DOI:
10.1039/D0QM00635A
(Research Article)
Mater. Chem. Front., 2021,
5, 375-385
Hierarchical MoP/NiFeP hybrid hollow spheres as highly efficient bifunctional electrocatalysts for overall water splitting†
Received
28th August 2020
, Accepted 11th October 2020
First published on 13th October 2020
Abstract
Although some progress on exploring non-noble-metal based materials with remarkable activity for the electrocatalytic hydrogen or oxygen evolution reactions has been made, it still remains a critical challenge especially for overall water splitting. Herein, we report a facile synthesis of novel hierarchical MoP/NiFeP hybrid hollow spheres (MoP/NiFeP HSs) as an electrocatalyst for the first time. The obtained MoP/NiFeP HSs enable not only abundant accessible sites, but also facilitated electron transfer through the heterointerfaces and synergistically promoted kinetics. The optimal MoP/NiFeP HSs present efficient bifunctional electrocatalytic performance with low overpotentials of 73 and 256 mV to achieve 10 mA cm−2 for the HER and OER in 1 M KOH, respectively. More importantly, when employed as electrocatalysts in a two-electrode device, low cell voltage of 1.51 V at 10 mA cm−2 with long term durability can be achieved, comparable to the Ir/C-Pt/C couple and exceeding those of the recently reported typical bifunctional electrocatalysts. This work might inspire the design and preparation of highly active and low-cost hybrid materials with hierarchical structures for water splitting applications.
Introduction
As the carrier of energy, H2 is now widely used in distributed generation and fuel cell vehicles, relieving the energy crisis.1–6 Currently, hydrogen is mostly produced by the transformation of fossil fuels under high temperature, which is low cost but has heavy pollution and low product purity.7,8
Electrochemical water splitting, which includes the hydrogen evolution reaction (HER) and oxygen evolution reaction (OER), is regarded as a potential way to settle the above issues. In order to reduce the power input and accelerate the reaction rate, catalysts such as platinum- and iridium-based materials should be loaded on the electrodes. Such high cost derived from noble-metal materials greatly restricts the large-scale popularization.9–14 Recently, much effort has been devoted to discovering low-cost efficient alternatives, such as Mo-based compounds for the HER and Ni-based compounds for the OER.15–20 To further boost the catalytic activity, various modulated strategies including elemental doping,21 phase modification22 and nanocarbon incorporation,23 such as Co doped Mo2C nanowires,24 NiCo2Px nanowires,25 NiFe-layered double hydroxide nanosheets,26etc., were conducted.
Nonetheless, these methods remain difficult to promote the HER and OER simultaneously. To work out this issue, well-designed HER and OER electrocatalysts in a single heterostructure would be more appealing for overall water splitting with high efficiency,27–32 because of abundant interfaces formed by different components that can exhibit electron redistribution and synergistically promoted kinetics for water splitting.33–37 For example, MoP, highly active for the HER, presents insufficient activity for overall water splitting on account of insufficient OER activity.38–40 Its OER and overall water splitting performance were enhanced by combining with CoP or Ni2P.41,42 Incorporation of Fe into Ni-based compounds could dramatically improve the OER performance owing to the stronger affinity to reaction intermediates.43–46 In addition, hierarchical hollow structures exhibit enhanced electrocatalytic performance owing to the massive surface area with accessible active sites and short diffusion paths for electrons and ions.47–53 Accordingly, constructing Mo–NiFe–P heterointerfaces with a hierarchical hollow structure is a great innovation for enhanced HER, OER and the corresponding overall water splitting. Nevertheless, it is very arduous to design and fabricate Mo–NiFe–P hierarchical hollow structures because of the multi-step process and the compatibility of elements, which thus has never been reported.
Herein, a novel one-step method to synthesize new hierarchical MoP/NiFeP hybrid hollow spheres (MoP/NiFeP HSs) as bifunctional electrocatalysts is reported for the first time. Profiting from strong interfacial coupling effects between MoP and NiFeP, the greatly conductive support of graphitic carbon and the hierarchical hollow structure, the optimal MoP/NiFeP HSs present efficient catalytic performance in 1 M KOH with low overpotentials of 73 and 256 mV to achieve 10 mA cm−2 for the HER and OER, respectively. More importantly, when employed as electrocatalysts in a two-electrode system, only 1.51 V is needed to achieve 10 mA cm−2, exceeding those of the recently reported typical bifunctional electrocatalysts.
Experimental section
Materials
(NH4)6Mo7O24·4H2O, dopamine hydrochloride, Ni(NO3)2·6H2O, K3[Fe(CN)6], ammonium hydroxide (25–28 wt%), ethanol, NaH2PO2·H2O, carbon paper and Nafion solution (5 wt%) were bought from Sinopharm Chemical Reagent Co., Ltd. Commercial 20 wt% Pt/C and Ir/C electrocatalysts were bought from Premetek Co.
Synthesis of hierarchical MoP/NiFeP hybrid hollow spheres
Typically, 37 mg of (NH4)6Mo7O24·4H2O, 37 mg of dopamine hydrochloride and 1 mg of K3[Fe(CN)6] were dissolved into water (8 mL) to obtain a transparent solution. Then, 16 mL of ethanol was added quickly into the above solution under stirring. After being stirred for 30 min, 100 μL of ammonium hydroxide was added to initiate the polymerization, followed by dropwise addition of a solution of Ni(NO3)2 (1 mg Ni(NO3)2·6H2O dissolved into 2 mL of water). After being stirred for 2 h, Mo-PDA/NiFe-PBA-1 hollow spheres were separated by centrifugation, washed several times with ethanol and dried at 40 °C in an oven. The obtained black powder was then placed in a tube furnace with 1 g of NaH2PO2·H2O upstream and annealed at 300 °C for 2 h with a ramp of 2 °C min−1 under an argon atmosphere and further at 800 °C for 1 h at a ramp of 5 °C min−1 to obtain MoP/NiFeP-1 HSs after being cooled to ambient temperature. MoP/NiFeP-2 HSs were synthesized using the same procedure as MoP/NiFeP-1 HSs, except for different addition amounts of K3[Fe(CN)6] (4 mg) and Ni(NO3)2·6H2O (4 mg).
Characterization
Transmission electron microscopy (JEOL, JEM-2100F and FEI, Tecnai G2 20 TWIN) was applied to observe the hollow structure and nanoparticles at 200 kV. Scanning electron microscopy (Zeiss, Ultra 55) was applied to characterize the surface texture. X-ray diffraction (Bruker, D8 Advance, Cu Kα radiation λ = 1.5406 Å) was applied to record the crystallographic information. To confirm the actual Mo, Ni and Fe contents of the catalysts, inductively coupled plasma-optical emission spectroscopy (Thermo Scientific, iCAP 7400) was applied. X-ray photoelectron spectroscopy (ULVAC-PHI, PHI 5000C & PHI 5300) was used to characterize the surface composition. A Micromeritics ASAP 2020 system was applied to determine the specific surface area. RM 2000 was applied to acquire Raman spectra with a laser of 532 nm.
