu and K. Pu, Organic photodynamic nanoinhibitor for synergistic cancer therapy, Angew. Chem., Int. Ed., 2019, 58, 8161–8165 CrossRef CAS.
- C. Zhang, J. Ren, J. Hua, L. Xia, J. He, D. Huo and Y. Hu, Multifunctional Bi2WO6 nanoparticles for CT-guided photothermal and oxygen-free photodynamic therapy, ACS Appl. Mater. Interfaces, 2018, 10, 1132–1146 CrossRef CAS.
- D. Gao, B. Zhang, Y. Liu, D. Hu, Z. Sheng, X. Zhang and Z. Yuan, Molecular engineering of near-infrared light-responsive BODIPY-based nanoparticles with enhanced photothermal and photoacoustic efficiencies for cancer theranostics, Theranostics, 2019, 9, 5315–5331 CrossRef CAS.
- C. Li, W. Zhang, S. Liu, X. Hu and Z. Xie, Mitochondria-targeting organic nanoparticles for enhanced photodynamic/photothermal therapy, ACS Appl. Mater. Interfaces, 2020, 12, 30077–30084 CrossRef CAS.
- Z. Guo, Y. Zou, H. He, J. Rao, S. Ji, X. Cui, H. Ke, Y. Deng, H. Yang, C. Chen, Y. Zhao and H. Chen, Bifunctional platinated nanoparticles for photoinduced tumor ablation, Adv. Mater., 2016, 28, 10155–10164 CrossRef CAS.
- H. Zuo, J. Tao, H. Shi, J. He, Z. Zhou and C. Zhang, Platelet-mimicking nanoparticles co-loaded with W18O49 and metformin alleviate tumor hypoxia for enhanced photodynamic therapy and photothermal therapy, Acta Biomater., 2018, 80, 296–307 CrossRef CAS.
- H. Huang, C. Zhang, X. Wang, J. Shao, C. Chen, H. Li, C. Ju, J. He, H. Gu and D. Xia, Overcoming hypoxia-restrained radiotherapy using an erythrocyte-inspired and glucose-activatable platform, Nano Lett., 2020, 20, 4211–4219 CrossRef CAS.
- P. Zhao, S. Ren, Y. Liu, W. Huang, C. Zhang and J. He, PL-W18O49-TPZ nanoparticles for simultaneous hypoxia-activated chemotherapy and photothermal therapy, ACS Appl. Mater. Interfaces, 2018, 10, 3405–3413 CrossRef CAS.
- C. Zhang, D. Xia, J. Liu, D. Huo, X. Jiang and Y. Hu, Bypassing the immunosuppression of myeloid-derived suppressor cells by reversing tumor hypoxia using a platelet-inspired platform, Adv. Funct. Mater., 2020, 30, 2000189 CrossRef CAS.
- C. Ou, Y. Zhang, D. Pan, K. Ding, S. Zhang, W. Xu, W. Wang, W. Si, Z. Yang and X. Dong, Zinc porphyrin-polydopamine core-shell nanostructures for enhanced photodynamic/photothermal cancer therapy, Mater. Chem. Front., 2019, 3, 1786–1792 RSC.
- C. Wang, R. Xue, A. Gulzar, Y. Kuang, H. Shao, S. Gai, D. Yang, F. He and P. Yang, Targeted and imaging-guided chemo-photothermal ablation achieved by combining upconversion nanoparticles and protein-capped gold nanodots, Chem. Eng. J., 2019, 370, 1239–1250 CrossRef CAS.
- J. Dai, Y. Luo, D. Nie, J. Jin, S. Yang, G. Li, Y. Yang and W. Zhang, pH/photothermal dual-responsive drug delivery and synergistic chemo-photothermal therapy by novel porous carbon nanofibers, Chem. Eng. J., 2020, 397, 125402 CrossRef CAS.
- T. Sun, J. Han, S. Liu, X. Wang, Z. Y. Wang and Z. Xie, Tailor-made semiconducting polymers for second near-infrared photothermal therapy of orthotopic liver cancer, ACS Nano, 2019, 13, 7345–7354 CrossRef CAS.
- Y. Zhang, C. Xu, X. Yang and K. Pu, Photoactivatable protherapeutic nanomedicine for cancer, Adv. Mater., 2020, 32, e2002661 CrossRef.
- J. Li, D. Cui, J. Huang, S. He, Z. Yang, Y. Zhang, Y. Luo and K. Pu, Organic semiconducting pro-nanostimulants for near-infrared photoactivatable cancer immunotherapy, Angew. Chem., Int. Ed., 2019, 58, 12680–12687 CrossRef CAS.
- G. Gao, Y. W. Jiang, Y. Guo, H. R. Jia, X. Cheng, Y. Deng, X. W. Yu, Y. X. Zhu, H. Y. Guo, W. Sun, X. Liu, J. Zhao, S. Yang, Z. W. Yu, F. M. S. Raya, G. Liang and F. G. Wu, Enzyme-mediated tumor starvation and phototherapy enhance mild-temperature photothermal therapy, Adv. Funct. Mater., 2020, 30, 1909391 CrossRef CAS.
- Y. Jiang, X. Zhao, J. Huang, J. Li, P. K. Upputuri, H. Sun, X. Han, M. Pramanik, Y. Miao, H. Duan, K. Pu and R. Zhang, Transformable hybrid semiconducting polymer nanozyme for second near-infrared photothermal ferrotherapy, Nat. Commun., 2020, 11, 1857 CrossRef CAS.
- X. Zhen, C. Xie and K. Pu, Temperature-correlated afterglow of a semiconducting polymer nanococktail for imaging-guided photothermal therapy, Angew. Chem., Int. Ed., 2018, 57, 3938–3942 CrossRef CAS.
- X. Li, L. Liu, S. Li, Y. Wan, J. X. Chen, S. Tian, Z. Huang, Y. F. Xiao, X. Cui, C. Xiang, Q. Tan, X. H. Zhang, W. Guo, X. J. Liang and C. S. Lee, Biodegradable π-conjugated oligomer nanoparticles with high photothermal conversion efficiency for cancer theranostics, ACS Nano, 2019, 13, 12901–12911 CrossRef CAS.
- D. Wang, M. M. S. Lee, W. Xu, G. Shan, X. Zheng, R. T. K. Kwok, J. W. Y. Lam, X. Hu and B. Z. Tang, Boosting non-radiative decay to do useful work: development of a multi-modality theranostic system from an AIEgen, Angew. Chem., Int. Ed., 2019, 58, 5628–5632 CrossRef CAS.
- L. Zhao, Y. Liu, R. Chang, R. Xing and X. Yan, Supramolecular photothermal nanomaterials as an emerging paradigm toward precision cancer therapy, Adv. Funct. Mater., 2019, 29, 1806877 CrossRef.
- G. Feng, G.-Q. Zhang and D. Ding, Design of superior phototheranostic agents guided by Jablonski diagrams, Chem. Soc. Rev., 2020 10.1039/d0cs00671h.
- W. Sun, X. Zhao, J. Fan, J. Du and X. Peng, Boron dipyrromethene nano-photosensitizers for anticancer phototherapies, Small, 2019, 15, e1804927 CrossRef.
- J. Li and K. Pu, Semiconducting polymer nanomaterials as near-infrared photoactivatable protherapeutics for cancer, Acc. Chem. Res., 2020, 53, 752–762 CrossRef CAS.
- Y. Xu, T. Feng, T. Yang, H. Wei, H. Yang, G. Li, M. Zhao, S. Liu, W. Huang and Q. Zhao, Utilizing Intramolecular Photoinduced Electron Transfer to Enhance Photothermal Tumor Treatment of Aza-BODIPY-Based Near-Infrared Nanoparticles, ACS Appl. Mater. Interfaces, 2018, 10, 16299–16307 CrossRef CAS.