Electrochemical measurements
A CHI 760E electrochemistry workstation was applied to perform all electrochemical measurements at ambient temperature with a three-electrode device in 1 M KOH (pH 14). A glassy carbon electrode (GCE) (diameter of 5 mm) was used as the working electrode, with a graphite rod and saturated calomel electrode (SCE, in saturated KCl solution) as a counter and reference electrode, respectively. To prepare a homogeneous catalyst ink, 3 mg of catalyst was dispersed in 880 μL of ethanol and 120 μL of Nafion solution (5 wt%) with at least 30 min ultrasonication. By using a pipette, the catalyst ink was drop cast on the GCE. The HER and OER performances were evaluated in Ar- and O2-saturated solution, respectively. E (vs. RHE) = E (vs. SCE) + (0.242 + 0.059 pH) V. IR compensation with a level of 90% was applied before all the linear sweep voltammetry (LSV) tests. The polarization curves were acquired by LSV with a scan rate of 5 mV s−1. A frequency range from 0.1 to 100 kHz was determined to employ electrochemical impedance spectroscopy (EIS) measurements.
Pre-treated carbon paper with catalyst ink drop-cast was applied in a two-electrode device to perform overall water splitting tests in 1 M KOH. A noble metal-based catalyst, Ir/C/CP(+)||Pt/C/CP(−) was used as the benchmark.
Results and discussion
Fig. 1 elucidates the schematic process of synthesizing MoP and MoP/NiFeP HSs via a facile and effective strategy. Under the catalysis of ammonium hydroxide, dopamine molecules would self-polymerize and chelate with the Mo7O246− anions in water to form Mo–polydopamine (Mo–PDA) composites.54,55 After the addition of ethanol, the water-soluble Mo–PDA would aggregate at the interface of ethanol/water to form hierarchical hollow spheres assembled by the nanosheets, which were denoted as Mo–PDA HSs (Fig. S1a and d, ESI†). If a small amount of [Fe(CN)6]3− were further introduced into the reaction mixture, [Fe(CN)6]3− anions would be adsorbed and chelated in the Mo–PDA composites. Subsequently adding an equal number of Ni2+ cations during the formation of hierarchical hollow spheres, the NiFe-Prussian-blue analog nanoparticles (NiFe-PBA NPs) would precipitate in situ and be wrapped inside the Mo–PDA nanosheets to directly produce Mo-PDA/NiFe-PBA HSs via only one-step. Generally, converting different precursors into an individual nanostructure usually involves a multi-step process and is restricted by the compatibility of the components or elements,56,57 but our one-step strategy only needs one-step coprecipitation with good integration of the metal precursors, revealing simplicity and superiority. Compared with the original Mo-PDA HSs, the Mo-PDA/NiFe-PBA-1 HSs from 1 mg of K3[Fe(CN)6] and Ni(NO3)2·6H2O reveal no significant morphological differences (Fig. S1b and e, ESi1). However, the loading capacity of Mo-PDA is limited. An increasing number of [Fe(CN)6]3− and Ni2+ cannot be completely wrapped inside the nanosheets. Instead, the excess precipitated NiFe-PBA NPs would adhere to the exterior of the hollow spheres via surface adsorption, as shown in Fig. S1c and f (ESI†) for Mo-PDA/NiFe-PBA-2 HSs from 4 mg of K3[Fe(CN)6] and Ni(NO3)2·6H2O.
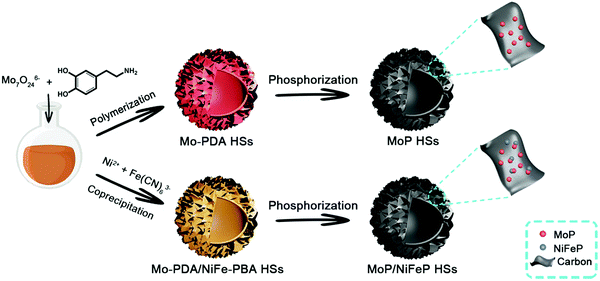 |
| Fig. 1 Diagram for the synthesis of hierarchical MoP and MoP/NiFeP hollow spheres. | |
After high-temperature phosphorization, hollow structure still remains, and the metal precursors are converted into highly crystalline metal phosphide nanoparticles, coated by the carbon nanosheets (Fig. 2). Compared to MoP HSs and MoP/NiFeP-1 HSs, the MoP/NiFeP-2 HSs are larger and severely agglomerated owing to the excess introduction of NiFe-PBA NPs. To verify this, NiFe-PBA NPs were also prepared by one-step coprecipitation. Without the encapsulation of carbon, nanoparticles tend to integrate together to form bigger size during calcination (Fig. S2, ESI†). Scanning electron microscopy (SEM) images indicated that the hierarchical structures of MoP HSs and MoP/NiFeP-1 HSs were well-defined (Fig. S3, ESI†) while the external surfaces of MoP/NiFeP-2 HSs were relatively smooth, and the boundaries of the hollow spheres were not clearly distinguished. The specific surface area of the MoP HSs was 425.1 m2 g−1, larger than those of MoP/NiFeP-1 HSs (411.5 m2 g−1) and MoP/NiFeP-2 HSs (355.8 m2 g−1), further indicating that more NiFeP NPs not only harm the hierarchical structure, but also decrease the surface area (Fig. S4, ESI†).
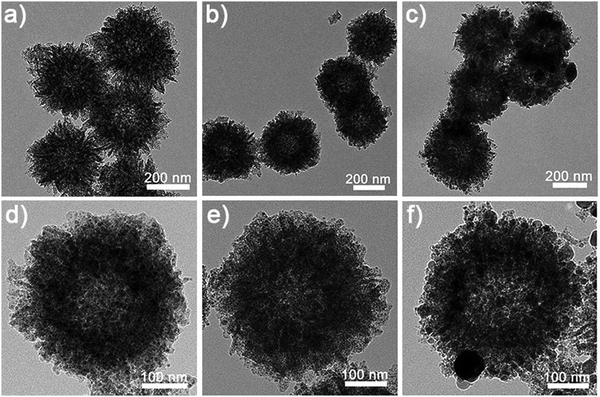 |
| Fig. 2 TEM images of (a and d) MoP HSs, (b and e) MoP/NiFeP-1 HSs, and (c and f) MoP/NiFeP-2 HSs. | |
The X-ray diffraction (XRD) patterns of MoP and MoP/NiFeP HSs exhibit several diffraction peaks at 32.0, 43.0, and 57.1° corresponding to the (100), (101), and (110) planes of MoP (JCPDS No. 65-6487) (Fig. 3a).58,59 After the introduction of NiFeP, no peak shift is observed, suggesting that Ni and Fe have not been incorporated into the crystal structure of MoP. Although the characteristic diffraction peaks of NiFeP cannot be observed for MoP/NiFeP-1 HSs owing to too low amount of NiFeP, the MoP/NiFeP-2 HSs present a small peak around 40° corresponding to NiFeP (40.7°, Fig. S5, ESI†),60,61 confirming the existence of NiFeP. Raman spectra display typical peaks at about 1350 and 1590 cm−1 for all the HS samples (Fig. 3b), in accordance with D (defect) and G (graphitic) bands of graphitized carbon, revealing the existence of graphitic carbon.62–64 The corrosion resistance of the nanoparticles can be improved by covering with graphitized carbon layers. Also, electron transfer can be accelerated to boost the electrocatalytic efficiency.65,66
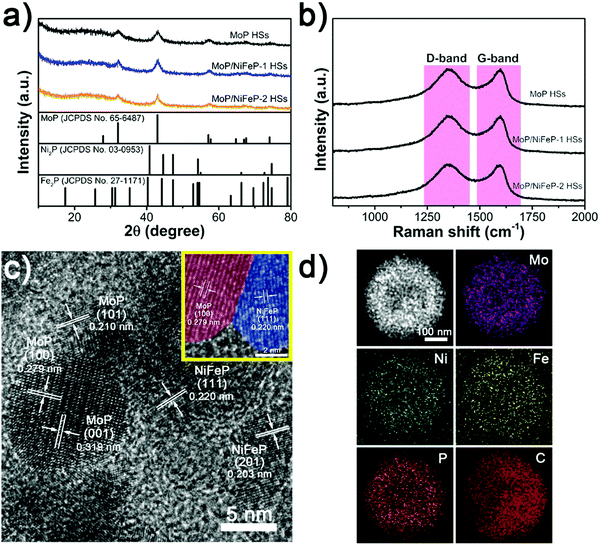 |
| Fig. 3 (a) XRD patterns and (b) Raman spectra of MoP HSs, MoP/NiFeP-1 HSs and MoP/NiFeP-2 HSs. (c) High-resolution TEM image of MoP/NiFeP-1 HSs (inset: interface between MoP and NiFeP) and (d) EDS images of MoP/NiFeP-1 HSs. | |
Fig. 3c presents distinct lattice fringes of MoP/NiFeP-1 HSs, which can be assigned to the (001), (100) and (101) planes of MoP, and also the (111) and (201) planes of NiFeP.42,67 Moreover, these nanocrystals of MoP and NiFeP closely stacked, creating abundant interfaces of the heterogeneous materials. Moreover, the homogeneous distribution of Mo, Ni, Fe, P and C is revealed in Fig. 3d.