- Y. Lyu, Y. Fang, Q. Miao, X. Zhen, D. Ding and K. Pu, Intraparticle molecular orbital engineering of semiconducting polymer nanoparticles as amplified theranostics for in vivo photoacoustic imaging and photothermal therapy, ACS Nano, 2016, 10, 4472–4481 CrossRef CAS.
- J. Li, C. Xie, J. Huang, Y. Jiang, Q. Miao and K. Pu, Semiconducting polymer nanoenzymes with photothermic activity for enhanced cancer therapy, Angew. Chem., Int. Ed., 2018, 57, 3995–3998 CrossRef CAS.
- T. Dong, K. Wen, J. Chen, J. Xie, W. Fan, H. Ma, L. Yang, X. Wu, F. Xu, A. Peng and H. Huang, Significant enhancement of photothermal and photoacoustic efficiencies for semiconducting polymer nanoparticles through simply molecular engineering, Adv. Funct. Mater., 2018, 28, 1800135 CrossRef.
- B. Guo, G. Feng, P. N. Manghnani, X. Cai, J. Liu, W. Wu, S. Xu, X. Cheng, C. Teh and B. Liu, A porphyrin-based conjugated polymer for highly efficient in vitro and in vivo photothermal therapy, Small, 2016, 12, 6243–6254 CrossRef CAS.
- B. Guo, Z. Sheng, D. Hu, A. Li, S. Xu, P. N. Manghnani, C. Liu, L. Guo, H. Zheng and B. Liu, Molecular engineering of conjugated polymers for biocompatible organic nanoparticles with highly efficient photoacoustic and photothermal performance in cancer theranostics, ACS Nano, 2017, 11, 10124–10134 CrossRef CAS.
- Y. Lyu, S. He, J. Li, Y. Jiang, H. Sun, Y. Miao and K. Pu, A photolabile semiconducting polymer nanotransducer for near-infrared regulation of CRISPR/Cas9 gene editing, Angew. Chem., Int. Ed., 2019, 58, 18197–18201 CrossRef CAS.
- J. Li and K. Pu, Development of organic semiconducting materials for deep-tissue optical imaging, phototherapy and photoactivation, Chem. Soc. Rev., 2019, 48, 38–71 RSC.
- D. Xi, M. Xiao, J. Cao, L. Zhao, N. Xu, S. Long, J. Fan, K. Shao, W. Sun, X. Yan and X. Peng, NIR light-driving barrier-free group rotation in nanoparticles with an 88.3% photothermal conversion efficiency for photothermal therapy, Adv. Mater., 2020, 32, e1907855 CrossRef.
- L. Zhao, Y. Liu, R. Xing and X. Yan, Supramolecular photothermal effects: a promising mechanism for efficient thermal conversion, Angew. Chem., Int. Ed., 2020, 59, 3793–3801 CrossRef CAS.
- H. S. Jung, P. Verwilst, A. Sharma, J. Shin, J. L. Sessler and J. S. Kim, Organic molecule-based photothermal agents: an expanding photothermal therapy universe, Chem. Soc. Rev., 2018, 47, 2280–2297 RSC.
- H. Wang, J. Chang, M. Shi, W. Pan, N. Li and B. Tang, A dual-targeted organic photothermal agent for enhanced photothermal therapy, Angew. Chem., Int. Ed., 2019, 58, 1057–1061 CrossRef CAS.
- V. R. Donuru, G. K. Vegesna, S. Velayudham, S. Green and H. Liu, Synthesis and optical properties of red and deep-red emissive polymeric and copolymeric BODIPY dyes, Chem. Mater., 2009, 21, 2130–2138 CrossRef CAS.
- X. Zheng, L. Wang, M. Liu, P. Lei, F. Liu and Z. Xie, Nanoscale mixed-component metal–organic frameworks with photosensitizer spatial-arrangement-dependent photochemistry for multimodal-imaging-guided photothermal therapy, Chem. Mater., 2018, 30, 6867–6876 CrossRef CAS.