In addition, to inspect the changes of valence states and bonding structures induced by the introduction of NiFeP into MoP HSs, X-ray photoelectron spectroscopy (XPS) was applied. The high-resolution Mo 3d spectra (Fig. S6a, ESI†) can be deconvoluted and assigned to three states of Mo (Mo-P, Mo4+ and Mo6+). Due to inevitable surface oxidization in the ambient environment, Mo4+ and Mo6+ species appear.39,68,69 Since MoP is regarded as the main active site toward the HER, more effort is devoted to exploring the change of Mo-P species here. The peaks centered at 228.6 and 232.0 eV are assigned to Mo-P (3d5/2) and Mo-P (3d3/2) in the primitive MoP HSs, respectively. Along with the introduction of NiFeP, the binding energy peaks of Mo-P species visibly redshift to lower positions. Peaks of Mo-P (3d5/2) and Mo-P (3d3/2) of MoP/NiFeP-2 HSs red-shift 0.1 and 0.5 eV compared to the pristine MoP HSs, respectively. Similarly, the peaks of P–M (P-Metal) and P–C deconvoluted in the P 2p spectra also reveal lower binding energies after addition of NiFeP into MoP HSs (Fig. S6b, ESI†). The decreasing binding energies for Mo and P species suggest electron transfer from NiFeP to MoP, which can be ascribed to the interface coupling effect between MoP and NiFeP. To further prove this assumption, 2p3/2 spectra of Ni and Fe are also shown in Fig. S6c and d (ESI†). Compared to the NiFeP NPs whose Ni-P is centered at 853.3 eV, the binding energies of Ni-P for MoP/NiFeP-1 HSs and MoP/NiFeP-2 HSs increase 0.2 and 0.1 eV, respectively. Similar results can be found after deconvoluting the Fe 2p3/2 spectra. The characteristic peaks of Fe-P positively shift to 707.3 and 707.2 eV for MoP/NiFeP-1 HSs and MoP/NiFeP-2 HSs respectively, while the Fe-P for NiFeP NPs are centered at 706.8 eV.46,61,70 All these results indicate the existence of electronic interaction between MoP and NiFeP. Generally, MoP and NiFeP are considered as highly active catalysts for the HER and OER, respectively. Because enriched electrons around MoP can enhance the adsorption of H, while electron-deficiency favors the combination with oxygen-containing intermediates for NiFeP, the heterointerfaces between MoP and NiFeP can utilize the synergistic effect to accelerate the water dissociation, facilitating the reaction kinetics of the HER and OER.71–73
To investigate the HER activity in basic media, the obtained electrocatalysts were loaded onto a glassy carbon electrode in a three-electrode device. As displayed in Fig. 4a, the primitive MoP HSs need an overpotential of 146 mV to achieve 10 mA cm−2. After the introduction of NiFeP, the overpotentials of MoP/NiFeP-1 HSs and MoP/NiFeP-2 HSs are reduced to 73 and 118 mV, respectively. The HER performance of the MoP/NiFeP-1 HSs exceeds those of the recently reported HER electrocatalysts in an alkaline environment (Table S2, ESI†), though not comparable to commercial Pt/C. In contrast, the NiFeP NPs do not display any HER activity. The Tafel slope of MoP/NiFeP-1 HSs is fitted to be 31 mV dec−1 compared to the primitive MoP HSs (66 mV dec−1) and NiFeP NPs (163 mV dec−1) (Fig. 4b), implying much faster reaction kinetics of the Volmer–Tafel mechanism (H2O + e− = H* + OH− and H* + H* = H2, * denotes as the active sites).17,74,75 The improved HER activity and accelerated reaction kinetics manifest the benefit of constructing the MoP/NiFeP heterostructures. However, excess introduction of inactive NiFeP would cover the active sites of MoP and slow down the reaction rate. The Tafel slope of MoP/NiFeP-2 HSs is 45 mV dec−1, with Heyrovsky reaction (H2O + e− + H* = H2 + OH−) as the rate-limiting step. The turnover frequency (TOF) is applied to understand the intrinsic activity of the electrocatalysts.76–78 At the overpotentials of 80 and 100 mV, the TOFs of the MoP/NiFeP-1 HSs are 0.128 and 0.42 s−1, significantly higher than those of other electrocatalysts, indicating the enhanced activity of MoP after moderate introduction by NiFeP (Fig. 4c). By employing the water-gas displacing method to collect the generated H2 on the cathode, the faradaic efficiency is calculated to be almost 100% (Fig. 4d), indicating high utilization ratio of electrons and negligible by-products during the electrocatalytic HER.
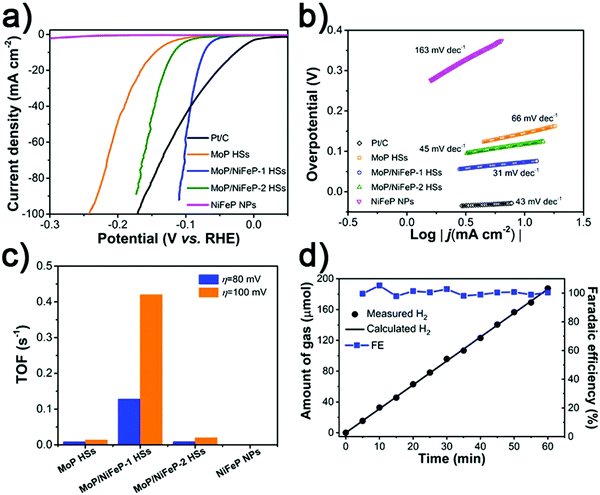 |
| Fig. 4 (a) HER polarization curves, (b) Tafel plots, (c) TOFs and (d) faradaic efficiency of H2 generation over the MoP/NiFeP-1 HS electrode at the current of −10 mA obtained in 1 M KOH. | |
Results obtained from electrochemical impedance measurements displayed that a small amount of NiFeP can facilitate the charge transfer by the interface coupling effect (Fig. S7, ESI†), resulting in the decreasing resistance of charge transfer (Rct). To eliminate the morphological contribution to catalytic activity, polarization curves were normalized by electrochemical active surface area (ECSA).27,79 As shown in Fig. S8 and S9 (ESI†), MoP/NiFeP-1 HSs still considerably outperformed other electrocatalysts, indicating the performance improvement induced by the formation of MoP/NiFeP heterostructures.