- Y. Zou, M. Li, T. Xiong, X. Zhao, J. Du, J. Fan and X. Peng, A single molecule drug targeting photosensitizer for enhanced breast cancer photothermal therapy, Small, 2020, 16, e1907677 CrossRef.
- J. Shen, Q. Wang, Y. Lv, J. Dong, G. Xuan, J. Yang, D. Wu, J. Zhou, G. Yu, G. Tang, X. Li, F. Huang and X. Chen, Nanomedicine fabricated from a boron-dipyrromethene (BODIPY)-embedded amphiphilic copolymer for photothermal-enhanced chemotherapy, ACS Biomater. Sci. Eng., 2019, 5, 4463–4473 CrossRef CAS.
- W. Hu, X. Miao, H. Tao, A. Baev, C. Ren, Q. Fan, T. He, W. Huang and P. N. Prasad, Manipulating nonradiative decay channel by intermolecular charge transfer for exceptionally improved photothermal conversion, ACS Nano, 2019, 13, 12006–12014 CrossRef CAS.
- T. Zhang, C. Ma, T. Sun and Z. Xie, Unadulterated BODIPY nanoparticles for biomedical applications, Coord. Chem. Rev., 2019, 390, 76–85 CrossRef CAS.
- Y. Liu, C. Xu, L. Teng, H.-W. Liu, T.-B. Ren, S. Xu, X. Lou, H. Guo, L. Yuan and X.-B. Zhang, pH stimulus-disaggregated BODIPY: an activated photodynamic/photothermal sensitizer applicable to tumor ablation, Chem. Commun., 2020, 56, 1956–1959 RSC.
- H. Lu, J. Mack, Y. Yang and Z. Shen, Structural modification strategies for the rational design of red/NIR region BODIPYs, Chem. Soc. Rev., 2014, 43, 4778–4823 RSC.
- C. Li, W. Lin, S. Liu, W. Zhang and Z. Xie, Self-destructive PEG-BODIPY nanomaterials for photodynamic and photothermal therapy, J. Mater. Chem. B, 2019, 7, 4655–4660 RSC.
- W. Shao, Q. Wei, S. Wang, F. Li, J. Wu, J. Ren, F. Cao, H. Liao, J. Gao, M. Zhou and D. Ling, Molecular engineering of D-A-D conjugated small molecule nanoparticles for high performance NIR-II photothermal therapy, Mater. Horiz., 2020, 7, 1379–1386 RSC.
- G. Qian and Z. Y. Wang, Near-Infrared Organic Compounds and Emerging Applications, Chem. – Asian J., 2010, 5, 1006–1029 CrossRef CAS.
- S. Wang, W. Wu, P. Manghnani, S. Xu, Y. Wang, C. C. Goh, L. G. Ng and B. Liu, Polymerization-enhanced two-photon photosensitization for precise photodynamic therapy, ACS Nano, 2019, 13, 3095–3105 CrossRef CAS.
- C. Zhang, J. Ren, J. He, Y. Ding, D. Huo and Y. Hu, Long-term monitoring of tumor-related autophagy in vivo by Fe3O4–NO. nanoparticles, Biomaterials, 2018, 179, 186–198 CrossRef CAS.
- C. Zhang, J. Ren, Y. Yang, D. Wang, J. He, D. Huo and Y. Hu, Ultra-sensitive diagnosis of orthotopic patient derived hepatocellular carcinoma by Fe@graphene nanoparticles in MRI, RSC Adv., 2016, 6, 113919–113923 RSC.
Footnote |
† Electronic supplementary information (ESI) available: Materials, characterizations and experiments. See DOI: 10.1039/d0qm00624f |
|
This journal is © the Partner Organisations 2021 |
Click here to see how this site uses Cookies. View our privacy policy here.