The OER activity of the as-prepared electrocatalysts was also studied. As shown in Fig. 5a, MoP HSs, MoP/NiFeP-2 HSs, NiFeP NPs and commercial Ir/C require relatively high overpotentials of 325, 271, 285 and 406 mV respectively, to achieve 10 mA cm−2, while the MoP/NiFeP-1 HSs only need 256 mV, which also exceeds the recently reported typical OER catalysts (Table S3, ESI†). The Tafel slopes of MoP/NiFeP-1 HSs and MoP/NiFeP-2 HSs are 44 and 57 mV dec−1, respectively, substantially lower than those of the primitive MoP HSs and NiFeP NPs (Fig. 5b), indicating an effectively facilitated kinetics of the OER. Similar results can also be obtained by comparing the TOFs of the electrocatalysts (Fig. 5c). Since MoP and NiFeP favor different intermediates in the reaction, the interfaces of the MoP/NiFeP heterostructures can facilitate the formation of O* (HO* + OH− = O* + H2O + e−) and HOO* (O* + OH− = HOO* + e−) during the OER by a synergistic effect.41,80 However, the OER performance cannot be further enhanced by introducing more NiFeP into the MoP HSs owing to the concealed interfaces. The faradaic efficiency of the MoP/NiFeP-1 HSs is determined to be about 98% for 60 min electrolysis (Fig. 5d), also revealing high OER productivity.
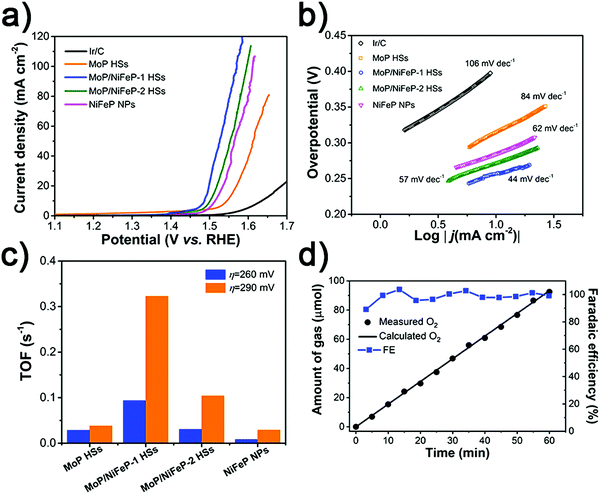 |
| Fig. 5 (a) OER polarization curves, (b) Tafel plots, (c) TOFs and (d) faradaic efficiency of O2 generation over the MoP/NiFeP-1 HS electrode at the current of 10 mA obtained in 1 M KOH. | |
Meanwhile, EIS measurements displayed the varied Rct corresponding to the order of OER activity (Fig. S10, ESI†). This indicates the rapid electron transfer for the OER on the MoP/NiFeP heterostructures. The polarization curves normalized by ECSA indicated that MoP/NiFeP-1 has the most superior catalytic properties (Fig. S11 and S12, ESI†). However, more NiFeP gradually weakens the interface effect.
Encouraged by the highly electrocatalytic performance toward both the HER and OER in 1 M KOH, we further utilized MoP/NiFeP-1 HSs for the overall water electrolytic test in a two-electrode device. As shown in Fig. 6a, the MoP/NiFeP HSs can afford 10 mA cm−2 at 1.51 V, which is very comparable to the commercial Ir/C-Pt/C couple (1.48 V) and surpass those of the recently reported typical bifunctional electrocatalysts (Table S4, ESI†). Profiting from the massive surface area and short diffusion paths for electrons/ions endowed by the hierarchical hollow structure, the MoP/NiFeP HSs even significantly outperform the Ir/C-Pt/C couple in the high current density region. Moreover, the MoP/NiFeP HSs exhibit excellent stability with only insignificant deactivation observed over 40 h tests (Fig. 6b). Besides, after prolonged operation, the polarization curve shows no obvious shift (Fig. 6c).
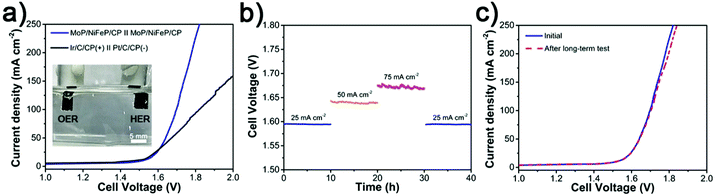 |
| Fig. 6 (a) Polarization curves obtained in the two-electrode system, (b) multistep chronopotentiometry response of MoP/NiFeP HS and (c) polarization curves of MoP/NiFeP-1 HS electrodes recorded before and after a long-term test. | |
Various measurements were further utilized to inspect the morphological and compositional change of the MoP/NiFeP-1 HSs after a durability test. On the cathode, the TEM image showed that the hierarchical hollow structure of the MoP/NiFeP HSs was well maintained (Fig. S13a and b, ESI†). Based on the analysis of the HRTEM and XRD results, no significant changes were revealed in the material structure or composition (Fig. S13c and d, ESI†). The surface species of MoP and NiFeP could still be distinguished in the XPS spectra after a prolonged test (Fig. S14, ESI†), indicating that MoP/NiFeP can stably exist in basic media during the HER.81,82 On the other hand, the nanostructure of the MoP/NiFeP HSs also remained intact on the anode (Fig. S15a and b, ESI†). However, by HRTEM analysis (Fig. S15c, ESI†), only the lattice fringes of MoP were easily observed. The area of the amorphous region increased after the test, possibly due to the in situ formed oxidized species from NiFeP under high oxidation potential. Meanwhile, the XRD pattern showed no significant difference (Fig. S15d, ESI†). According to the XPS analyses (Fig. S16, ESI†), the characteristic peaks of Mo-P disappeared in the Mo 3d region, while the peak intensity of the Mo4+ and Mo6+ species increased, implying the surface oxidation of MoP during the OER.83 Similarly, the peaks of P–M, Ni–P and Fe–P also disappeared after the test indicating the transformation of NiFeP into the corresponding oxidized species.84–87 The surface oxidized species serve as not only the active sites for the oxidation of oxygen containing species to O2, but also the protective layer to prevent the materials from corrosion.88–91 Such excellent water splitting efficiency and stability render the MoP/NiFeP HSs as a feasible alternative to noble-metal materials in practical use.
Conclusions
In summary, we have demonstrated an effective one-step strategy of synthesizing hierarchical MoP/NiFeP hybrid hollow spheres as bifunctional electrocatalysts with excellent efficiency for the HER and OER in alkaline electrolyte. Owing to the strong interfacial coupling effects between MoP and NiFeP, the greatly conductive support of graphitic carbon and the hierarchical hollow structure, the obtained MoP/NiFeP HSs exhibit superior electrocatalytic performances to the recently reported non-noble metal bifunctional electrocatalysts, with 73 and 256 mV overpotentials for the HER and OER, and a low cell voltage of 1.51 V to achieve 10 mA cm−2 for overall water electrolysis. This facile coprecipitation strategy can further be extended to design and produce other hybrid materials with hierarchical structures for diverse potential energy-related applications.
Conflicts of interest
There are no conflicts to declare.
Acknowledgements
Financial support provided for this research by the National Key Research and Development Program of China (2017YFA0204600) and the National Natural Science Foundation of China (51721002 and 52033003) is appreciated.
References
- M. K. Debe, Electrocatalyst approaches and challenges for automotive fuel cells, Nature, 2012, 486, 43–51 CrossRef CAS.
- J. A. Turner, Sustainable Hydrogen Production, Science, 2004, 305, 972–974 CrossRef CAS.
- X. Zou and Y. Zhang, Noble metal-free hydrogen evolution catalysts for water splitting, Chem. Soc. Rev., 2015, 44, 5148–5180 RSC.
- Y. Liu, Y. Zhao, Y. Sun, J. Cao, H. Wang, X. Wang, H. Huang, M. Shao, Y. Liu and Z. Kang, A 4e−–2e− cascaded pathway for highly efficient production of H2 and H2O2 from water photo-splitting at normal pressure, Appl. Catal., B, 2020, 270, 118875 CrossRef CAS.
- N. Yao, P. Li, Z. Zhou, Y. Zhao, G. Cheng, S. Chen and W. Luo, Synergistically Tuning Water and Hydrogen Binding Abilities Over Co4N by Cr Doping for Exceptional Alkaline Hydrogen Evolution Electrocatalysis, Adv. Energy Mater., 2019, 9, 1902449 CrossRef CAS.
- T. Liu, P. Li, N. Yao, T. Kong, G. Cheng, S. Chen and W. Luo, Self-Sacrificial Template-Directed Vapor-Phase Growth of MOF Assemblies and Surface Vulcanization for Efficient Water Splitting, Adv. Mater., 2019, 31, 1806672 CrossRef.
- J. Zhang, X. Sun, Y. Liu, L. Hou and C. Yuan, Design and construction of bi-metal MOF-derived yolk–shell Ni2P/ZnP2 hollow microspheres for efficient electrocatalytic oxygen evolution, Mater. Chem. Front., 2020, 4, 1366–1374 RSC.
- Y. Zhao, N. Yang, H. Yao, D. Liu, L. Song, J. Zhu, S. Li, L. Gu, K. Lin and D. Wang, Stereodefined Codoping of sp-N and S Atoms in Few-Layer Graphdiyne for Oxygen Evolution Reaction, J. Am. Chem. Soc., 2019, 141, 7240–7244 CrossRef CAS.
- P. Du and R. Eisenberg, Catalysts made of earth-abundant elements (Co, Ni, Fe) for water splitting: recent progress and future challenges, Energy Environ. Sci., 2012, 5, 6012 RSC.
- Z. W. Seh, J. Kibsgaard, C. F. Dickens, I. Chorkendorff, J. K. Norskov and T. F. Jaramillo, Combining theory and experiment in electrocatalysis: insights into materials design, Science, 2017, 355, eaad4998 CrossRef.
- Y. Shi and B. Zhang, Recent advances in transition metal phosphide nanomaterials: synthesis and applications in hydrogen evolution reaction, Chem. Soc. Rev., 2016, 45, 1529–1541 RSC.
- Y. Li, Z. Mao, Q. Wang, D. Li, R. Wang, B. He, Y. Gong and H. Wang, Hollow nanosheet array of phosphorus-anion-decorated cobalt disulfide as an efficient electrocatalyst for overall water splitting, Chem. Eng. J., 2020, 390, 124556 CrossRef CAS.
- M. Zhu, Y. Zhou, Y. Sun, C. Zhu, L. Hu, J. Gao, H. Huang, Y. Liu and Z. Kang, Cobalt phosphide/carbon dots composite as an efficient electrocatalyst for oxygen evolution reaction, Dalton Trans., 2018, 47, 5459–5464 RSC.
- F. Yang, Y. Zhao, Y. Du, Y. Chen, G. Cheng, S. Chen and W. Luo, A Monodisperse Rh2P-Based Electrocatalyst for Highly Efficient and pH-Universal Hydrogen Evolution Reaction, Adv. Energy Mater., 2018, 8, 1703489 CrossRef.
- J. Zhang, T. Wang, P. Liu, Z. Liao, S. Liu, X. Zhuang, M. Chen, E. Zschech and X. Feng, Efficient hydrogen production on MoNi4 electrocatalysts with fast water dissociation kinetics, Nat. Commun., 2017, 8, 15437 CrossRef CAS.
- F. Hu, S. Zhu, S. Chen, Y. Li, L. Ma, T. Wu, Y. Zhang, C. Wang, C. Liu, X. Yang, L. Song, X. Yang and Y. Xiong, Amorphous Metallic NiFeP: A Conductive Bulk Material Achieving High Activity for Oxygen Evolution Reaction in Both Alkaline and Acidic Media, Adv. Mater., 2017, 29, 1606570 CrossRef.
- D. Wang, Q. Li, C. Han, Z. Xing and X. Yang, When NiO@Ni Meets WS2 Nanosheet Array: A Highly Efficient and Ultrastable Electrocatalyst for Overall Water Splitting, ACS Cent. Sci., 2018, 4, 112–119 CrossRef CAS.
- Z. Wang, Q. Lei, Z. Wang, H. Yuan, L. Cao, N. Qin, Z. Lu, J. Xiao and J. Liu, In-situ synthesis of free-standing FeNi-oxyhydroxide nanosheets as a highly efficient electrocatalyst for water oxidation, Chem. Eng. J., 2020, 395, 125180 CrossRef CAS.
- H. Zhu, R. Jiang, X. Chen, Y. Chen and L. Wang, 3D nickel-cobalt diselenide nanonetwork for highly efficient oxygen evolution, Sci. Bull., 2017, 62, 1373–1379 CrossRef CAS.
- J. Guo, J. Sun, Y. Sun, Q. Liu and X. Zhang, Electrodepositing Pd on NiFe layered double hydroxide for improved water electrolysis, Mater. Chem. Front., 2019, 3, 842–850 RSC.
- J. Zhang, Y. Liu, C. Sun, P. Xi, S. Peng, D. Gao and D. Xue, Accelerated Hydrogen Evolution Reaction in CoS2 by Transition-Metal Doping, ACS Energy Lett., 2018, 3, 779–786 CrossRef CAS.
- C. Wan, Y. N. Regmi and B. M. Leonard, Multiple phases of molybdenum carbide as electrocatalysts for the hydrogen evolution reaction, Angew. Chem., Int. Ed., 2014, 53, 6407–6410 CrossRef CAS.
- Y. Shen, Y. Zhou, D. Wang, X. Wu, J. Li and J. Xi, Nickel–Copper Alloy Encapsulated in Graphitic Carbon Shells as Electrocatalysts for Hydrogen Evolution Reaction, Adv. Energy Mater., 2018, 8, 1701759 CrossRef.
- H. Lin, N. Liu, Z. Shi, Y. Guo, Y. Tang and Q. Gao, Cobalt-Doping in Molybdenum–Carbide Nanowires Toward Efficient Electrocatalytic Hydrogen Evolution, Adv. Funct. Mater., 2016, 26, 5590–5598 CrossRef CAS.
- R. Zhang, X. Wang, S. Yu, T. Wen, X. Zhu, F. Yang, X. Sun, X. Wang and W. Hu, Ternary NiCo2Px Nanowires as pH-Universal Electrocatalysts for Highly Efficient Hydrogen Evolution Reaction, Adv. Mater., 2017, 29, 1605502 CrossRef.
- Y. Zhao, X. Zhang, X. Jia, G. I. N. Waterhouse, R. Shi, X. Zhang, F. Zhan, Y. Tao, L.-Z. Wu, C.-H. Tung, D. O'Hare and T. Zhang, Sub-3 nm Ultrafine Monolayer Layered Double Hydroxide Nanosheets for Electrochemical Water Oxidation, Adv. Energy Mater., 2018, 8, 1703585 CrossRef.
- Y. Y. Chen, Y. Zhang, W. J. Jiang, X. Zhang, Z. Dai, L. J. Wan and J. S. Hu, Pomegranate-like N,P-Doped Mo2C@C Nanospheres as Highly Active Electrocatalysts for Alkaline Hydrogen Evolution, ACS Nano, 2016, 10, 8851–8860 CrossRef CAS.
- Y. Yang, K. Zhang, H. Lin, X. Li, H. C. Chan, L. Yang and Q. Gao, MoS2–Ni3S2 Heteronanorods as Efficient and Stable Bifunctional Electrocatalysts for Overall Water Splitting, ACS Catal., 2017, 7, 2357–2366 CrossRef CAS.
- G.-F. Chen, T. Y. Ma, Z.-Q. Liu, N. Li, Y.-Z. Su, K. Davey and S.-Z. Qiao, Efficient and Stable Bifunctional Electrocatalysts Ni/NixMy (M = P, S) for Overall Water Splitting, Adv. Funct. Mater., 2016, 26, 3314–3323 CrossRef CAS.
- K. Guo, Y. Wang, S. Yang, J. Huang, Z. Zou, H. Pan, P. S. Shinde, S. Pan, J. Huang and C. Xu, Bonding interface boosts the intrinsic activity and durability of NiSe@Fe2O3 heterogeneous electrocatalyst for water oxidation, Sci. Bull., 2020 DOI:10.1016/j.scib.2020.06.003.
- X. Xiao, D. Huang, Y. Fu, M. Wen, X. Jiang, X. Lv, M. Li, L. Gao, S. Liu, M. Wang, C. Zhao and Y. Shen, Engineering NiS/Ni2P Heterostructures for Efficient Electrocatalytic Water Splitting, ACS Appl. Mater. Interfaces, 2018, 10, 4689–4696 CrossRef CAS.
- A. Jiang, W. Zhang, Z. Wang, N. P. Nidamanuri, Q. Li, X. C. Zeng and M. Dong, Direct synthesis of bifunctional nanorods from a Co–adenine–MoO3 hybrid for overall water splitting, Mater. Chem. Front., 2020, 4, 546–554 RSC.
- J. Miao, F.-X. Xiao, H. B. Yang, S. Y. Khoo, J. Chen, Z. Fan, Y.-Y. Hsu, H. M. Chen, H. Zhang and B. Liu, Hierarchical Ni–Mo–S nanosheets on carbon fiber cloth: a flexible electrode for efficient hydrogen generation in neutral electrolyte, Sci. Adv., 2015, 1, e1500259 CrossRef.
- X. Yan, K. Li, L. Lyu, F. Song, J. He, D. Niu, L. Liu, X. Hu and X. Chen, From Water Oxidation to Reduction: Transformation from NixCo3−xO4 Nanowires to NiCo/NiCoOx Heterostructures, ACS Appl. Mater. Interfaces, 2016, 8, 3208–3214 CrossRef CAS.
- M.-R. Gao, J.-X. Liang, Y.-R. Zheng, Y.-F. Xu, J. Jiang, Q. Gao, J. Li and S.-H. Yu, An efficient molybdenum disulfide/cobalt diselenide hybrid catalyst for electrochemical hydrogen generation, Nat. Commun., 2015, 6, 5982 CrossRef CAS.
- H. Lin, Z. Shi, S. He, X. Yu, S. Wang, Q. Gao and Y. Tang, Heteronanowires of MoC-Mo2C as efficient electrocatalysts for hydrogen evolution reaction, Chem. Sci., 2016, 7, 3399–3405 RSC.
- J. Yan, L. Chen and X. Liang, Co9S8 nanowires@NiCo LDH nanosheets arrays on nickel foams towards efficient overall water splitting, Sci. Bull., 2019, 64, 158–165 CrossRef CAS.
- G. Li, Y. Sun, J. Rao, J. Wu, A. Kumar, Q. N. Xu, C. Fu, E. Liu, G. R. Blake, P. Werner, B. Shao, K. Liu, S. Parkin, X. Liu, M. Fahlman, S.-C. Liou, G. Auffermann, J. Zhang, C. Felser and X. Feng, Carbon-Tailored Semimetal MoP as an Efficient Hydrogen Evolution Electrocatalyst in Both Alkaline and Acid Media, Adv. Energy Mater., 2018, 8, 1801258 CrossRef.
- B. Liu, H. Li, B. Cao, J. Jiang, R. Gao and J. Zhang, Few Layered N, P Dual-Doped Carbon-Encapsulated Ultrafine MoP Nanocrystal/MoP Cluster Hybrids on Carbon Cloth: An Ultrahigh Active and Durable 3D Self-Supported Integrated Electrode for Hydrogen Evolution Reaction in a Wide pH Range, Adv. Funct. Mater., 2018, 28, 1801527 CrossRef.
- C.-F. Du, K. N. Dinh, Q. Liang, Y. Zheng, Y. Luo, J. Zhang and Q. Yan, Self-Assemble and In Situ Formation of Ni1−xFexPS3 Nanomosaic-Decorated MXene Hybrids for Overall Water Splitting, Adv. Energy Mater., 2018, 8, 1801127 CrossRef.
- Y.-J. Tang, H.-J. Zhu, L.-Z. Dong, A. M. Zhang, S.-L. Li, J. Liu and Y.-Q. Lan, Solid-phase hot-pressing of POMs-ZIFs precursor and derived phosphide for overall water splitting, Appl. Catal., B, 2019, 245, 528–535 CrossRef CAS.
- C. Du, M. Shang, J. Mao and W. Song, Hierarchical MoP/Ni2P heterostructures on nickel foam for efficient water splitting, J. Mater. Chem. A, 2017, 5, 15940–15949 RSC.
- Y. Liu, S. Jiang, S. Li, L. Zhou, Z. Li, J. Li and M. Shao, Interface engineering of (Ni, Fe)S2@MoS2 heterostructures for synergetic electrochemical water splitting, Appl. Catal., B, 2019, 247, 107–114 CrossRef CAS.
- Q. Hu, X. Liu, B. Zhu, L. Fan, X. Chai, Q. Zhang, J. Liu, C. He and Z. Lin, Crafting MoC2-doped bimetallic alloy nanoparticles encapsulated within N-doped graphene as roust bifunctional electrocatalysts for overall water splitting, Nano Energy, 2018, 50, 212–219 CrossRef CAS.
- M. Miao, R. Hou, R. Qi, Y. Yan, L. Q. Gong, K. Qi, H. Liu and B. Y. Xia, Surface evolution and reconstruction of oxygen-abundant FePi/NiFeP synergy in NiFe phosphides for efficient water oxidation, J. Mater. Chem. A, 2019, 7, 18925–18931 RSC.
- J. Yu, G. Cheng and W. Luo, Hierarchical NiFeP microflowers directly grown on Ni foam for efficient electrocatalytic oxygen evolution, J. Mater. Chem. A, 2017, 5, 11229–11235 RSC.
- L. Lin, M. Chen and L. Wu, Facile synthesis of nickel–copper hollow spheres as efficient bifunctional electrocatalysts for overall water splitting, Mater. Chem. Front., 2020, 4, 996–1005 RSC.
- M. Fang, G. Dong, R. Wei and J. C. Ho, Hierarchical Nanostructures: Design for Sustainable Water Splitting, Adv. Energy Mater., 2017, 7, 1700559 CrossRef.
- I. K. Mishra, H. Zhou, J. Sun, F. Qin, K. Dahal, J. Bao, S. Chen and Z. Ren, Hierarchical CoP/Ni5P4/CoP microsheet arrays as a robust pH-universal electrocatalyst for efficient hydrogen generation, Energy Environ. Sci., 2018, 11, 2246–2252 RSC.
- J. Wang, D. Chao, J. Liu, L. Li, L. Lai, J. Lin and Z. Shen, Ni3S2@MoS2 core/shell nanorod arrays on Ni foam for high-performance electrochemical energy storage, Nano Energy, 2014, 7, 151–160 CrossRef CAS.
- T. An, Y. Wang, J. Tang, W. Wei, X. Cui, A. M. Alenizi, L. Zhang and G. Zheng, Interlaced NiS2–MoS2 nanoflake-nanowires as efficient hydrogen evolution electrocatalysts in basic solutions, J. Mater. Chem. A, 2016, 4, 13439–13443 RSC.
- Q. Yue, J. Sun, S. Chen, Y. Zhou, H. Li, Y. Chen, R. Zhang, G. Wei and Y. Kang, Hierarchical Mesoporous MXene–NiCoP Electrocatalyst for Water-Splitting, ACS Appl. Mater. Interfaces, 2020, 12, 18570–18577 CrossRef CAS.
- D. Mao, J. Wan, J. Wang and D. Wang, Sequential Templating Approach: A Groundbreaking Strategy to Create Hollow Multishelled Structures, Adv. Mater., 2019, 31, 1802874 CrossRef.
- W. Hao, R. Wu, R. Zhang, Y. Ha, Z. Chen, L. Wang, Y. Yang, X. Ma, D. Sun, F. Fang and Y. Guo, Electroless Plating of Highly Efficient Bifunctional Boride-Based Electrodes toward Practical Overall Water Splitting, Adv. Energy Mater., 2018, 8, 1801372 CrossRef.
- C. Wang, L. Sun, F. Zhang, X. Wang, Q. Sun, Y. Cheng and L. Wang, Formation of Mo-Polydopamine Hollow Spheres and Their Conversions to MoO2/C and Mo2C/C for Efficient Electrochemical Energy Storage and Catalyst, Small, 2017, 13, 1701246 CrossRef.
- H. Zhang, L. Yu, T. Chen, W. Zhou and X. W. D. Lou, Surface Modulation of Hierarchical MoS2 Nanosheets by Ni Single Atoms for Enhanced Electrocatalytic Hydrogen Evolution, Adv. Funct. Mater., 2018, 28, 1807086 CrossRef.
- Y. Gong, Z. Yang, Y. Lin, J. Wang, H. Pan and Z. Xu, Hierarchical heterostructure NiCo2O4@CoMoO4/NF as an efficient bifunctional electrocatalyst for overall water splitting, J. Mater. Chem. A, 2018, 6, 16950–16958 RSC.
- J.-Q. Chi, W.-K. Gao, L.-M. Zhang, B. Dong, K.-L. Yan, J.-H. Lin, B. Liu, Y.-M. Chai and C.-G. Liu, Induced Phosphorization-Derived Well-Dispersed Molybdenum Phosphide Nanoparticles Encapsulated in Hollow N-Doped Carbon Nanospheres for Efficient Hydrogen Evolution, ACS Sustainable Chem. Eng., 2018, 6, 7676–7686 CrossRef CAS.
- J.-S. Li, X.-R. Wang, J.-Y. Li, S. Zhang, J.-Q. Sha, G.-D. Liu and B. Tang, Pomegranate-like molybdenum phosphide@phosphorus-doped carbon nanospheres coupled with carbon nanotubes for efficient hydrogen evolution reaction, Carbon, 2018, 139, 234–240 CrossRef CAS.
- Y. Ge, P. Dong, S. R. Craig, P. M. Ajayan, M. Ye and J. Shen, Transforming Nickel Hydroxide into 3D Prussian Blue Analogue Array to Obtain Ni2P/Fe2P for Efficient Hydrogen Evolution Reaction, Adv. Energy Mater., 2018, 8, 1800484 CrossRef.
- R.-Q. Li, B.-L. Wang, T. Gao, R. Zhang, C. Xu, X. Jiang, J. Zeng, Y. Bando, P. Hu, Y. Li and X.-B. Wang, Monolithic electrode integrated of ultrathin NiFeP on 3D strutted graphene for bifunctionally efficient overall water splitting, Nano Energy, 2019, 58, 870–876 CrossRef CAS.
- P. He, X.-Y. Yu and X. W. Lou, Carbon-Incorporated Nickel–Cobalt Mixed Metal Phosphide Nanoboxes with Enhanced Electrocatalytic Activity for Oxygen Evolution, Angew. Chem., Int. Ed., 2017, 129, 3955–3958 CrossRef.
- Y.-Y. Ma, C.-X. Wu, X.-J. Feng, H.-Q. Tan, L.-K. Yan, Y. Liu, Z.-H. Kang, E.-B. Wang and Y.-G. Li, Highly efficient hydrogen evolution from seawater by a low-cost and stable CoMoP@C electrocatalyst superior to Pt/C, Energy Environ. Sci., 2017, 10, 788–798 RSC.
- S. Jeoung, B. Seo, J. M. Hwang, S. H. Joo and H. R. Moon, Direct conversion of coordination compounds into Ni2P nanoparticles entrapped in 3D mesoporous graphene for an efficient hydrogen evolution reaction, Mater. Chem. Front., 2017, 1, 973–978 RSC.
- G. Fu, Z. Cui, Y. Chen, Y. Li, Y. Tang and J. B. Goodenough, Ni3Fe-N Doped Carbon Sheets as a Bifunctional Electrocatalyst for Air Cathodes, Adv. Energy Mater., 2017, 7, 1601172 CrossRef.
- L. Yang and L. Zhang, N-enriched porous carbon encapsulated bimetallic phosphides with hierarchical structure derived from controlled electrodepositing multilayer ZIFs for electrochemical overall water splitting, Appl. Catal., B, 2019, 259, 118053 CrossRef CAS.
- J.-S. Li, S. Zhang, J.-Q. Sha, J.-Y. Li, X.-R. Wang and H. Wang, A Polyoxometalate-Based Metal–Organic Framework-Derived FeP/MoP Hybrid Encapsulated in N/P Dual-Doped Carbon as Efficient Electrocatalyst for Hydrogen Evolution, Cryst. Growth Des., 2018, 18, 4265–4269 CrossRef CAS.
- Y. Jiang, Y. Shen, J. Dong, S. Tan, Q. Wei, F. Xiong, Q. Li, X. Liao, Z. Liu, Q. An and L. Mai, Surface Pseudocapacitive Mechanism of Molybdenum Phosphide for High-Energy and High-Power Sodium-Ion Capacitors, Adv. Energy Mater., 2019, 9, 1900967 CrossRef.
- Y. Huang, X. Song, J. Deng, C. Zha, W. Huang, Y. Wu and Y. Li, Ultra-dispersed molybdenum phosphide and phosphosulfide nanoparticles on hierarchical
carbonaceous scaffolds for hydrogen evolution electrocatalysis, Appl. Catal., B, 2019, 245, 656–661 CrossRef CAS.
- Q. Zhu, B. Qiu, H. Duan, Y. Gong, Z. Qin, B. Shen, M. Xing and J. Zhang, Electron directed migration cooperated with thermodynamic regulation over bimetallic NiFeP/g-C3N4 for enhanced photocatalytic hydrogen evolution, Appl. Catal., B, 2019, 259, 118078 CrossRef CAS.
- B. Zhang, J. Liu, J. Wang, Y. Ruan, X. Ji, K. Xu, C. Chen, H. Wan, L. Miao and J. Jiang, Interface engineering: The Ni(OH)2/MoS2 heterostructure for highly efficient alkaline hydrogen evolution, Nano Energy, 2017, 37, 74–80 CrossRef CAS.
- A. Muthurasu, V. Maruthapandian and H. Y. Kim, Metal–organic framework derived Co3O4/MoS2 heterostructure for efficient bifunctional electrocatalysts for oxygen evolution reaction and hydrogen evolution reaction, Appl. Catal., B, 2019, 248, 202–210 CrossRef CAS.
- X. Zhou, Y. Liu, H. Ju, B. Pan, J. Zhu, T. Ding, C. Wang and Q. Yang, Design and Epitaxial Growth of MoSe2–NiSe Vertical Heteronanostructures with Electronic Modulation for Enhanced Hydrogen Evolution Reaction, Chem. Mater., 2016, 28, 1838–1846 CrossRef CAS.
- Y. N. Regmi, A. Roy, L. A. King, D. A. Cullen, H. M. Meyer, G. A. Goenaga, T. A. Zawodzinski, N. Labbé and S. C. Chmely, Lattice Matched Carbide–Phosphide Composites with Superior Electrocatalytic Activity and Stability, Chem. Mater., 2017, 29, 9369–9377 CrossRef CAS.
- J. Hu, C. Zhang, L. Jiang, H. Lin, Y. An, D. Zhou, M. K. H. Leung and S. Yang, Nanohybridization of MoS2 with Layered Double Hydroxides Efficiently Synergizes the Hydrogen Evolution in Alkaline Media, Joule, 2017, 1, 383–393 CrossRef CAS.
- J. Nai, Y. Lu, L. Yu, X. Wang and X. W. D. Lou, Formation of Ni-Fe Mixed Diselenide Nanocages as a Superior Oxygen Evolution Electrocatalyst, Adv. Mater., 2017, 29, 1703870 CrossRef.
- L. Yan, L. Cao, P. Dai, X. Gu, D. Liu, L. Li, Y. Wang and X. Zhao, Metal–Organic Frameworks Derived Nanotube of Nickel–Cobalt Bimetal Phosphides as Highly Efficient Electrocatalysts for Overall Water Splitting, Adv. Funct. Mater., 2017, 27, 1703455 CrossRef.
- F. Lai, J. Feng, X. Ye, W. Zong, G. He, Y.-E. Miao, X. Han, X. Y. Ling, I. P. Parkin, B. Pan, Y. Sun and T. Liu, Energy level engineering in transition-metal doped spinel-structured nanosheets for efficient overall water splitting, J. Mater. Chem. A, 2019, 7, 827–833 RSC.
- S. Anantharaj, S. R. Ede, K. Karthick, S. Sam Sankar, K. Sangeetha, P. E. Karthik and S. Kundu, Precision and correctness in the evaluation of electrocatalytic water splitting: revisiting activity parameters with a critical assessment, Energy Environ. Sci., 2018, 11, 744–771 RSC.
- Z. Kou, T. Wang, Q. Gu, M. Xiong, L. Zheng, X. Li, Z. Pan, H. Chen, F. Verpoort, A. K. Cheetham, S. Mu and J. Wang, Rational Design of Holey 2D Nonlayered Transition Metal Carbide/Nitride Heterostructure Nanosheets for Highly Efficient Water Oxidation, Adv. Energy Mater., 2019, 9, 1803768 CrossRef.
- H. Sun, Y. Min, W. Yang, Y. Lian, L. Lin, K. Feng, Z. Deng, M. Chen, J. Zhong, L. Xu and Y. Peng, Morphological and Electronic Tuning of Ni2P through Iron Doping toward Highly Efficient Water Splitting, ACS Catal., 2019, 9, 8882–8892 CrossRef CAS.
- C. Huang, C. Pi, X. Zhang, K. Ding, P. Qin, J. Fu, X. Peng, B. Gao, P. K. Chu and K. Huo,
In Situ Synthesis of MoP Nanoflakes Intercalated N-Doped Graphene Nanobelts from MoO3-Amine Hybrid for High-Efficient Hydrogen Evolution Reaction, Small, 2018, 14, 1800667 CrossRef.
- Y. Jiang, Y. Lu, J. Lin, X. Wang and Z. Shen, A Hierarchical MoP Nanoflake Array Supported on Ni Foam: A Bifunctional Electrocatalyst for Overall Water Splitting, Small Methods, 2018, 2, 1700369 CrossRef.
- H. Liang, A. N. Gandi, C. Xia, M. N. Hedhili, D. H. Anjum, U. Schwingenschlögl and H. N. Alshareef, Amorphous NiFe-OH/NiFeP Electrocatalyst Fabricated at Low Temperature for Water Oxidation Applications, ACS Energy Lett., 2017, 2, 1035–1042 CrossRef CAS.
- P. Li, X. Duan, Y. Kuang, Y. Li, G. Zhang, W. Liu and X. Sun, Tuning Electronic Structure of NiFe Layered Double Hydroxides with Vanadium Doping toward High Efficient Electrocatalytic Water Oxidation, Adv. Energy Mater., 2018, 8, 1703341 CrossRef.
- Y. Feng, S. Wang, H. Wang, Y. Zhong and Y. Hu, An efficient and stable Ni–Fe selenides/nitrogen-doped carbon nanotubes in situ-derived electrocatalyst for oxygen evolution reaction, J. Mater. Sci., 2020, 55, 13927–13937 CrossRef CAS.
- E. Hu, J. Ning, D. Zhao, C. Xu, Y. Lin, Y. Zhong, Z. Zhang, Y. Wang and Y. Hu, A Room-Temperature Postsynthetic Ligand Exchange Strategy to Construct Mesoporous Fe-Doped CoP Hollow Triangle Plate Arrays for Efficient Electrocatalytic Water Splitting, Small, 2018, 14, 1704233 CrossRef.
- M. K. Bates, Q. Jia, H. Doan, W. Liang and S. Mukerjee, Charge-Transfer Effects in Ni–Fe and Ni–Fe–Co Mixed-Metal Oxides for the Alkaline Oxygen Evolution Reaction, ACS Catal., 2016, 6, 155–161 CrossRef CAS.
- T. Wang, G. Nam, Y. Jin, X. Wang, P. Ren, M. G. Kim, J. Liang, X. Wen, H. Jang, J. Han, Y. Huang, Q. Li and J. Cho, NiFe (Oxy) Hydroxides Derived from NiFe Disulfides as an Efficient Oxygen Evolution Catalyst for Rechargeable Zn–Air Batteries: The Effect of Surface S Residues, Adv. Mater., 2018, 30, 1800757 CrossRef.
- L. Yan, Y. Sun, E. Hu, J. Ning, Y. Zhong, Z. Zhang and Y. Hu, Facile in-situ growth of Ni2P/Fe2P nanohybrids on Ni foam for highly efficient urea electrolysis, J. Colloid Interface Sci., 2019, 541, 279–286 CrossRef CAS.
- L. Yan, H. Wang, J. Shen, J. Ning, Y. Zhong and Y. Hu, Formation of mesoporous Co/CoS/Metal-N-C@S, N-codoped hairy carbon polyhedrons as an efficient trifunctional electrocatalyst for Zn-air batteries and water splitting, Chem. Eng. J., 2021, 403, 126385 CrossRef CAS.
Footnote |
† Electronic supplementary information (ESI) available: Structural and compositional characterization, electrochemical test, and performance comparison of various electrocatalysts. See DOI: 10.1039/d0qm00635a |
|
This journal is © the Partner Organisations 2021 |
Click here to see how this site uses Cookies. View our privacy policy here.